, Adriaan Campo1 and Annemie Van der Linden1
(1)
Bio-Imaging Lab, Department of Biomedical Sciences, University of Antwerp, Wilrijk, Belgium
Abstract
Dementia is a clinical diagnosis; however, none of the clinical scales guarantee high sensitivity or specificity. Therefore, neuroimaging is often crucial for proper assessment. The most typical neurological symptoms of dementia are often discerned using computed X-ray tomography (CT), magnetic resonance imaging (MRI), magnetic resonance spectroscopy (MRS), single-photon emission computerized tomography (SPECT), and positron-emission tomography (PET) techniques. In general, these different imaging modalities provide different information and can be considered as being complementary rather than competitive. Nowadays, structural neuroimaging is a routine component of the diagnostic evaluation of dementia. Neuroimaging offers promise as a surrogate marker for clinical trials, and new technologies have been developed to provide more molecular and physiological biomarkers such as markers for amyloid plaques, one of the neuropathological hallmarks of AD. The combined use of imaging technology and experimental in vivo animal models for human diseases provides a unique platform to study pathological mechanisms in longitudinal studies, develop accurate and early translational diagnostic tools, and evaluate therapeutic strategies. In this chapter, an overview of how brain imaging can be used to detect both early and late stages of dementia in small animal models is explained. The early stage offers a unique therapeutic window and is diagnostically most challenging.
Key words
Small animalbrainearly diagnosisnoninvasive1 Introduction to In Vivo Small Animal Neuroimaging
The technology of neuroimaging and the development of a variety of small animal models for neurodegenerative diseases and dementia are two fields that have made rapid progress over the last years. The combined use of imaging technology and experimental in vivo animal models for human diseases provides a unique platform to study pathological mechanisms in longitudinal studies, develop accurate and early translational diagnostic tools, and evaluate therapeutic strategies. Technical advances in imaging modalities have allowed the evaluation of functional, neurochemical, anatomical, and even molecular changes in brains of small animals.
Inherent to neurodegeneration and subsequent dementia is neuronal loss, which can be detected with neuroimaging tools at terminal stages. However, monitoring of altered biological processes that might precede this stage, including changes in neurotransmitter turnover, cerebral blood flow (CBF), brain activity, and connectivity, is possible through noninvasive imaging. Molecular imaging is a rather new discipline that focuses particularly on early diagnostics combining molecular biology and in vivo imaging tools. It enables the visualization of cellular function and the follow up of molecular processes in living organisms. It differs from traditional imaging by probing disease-specific biomarkers to help imaging various targets or pathways, in particular, at the basis of disease rather than imaging the end-stage effects of these molecular alterations.
Clinically established brain imaging methods include X-ray computerized tomography (CT), MRI, and nuclear imaging, i.e., PET and SPECT. Microversions of these imaging systems are available for use in small animals. In addition to this, optical imaging methods such as fluorescence and bioluminescence – based on a light generating principle – are two other imaging methods restricted to experimental animal use.
Success in animal models, as supported by positive imaging results, does not guarantee success in clinical trials, as was demonstrated by investigation of treatment for Parkinson’s disease (PD) (1). Relying solely on imaging technology is probably not appropriate enough, but combination with other research for ratification is necessary. Until now, imaging technology has advanced our understanding of animal models of dementia and plays a critical role in the development of valid animal models at a basic science level and in preclinical evaluation of potential therapies for human disorders. Valid animal models must satisfy the criteria of having good face, construct, and predictive validity, i.e., animals have to show behavior that resembles the clinical condition, to have similar etiology as the human disease, and treatments that have beneficial effects in humans should induce also positive changes in the animal model (2). One has to keep in mind that preclinical research is only as good as the animal model being employed.
This introduction gives an overview of the different imaging tools applied within the context of small animal neurological research. Traditionally, neuroimaging techniques have been categorized as either structural or functional, according to the primary information they provide, nowadays, initial structural techniques can be altered to observe functional information (e.g., functional MRI (fMRI)) as well. Similarly, traditional functional methods, such as PET, can also be used to view structures (e.g., amyloid plaques) (3–5). In general, different imaging modalities provide different information and can be considered as being complementary rather than competitive. Therefore, the combination of techniques such as coregistration of structural information with functional and molecular information is of high interest and receives a lot of attention.
1.1 MRI and MRS
MRI uses radio waves in the presence of a powerful magnetic field to align the nuclear magnetization of (usually) hydrogen atoms in water in the body. Atomic nuclei absorb and reemit electromagnetic waves at a resonant frequency, which falls in the radiofrequency range. Originally, MRI was used for producing anatomical images (6) but developed to a technique with a wealth of information contained in the signal regarding the physicochemical state of tissues, flow, diffusion, motion, and more recently, molecular targets. MR has much greater soft tissue contrast than CT, making it especially useful in neurological imaging.
For most medical brain examinations in general, both T1– and T2-weighted images are acquired for observation of white-matter and gray-matter brain structures and dementia-related morphological changes, such as changes in brain volume and size of the ventricle and specific substructures. Other specific MRI parameters correlate with the diffusion of water molecules (i.e., Brownian motion) in biological tissues (7) and are quite valuable in studying neuropathology-related changes in intra/extracellular water balance and subcellular structural changes (8). The recent development of diffusion tensor imaging (DTI) enables diffusion measurement simultaneously in different directions from which tissue anisotropy can be calculated per voxel (9). From these images, brain maps of fiber directions can be reconstructed to study brain connectivity, a feature affected in several neuropathologies (10). fMRI is capable of mapping functional regions of the cortex in real time during specific task activation. This is achieved using magnetic susceptibility image contrast, which detects the local increase in CBF due to activation. Deoxyhemoglobin is paramagnetic and interacts with the magnetic field, whereas oxyhemoglobin is not. As a consequence, a higher influx of oxygenated blood during activation increases local T2 and T2* values. Such signal intensity change is termed blood oxygenation level-dependent (BOLD) contrast (11). MRI is currently still a new modality in the fields of in vivo molecular and cellular imaging compared with the established methods such as PET and optical imaging. This delayed development is probably inherent to the low sensitivity of MRI. However, MRI is gaining headway, since its main contribution to molecular imaging is its high spatial resolution compared to other methods. MRI can achieve a microscopic resolution of 100 µm in all dimensions on a routine basis in living animals, and 10 µm is feasible in fixed specimens (12). This almost represents conventional histology, while allowing the object to be viewed interactively in any plane. Merely prolonging scan time to increase spatial resolution is not a viable option in fragile, anesthetized transgenic animals. However, with the development of higher field strength magnets and the use of small cryogenically operated coils (13), spatial resolution is still improving because of the increase in signal-to-noise ratio. Some in vivo MRI studies of mouse brain reported so far cover a wide range of voxel sizes and field strengths, e.g., 98 × 156 × 1,500 μm3 at 11.7 T (14), 140 × 140 × 1,200 μm3 at 4.7 T (15), 156 × 156 × 1,000 μm3 at 14 T (16), and 78 × 156 × 343 μm3 at 7 T (17).
Advances in MRI have expanded its use in neuroimaging by applying exogenous contrast agents. The range of applications of these compounds is determined largely by their biodistribution and pharmacokinetics. Contrast agents are typically injected intravenously and, depending on their chemical structure, may remain in the vasculature (iron oxide particles), enter the interstitial space (Gadolinium chelates), or be taken up by cells (manganese). Macromolecular contrast agents are intravascular agents and are well suited to quantitative imaging of perfusion and vascular volume in brain activity studies (18). Freely diffusible contrast agents such as Gadolinium chelates are used in tissue permeability studies such as blood–brain barrier (BBB) leakage, as none of the contrast agents can pass the BBB. For molecular imaging applications, “targeted” and “smart” contrast agents were developed (19,20). Targeted agents incorporate ligands, such as antibodies that bind to specific molecular markers in the tissue, whereas smart agents are activated by the presence of specific ions or enzymes. These agents allow in vivo visualization of gene expression, an emerging field within molecular imaging (21). Moreover, MRI is also one of the completely safe imaging tools since this technique does not make use of ionizing or nuclear compound injections.
MRS is a magnetic resonance technique that can measure brain biochemistry. This technique together with other MRI applications provides metabolic information such as anatomical, physiological, functional, or molecular imaging. There is increasing evidence that impairment of energy metabolism, mitochondrial dysfunction, and altered glutamatergic neurotransmission are critical factors in the development and progression of neurodegenerative diseases. Therefore, in vivo MRS provides a useful tool in the evaluation of the underlying processes, as many of the observable metabolites prove to be relevant in the assessment of neurochemical changes associated with neurodegeneration and dementia.
In localized 1H-MRS, the target is the proton with a natural abundance of almost 100%, ultimately allowing the quantification of more than 18 1H containing metabolites in vivo (22). Localized MRS techniques allow assessment of metabolites resorting from a well-defined volume, e.g., an affected brain region, and can return single- or multivolume information with microliter resolution. Multivolume methods are known as chemical shift imaging (CSI) or spectroscopical imaging (SI), which give a voxelwise metabolite distribution, i.e., a metabolite image (23,24) (Fig. 5).
Metabolites relevant for neurodegeneration can be used as fingerprints for the diseased brain. The 1H-MRS spectrum of the normal brain is dominated by the large N-acetyl aspartate (NAA) resonance at 2.01 ppm. The exact role of NAA remains unknown, but it has been used extensively as a marker for neuronal density, thereby giving a measure for neuron survival and death (25). Myo-inositol (mI) is a cyclic sugar alcohol present in high concentrations. The precise function of mI is not known, but it has been dedicated as being an osmolyte, a glial marker, or a breakdown product of myelin. Typical changes of mI over time are seen in AD, cognitive impairment, and brain injury (26,27). Taurine (Tau) is another putative osmolyte of the brain. It has also been implicated as a modulator of neurotransmitter action. Tau is present in all cell types of the CNS, being a prominent metabolite in rodents rather than in humans (28). Glutamate (Glu), the major excitatory neurotransmitter and main precursor of γ-aminobutyric acid (GABA) and gluthation (GSH) (29), can be detected with MRS together with glutamine (Gln). Lactate (Lac) is normally present at low concentrations in the brain and is elevated in cases such as stroke, ischemia, or tumors (30–32). One of the most apparent resonances in a 1H-MRS spectrum of the brain is the combined resonance of both creatine (Cr) and phosphocreatine (PCr) at 3.03 ppm, also referred to as total creatine (tCr). The tCr signal is relatively constant with only negligible changes reported with age or disease. This is the reason why it is often used as an internal reference for quantification (33). Choline (Cho)-containing compounds form a prominent resonance in 1H-MRS spectra of the brain at 3.2 ppm. Since there are contributions of glycerophosphocholine (GPC), Cho, and phosphocholine (PCho), it is often referred to as total choline (tCho). Together with mI, tCho is often considered as a glial marker, and thus a marker for gliosis, since concentrations of these compounds are typically much higher in glia than in neurons (34,35).
1.2 Computerized Tomography (CT)
The techniques of CT and MRI are commonly used as instrumental aids for structural imaging in the diagnosis of dementia (21,36,37). However, new imaging tools are emerging and might significantly change the way CT and MRI are used nowadays in clinical settings. CT is a medical imaging method employing tomography, which means that digital geometry processing is used to generate a 3D image of the inside of an object from a large series of 2D X-ray images taken around a single axis of rotation. CT visualizes differences in the physical densities of biological tissues through X-ray absorption. The main issue within radiology today is how to reduce the radiation dose during CT examinations without compromising image quality.
For animal research, µCT systems are available. They are fivefold cheaper than µMRI systems and are straightforward to site in a laboratory. 3D µCT has proven to be a powerful technique for imaging and analysis of bone structure and density in small animals (38). Although initial µCT imaging was performed ex vivo, recent advances allow for similar imaging protocols to be performed in vivo (39). Unlike ex vivo µCT, which provides very fine spatial resolution (typically less than 10 μm), the spatial resolution of in vivo µCT studies suffers from physiologic (e.g., cardiac and respiratory) motion. High-resolution in vivo µCT has become important for examining morphology in small animal models for tumor disease (40,41).
Experimental CT imaging in animal models for dementia is very limited since soft tissue imaging using CT requires the use of X-ray absorbing contrast agents due to the lack of native CT contrast in the brain. Especially in the brain, distinction between gray and white matter (in quantitative terms, gray matter 20–35 HU (Hounsfield units), white matter 30–40 HU) needs addition of a radio-opaque component (iodine anion). There is, however, a CT study in a mouse model for dementia wherein postmortem material amyloid plaques could be discerned (42).
Upon application of intravenous injection of a CT contrast agent, investigation of cerebral vascular system impairments is made possible (43). The occurrence of cerebral vascular abnormalities is not only linked to vascular dementia but is also an issue often discussed concerning all types of dementia.
1.3 Nuclear Imaging Techniques (PET and SPECT)
Together with MRI, PET and SPECT are the most commonly used imaging techniques in animal models. PET uses biologically active molecules in µmolar or ηmolar concentrations that have been labeled with short-lived positron-emitting isotopes. Opposed to PET, the imaging agent used in SPECT emits gamma rays.
Both PET and SPECT can be used to measure regional CBF, which is physiologically coupled to brain metabolism making its use relevant in dementia studies. The radiotracer is assumed to accumulate in areas of the brain, in proportion to the rate of delivery of nutrients to that volume of brain tissue.
PET also provides measures of regional cerebral glucose metabolism (rCMRglc) by using a positron-emitting glucose analog, fluorodeoxyglucose (FDG). FDG-PET has become a standard technique in oncology and is gaining headway in dementia research in animal models.
Limitations to the widespread use of PET arise from the high costs of cyclotrons needed to produce the short-lived radionuclides for PET scanning and the need for specially adapted on-site chemical synthesis apparatus to produce the radiopharmaceuticals. SPECT scans using 99mTc-HMPAO (hexamethylpropylene amine oxime) competes with FDG-PET scanning of the brain to provide very similar information about local brain damage from many processes. SPECT is more widely available because the radioisotope generation technology is longer lasting and far less expensive. The same is true for the gamma scanning equipment.
A combined or multimodality scanner, such as SPECT/CT or PET/CT, can acquire coregistered structure and function in a single study. The data are complementary, allowing CT to accurately localize functional abnormalities and SPECT or PET to highlight areas of abnormal metabolism. Although technically more challenging, the simultaneous acquisition of MR and PET has also been demonstrated, initially for preclinical imaging of small animals, but more recently for the human brain (44).
PET is an extremely sensitive technique allowing for the identification of subtle changes in brain neurochemistry by labeling the molecules with high specificity. Selective tracers for many neurotransmitter receptors and other cellular proteins or tracers that act as an enzyme substrate, which allow the detection of a specific neuronal function, are available. Novel disease-specific probes are being developed very rapidly. New perspectives are opened by tracers for imaging amyloid plaques, tracers for measuring local acetylcholinesterase (AChE) activity and the binding capacity of nicotinic and serotonergic receptors, which address neurotransmitter deficits in dementia.
Nuclear medicine is a part of molecular imaging because it produces images that reflect biological processes taking place at the cellular and subcellular level. Nuclear imaging techniques, however, suffer from poor spatial resolution, with the highest resolution scanners today giving at best 4-mm resolution and for animal systems up to 1.5 mm. Therefore, PET and SPECT scans are increasingly read alongside CT or MRI scans, taking advantage of the coregistration of both anatomic and metabolic information to estimate brain regions of altered radioligand uptake. For the same reason, and very recently, small animal SPECT/MRI dual hybrid device combining pinhole SPECT imager adjacent to a small low-field MR imager has been developed (45).
1.4 Optical Imaging (BLI and FLI)
BLI and FLI enable visualization of genetic expression and physiological processes at the molecular level in living tissues. Reporter genes that encode either fluorescent or bioluminescent proteins have been used to create internal biological light in the study of animal models for human biology and are commonly used in tumor research (46). Longitudinal recordings of living animal models with these modalities have shown to provide clues for the development of clinical applications. Because of the presence of the skull, optical methods for brain applications are more restricted, but nevertheless abundant.
Near-infrared fluorescent (NIRF) probes are typically small fluorescent molecule dyes designed to absorb and emit light in the near-infrared (650–950 nm), where tissue scattering is lowest. The simple synthesis, the low cost, and the long life of NIRF probes present an attractive alternative for MRI and PET techniques in the field of molecular imaging.
BLI does not rely on an external light as for FLI, instead uses luminescence sourcing (e.g., luciferase) from, e.g., the firefly. Sensitive charged-coupled device (CCD) cameras are used to capture the bioluminescent data, which are superimposed on the photographic image for interpretation. Because there is no competing background signal, those techniques can detect low levels of light emission. The DNA encoding the luminescent protein is incorporated into the laboratory animal either via a viral vector or by creating a transgenic animal. Within these experimental animals, luciferine is injected and its oxidation by luciferase provides bioluminescence. Because luciferase imaging requires genetic transfection, it is very unlikely to ever find an application for this technique in human studies. Nevertheless, it can be very useful in predicting human response to therapy in the early stages of drug development.
In dementia research, progression is made in designing smart optical probes that emit a characteristic fluorescence signal only when bound to the studied target, i.e., a biomarker for the disease. The first NIRF probes for use in dementia were designed in 2001 and targeted amyloid in the brain of mouse models for AD (47). Until now, targeting amyloid is the most common application in dementia research.
The potential of BLI in dementia research finds its application in stem-cell-based therapies using bioluminescent stem cells and BLI as a superior viability marker. Stem-cell-based therapies may offer a potentially attractive and unlimited source of cells for the repair of damaged tissue in CNS disease. Experimental CNS transplantation studies have largely evaluated the in vivo survival, proliferation, and migrational capacity of neural grafts within the host tissue with histology at several different time points. Suitable noninvasive imaging technologies may serve as tools for probing neural graft behavior in the living brain. While other imaging approaches, such as MRI or PET, might have been chosen for this purpose, the limitations associated with these modalities outweigh their benefits. Particularly in MRI, which can monitor stem-cell populations that are iron-labeled, the in vivo distinction between viable and nonviable cells is not possible. Moreover, iron uptake can be confounded by other resident cells such as macrophages.
2 Small Animal Brain Atlases Using Imaging Methods
Localization of the major findings in the brain is an essential parameter both for the interpretation in an anatomical and functional context and for comparing findings across specimens and between healthy and diseased brains. As the importance of digital atlases, spatial indexes, and management systems for distribution data is increasingly recognized worldwide, several parallel advanced atlas systems emerge, providing both overlapping and complementary resources (48–54) (BIRN; www.nbirn.net).
Tomographic neuroimaging techniques allow visualization of functionally and structurally specific signals in the mouse and rat brain. The interpretation of the image data relies on accurate determination of anatomical location. However, lack of structural background information in the surrounding tissue limits the identification of anatomical boundaries. The primary benefit of CT is its ability to view the brain within the skull with a high spatial resolution. The acquisition of CT brain images has shown to be beneficial in the reconstruction of a reference brain atlas for rodents since skull landmarks (directly detectable with CT) could be directly mapped to the existing atlas coordinate space (55,56).
Side-by-side comparison of image data with conventional atlas diagram is hampered by the 2D format of the atlases and by the lack of an analytical environment for accumulation of data and integrative analyses. Hjornevik et al. (57) presented a method for reconstructing 3D atlases from digital 2D atlas diagrams and exemplified 3D atlas-based analysis of PET and MRI data. Using the in vivo pathway tracer MnCl2, Hjornevik et al. revealed the topographical organization of the corticothalamic structures. After injection of MnCl2 in the somatosensory cortex, the size and location of the MnCl2 injections were analyzed by 3D combined visualization of the MR-based representation of the brain surface and the atlas representations of different cerebrocortical areas. The position of the manganese-enhanced signal within the thalamus was analyzed by slicing the combined image and atlas volumes in 200-µm-thick slices using a multiplatform tool (Fig. 1).
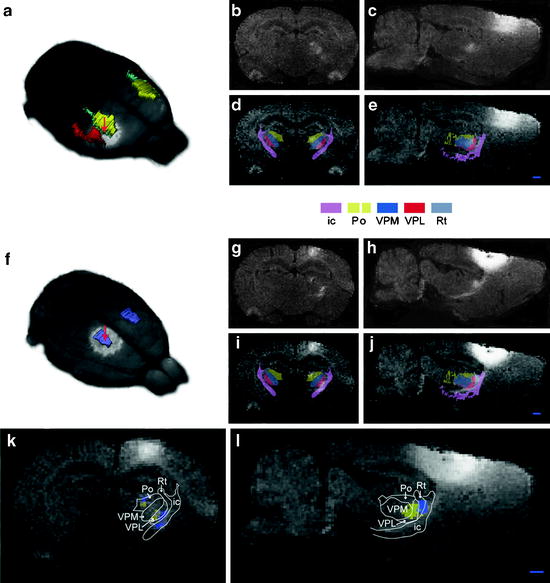
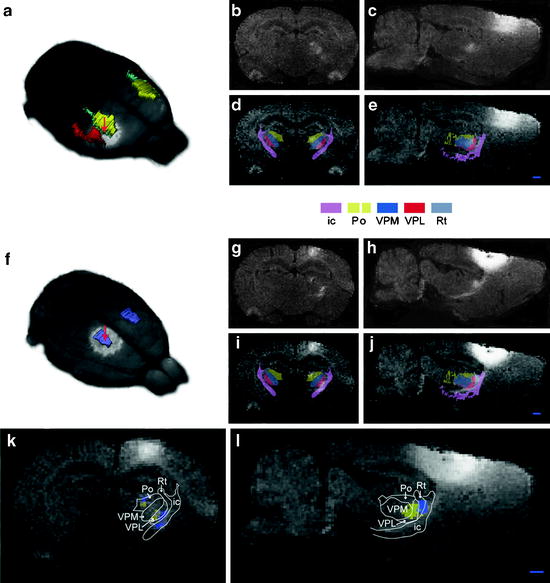
Fig. 1.
Corticothalamic topographical organization revealed by MnCl2/MRI tracing and 3D atlasing. Two cases are shown, case 1 (a–e), in which MnCl2 was injected in the dysgranular zone adjacent to the SI forelimb representation (b), and case 2 (f–j), in which MnCl2 was injected in the hind limb representation of SI. (b, c and g, h) Raw coronal and sagittal T1-weighted MRI images obtained 9 h after injection. (d, e and i, j) Corresponding images with overlay of selected reconstructed atlas structures. (k, l) Superimposed, pseudocolored images (case 1, yellow; case 2, blue) with an overlay of atlas-derived boundary lines, demonstrating a somatotopical organization of the different signals within the Po and VPM. Bar, 1 mm (Adapted from (57)) ic, internal capsule; Po, posterior thalamic nucleus; VPM, ventral posterolateral thalamic nucleus; VPL, ventral posteromedial thalamic nucleus; Rt, reticular thalamic nucleus.
Recently, an MRI-based small animal PET template was reconstructed for FDG metabolic activity and the dopamine transporter (58). The 3D MRI T2-weighted images were oriented according to the rat brain Paxinos atlas (56), which facilitated the accurate assessment and spatial localization of ligand-specific PET information. Currently, 30 mouse brain structures and 60 rat brain structures have been reconstructed. To exploit the 3D atlas models, they have developed a multiplatform atlas tool (available via The Rodent Workbench, http://rbwb.org), which allows combined visualization of experimental image data within the 3D atlas space together with 3D viewing and user-defined slicing of selected atlas structures. The presented tool facilitates assignment of location and comparative analysis of signal location in tomographic images with low structural contrast. The possibility of multimodal imaging responds to the desire of integrating information obtained by various imaging devices to unravel pathological mechanisms underlying neurological diseases. This means that the frequently used reference atlas space (59) for use in human brain imaging data has been made available in animals in many different formats to have a common reference space in the scientific community.
3 Imaging in Neurodegeneration and Dementia
We will focus on how brain imaging can be used to detect both early and late stages of dementia. The early stage offers a unique therapeutic window and is diagnostically most challenging. However, one problem that investigators still face is how to distinguish primary from secondary events.
Accumulating evidence suggests that chronic neurodegenerative disorders such as AD, PD, Huntington’s disease (HD), and amyotrophic lateral sclerosis (ALS) are caused by a combination of events that impair normal neuronal function. Clinical signs are evident prior to neuronal loss. Therefore, new efforts have focused on identifying various crucial changes that hamper normal neuronal function.
3.1 Imaging Early Events
The etiologies of neurodegenerative diseases may be diverse; however, a common pathological denominator is the formation of aberrant or misfolded proteins. There is an ongoing debate about the nature of the harmful proteins and how toxic conformations selectively can damage neuronal populations. Soluble oligomers are associated with early pathological alterations, and strikingly, oligomeric assemblies of different disease-associated proteins may share common structural features. A major step toward the understanding of mechanisms implicated in neuronal degeneration is the identification of related genes. Studies based on these disease-associated genes illuminated the two faces of protein misfolding in neurodegeneration: a gain of toxic function and a loss of physiological function, which can even occur in combination (60).
Here, we review the occurrence of specific protein accumulations in several dementia disorders and summarize how these early-stage accumulations can be detected through neuroimaging methods in animal models.
3.1.1 Probing Misfolded Proteins
HD is caused by expansion of a CAG repeat coding for polyglutamine in the N-terminus of the Huntington protein (61). In AD, extraneuronal aggregates are mainly composed of amyloid β (Aβ) peptide, whereas intraneuronal aggregates are made up of the microtubule-associated tau-protein and also amyloid in more diffuse plaques (62,63). Transgenic mice overexpressing superoxide dismutase (SOD) have cytoplasmic inclusions containing aggregates of SOD protein (64), similar to what is found in human brain samples. Prion disease can be caused by mutations in the prion gene, leading to alterations of the prion protein (PrP). In all cases, prion disease is caused by abnormally folded PrP, and aggregation can take place both extracellularly and intracellularly (65). The pathological hallmark of PD is the Lewy body, an inclusion found in the cytoplasm of neurons containing aggregates of the synuclein protein (66).
To localize and quantify the deposition of misfolded proteins with high specificity, researchers aim at probing the proteins, i.e., a molecular approach. Expectations are high for the development of techniques to detect biomarkers that will significantly improve diagnosis and therapy follow-up.
Such imaging efforts have been made mostly in mouse models for AD trying to identify extraneuronal amyloid. In contrast to research for other forms of dementia in which the cloning of novel causative genes led to the development of biochemical hypotheses, AD research has largely developed in the reverse direction. The identification of the proteins that make up the classic amyloid plaques suggested their respective genes as sites to search for pathogenic mutations in AD.
A plaque-depositing transgenic mouse model is an obvious model system for the development of PET tracers aimed at imaging amyloid. The development of amyloid-specific ligands for the application of in vivo imaging started in 1992 (67). A number of potential amyloid PET ligands have been developed with most of them in clinical context (68,69). The first PET study in mice was published in 2002 (70); unfortunately, failure to detect a significant difference in [11C)PIB retention in the brains of double mutant presenilin PS1/amyloid precursor protein (APP) mice compared with PS1 littermate controls was reported. Marked reductions in brain uptake of the radioligand in transgenic mice enlightened the difficulties in PET imaging due to high requirements of the radioligands. Zhang et al. (71) therefore suggested to lower the lipophilicity and to improve bioavailability. It was also suggested that the lower uptake could be linked to a reduced CBF relative to that in wild-type mice (5). Human PET studies attempting to label amyloid were more successful. Translation of animal results to human application is not always straightforward since in humans AD pathology develops over one or more decades, whereas mouse pathology develops over months. The long time frame of deposition in human brain allows for many posttranslational and postdeposition modifications, including oxidation, crosslinking, and N- and C-terminal truncation (3).
Novel fluorescent compounds, including styryl-fluorobenzoxazole derivatives, were proposed as new PET probes for imaging of neuritic and diffuse amyloid deposits.
These compounds showed a high binding affinity for both synthetic Aβ1–40 and Aβ1–42 aggregates. Some of these compounds also displayed distinct staining of neuritic and diffuse amyloid plaques in AD brain sections. A biodistribution study of styryl-fluorobenzoxazole derivatives in normal mice exhibited excellent brain uptakes (4.5–5.5% injected dose/g at 2 min postinjection). Furthermore, intravenous administration of BF-145, a styryl-fluorobenzoxazole derivative, demonstrated specific in vivo labeling of compact and diffuse amyloid deposits in APP23 transgenic mouse brain, in contrast to no accumulation in wild-type mouse brain. These findings suggest that BF-145 is a potential candidate as a probe for imaging early brain pathology in AD patients (72).
In MRI, specificity is low because of small intrinsic contrast between protein accumulations and the surrounding brain tissue. However, in many cases, MRI has surpassed the capabilities of PET and SPECT, offering a combination of physiological sensitivity and high spatial resolution. Typically, in human AD subjects and in most of the transgenic mouse models, plaques diameter ranges from 50 to 200 µm. This is beyond the spatial resolution of PET and SPECT, and, therefore, MR microscopy with a resolution of up to 40 µm harbors the realistic potential of imaging amyloid, at least in animal models.
Many different intrinsic MR contrast mechanisms have low specificity. Although it makes use of the inherent presence of iron in amyloid plaques in AD, it was feasible to visualize the amyloid plaques in vivo in the brains of mouse models using MRI T2 and T2*-weighted contrast (73–78). In the last decade, its repertoire has been extended with targeted contrast agents improving the sensitivity of detection. In this alternative concept, three properties to enhance the plaque-related signal are essential: (1) transport across the BBB following intravenous injection, (2) binding to plaques with molecular specificity, and (3) MRI contrast. The magnetic label carried by the amyloid peptide is constructed of either paramagnetic Gd-chelates or superparamagnetic monocrystalline iron oxide nanoparticles. Transient osmotic disruption of the BBB to allow the reporter molecules to penetrate the brain parenchyma may be necessary (79). The design of BBB-penetrating smart molecular probes is still ongoing, and MR images showed an excellent correlation with immunohistochemistry. Amyloid plaques in APP-overexpressing Tg2576 and double mutant PS/APP mice were probed in vivo by putrescine-gadolinium-amyloid-β peptide (PUT-Gd-Aβ), which, because of the presence of the putrescine moiety, is transported across the BBB following intravenous injection, thereby causing specific plaque enhancement in T1-weigthed images (80,81).
In regard of the BBB as being an obstacle, approaches that use plaque-specific ligands for PET or fluorescent dyes (82,83) are more advantageous. NIRF probes for amyloid are an exciting option for molecular imaging in AD research and may translate to clinical diagnostics. The design of smart optical probes emitting characteristic fluorescence signal only when bound to amyloid (84) results in tremendous improvements. An attractive NIRF ligand, the oxazine derivative AOI987, showed specific interaction with amyloid plaques in APP23 transgenic mice as illustrated in Fig. 2 (83).
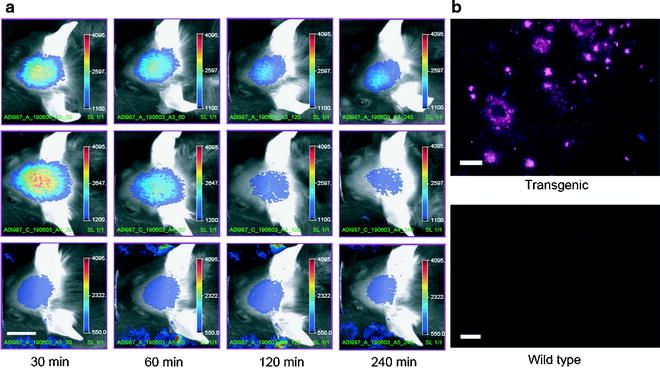
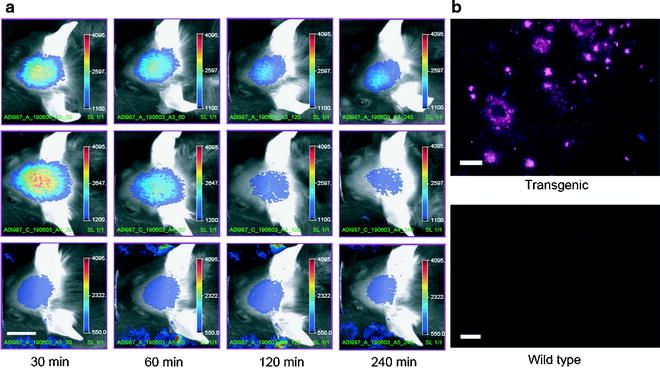
Fig. 2.
(a) Representative images of 17-month-old APP23 transgenic (top row) and wild-type (middle row) female mice injected i.v. with 0.1 mg/kg AOI98. The images were recorded 30, 60, 120, and 240 min after injection of the fluorescent dye. In the bottom row, corresponding images of a 17-month-old transgenic APP23 female mouse treated with 0.9% saline only are shown. Scale bar, 1 cm; color scale bars in arbitrary units. (b) NIRF microscopy of air-dried cryotome sections (20-µm thickness) of 16-month-old female mice that have been dosed with 0.1 mg/kg AOI987, i.v. The brains were excised and fixed 4 h after dye administration; left, APP23 transgenic; right: wild-type mouse. Scale bar, 100 µm (Adapted from (83)).
Unless CT has low soft contrast, neuritic plaques in PS/APP mice could be demonstrated making use of phase-contrast X-ray combined with 3D CT (42). High-density aggregates being neuritic plaques closely associated with neuritic dystrophy were identified ex vivo.
3.1.2 Probing Early Morphological Changes
If the detection of protein accumulations cannot be tackled directly because, for instance, the accumulations are present intraneuronally, or because of lack of specific probing ligands, research can also focus on the early loss of physiological function associated with the occurrence of protein aggregates. First, subtle functional changes in the brain can be reflected by altered brain perfusion, diffusion, or neuronal connectivity.
Proteins will deposit either extraneuronally of intraneuronally, thereby altering the diffusion properties of the brain tissue. Mueggler et al. (85) used diffusion-weighted MRI and demonstrated that extraneuronal amyloid deposits in APP23 mice are accompanied by a reduction of the diffusion coefficient. Reduced diffusivity within the interstitial space may afflict on its turn the transport of endogenous signaling molecules during synaptic or extrasynaptic transmission and constitute an early sign of cognitive impairment in AD.
With DTI, the early development of AD pathology can be studied in the white matter by quantifying axial and radial diffusivities. This was reported by Song et al. (86) showing that increased levels of amyloid deposition in the brains of PDAPP mice lead to white matter injury.
However, intraneuronal protein aggregates are intrinsically located in the gray matter, and because DTI correlates with tissue microstructure, it should find its application in the gray matter too, e.g., in cortical layers.
It is obvious that in neurons displaying aggregations, critical processes might be affected and the viability of the cell is in danger. Brain perfusion is a parameter of tissue viability and can be measured by MRI, PET, and SPECT. Detection of decreased brain perfusion might not be early enough to serve as diagnostic biomarker (see “Imaging functional changes” section). Therefore, one has to search for more subtle changes, such as brain vulnerability. It was hypothesized that pathological metabolism of APP enhances inflammation and thereby sensitizes the brain to ischemic insults (87). Unlike other mutant AD mouse models, APP751 mice do not develop plaques, and it is yet to be shown that they are highly vulnerable to a focal ischemic insult, while basal CBF was normal (as measured with MRI).
Another approach are studies conceived to elucidate the extent of deformations and eliminations that alter the morphology and architecture of the (micro)vasculature in AD mouse models (88,89). In APP23 mice, Meyer et al. clearly showed that before amyloid plaques and other hallmarks of the disease appear, APP23 mice already have altered cerebrovasculature. Analogously, Beckmann et al. (88) reported flow disturbances in large arteries of APP23 mice in the absence of plaques using MRI angiography. Absence of amyloid deposits in large arteries in the region of the circle of Willis suggests that soluble amyloid may exert deleterious effects on the vasculature, thereby supporting the idea that evolving progressively cerebral circulatory abnormalities could contribute to AD pathogenesis, and that soluble amyloid may exert deleterious effects on the vasculature. Other groups have used MRI tools to investigate the effects of dietary lipids on amyloid deposition and blood circulation in the brains of 18-month-old PS1/APP mice. Relative cerebral blood volume (CBV) and flow (CBF) were determined with proton MRS and gradient-echo contrast-enhanced MRI and support the involvement of hemodynamic changes in the development of AD (90).
3.1.3 Probing Axonal Transport Rates
Manganese has recently emerged as an easily accessible and promising positive contrast agent for MRI that covers a broad spectrum of functional and anatomical applications in small animal models. This application of manganese became known as manganese-enhanced MRI (MEMRI) (91).
The capacity of the Mn2+ ion to substitute Ca2+ in excitable tissues, such as muscle and nervous tissue, combined with the ability to obtain highly localized MRI contrast changes by using high-resolution scanning techniques, provides a new route to map cellular activity or malfunctions with high accuracy. During cellular activations of excitable tissues, Mn2+ can enter through voltage-gated Ca2+ channels and an acute increase of extracellular Mn2+ concentrations together with the activation of specific brain regions has been shown to result in an increased local MRI signal intensity. After uptake in the neuronal body, Mn2+ is transported to the synapse via fast anterograde axonal transport (92). Finally, Mn2+ can travel across the synapse and thus can enter a subsequent step of the neuronal network. These properties can be extremely useful for in vivo imaging of anatomy, physiology, and activity of neuronal networks. Moreover, because of the way Mn2+ is transported, time-related (dynamic) changes in MRI signal intensity in specific brain regions can be used to quantify axonal transport rates between the injected and the projecting area, thereby providing an accurate in vivo tool to monitor axonal transport rates.
Axonopathy is a pronounced attribute of many neurodegenerative diseases. In AD, axonal swelling and degeneration are prevalent and may contribute to the symptoms of AD senile dementia. Current limitations in identifying the contribution of axonal damage to AD include the inability to detect when this damage occurs in relation to other identifiers of AD because of the invasiveness of the existing methods. However, MEMRI after Mn2+ administration to the nostrils of the mouse was recently used to noninvasively assess in vivo axonal transport rates in wild-type mice and the Tg2576 mouse model of AD (93). These authors discerned that in vivo axonal transport rates decrease before plaque formation in the Tg2576 mouse model and illustrated the power of this in vivo methodology to assess axonopathy.
3.2 Imaging Brain Connectivity Changes
MEMRI can also be used to study brain connectivity and potential changes upon neurodegeneration. In that case, specific brain nuclei of the affected circuit are injected (targeted) with manganese, and subsequently, the signal changes in the projection areas reveal the integrity and the activity of the connections. Pelled et al. (94) performed such a study in healthy and in unilateral 6-hydroxydopamine (6-OHDA) Parkinson disease rat model to test the basal ganglia interhemispheric connectivity after injection of MnCl2 into the entopeduncular nucleus, substantia nigra (SN), and the habenula. They discovered modulations in the effective intra- and interhemispheric basal ganglia connectivity in the unilateral 6-OHDA rat model, which suggests a linkage between the dopaminergic and serotonergic systems in PD, in line with clinical symptoms. The use of manganese has been, however, limited due to concerns about toxicity, specifically acute cardiovascular depression, as it acts as a transient competitive antagonist to calcium activity, reducing contractility and systemic blood pressure. To date, it has been approved for clinical imaging only in a chelated form, i.e., manganese dipyridoxyl diphosphate (Mn-DPDP). While chelation markedly improves the acute safety profile of manganese, it does so at the expense of many of the properties that make it desirable for neuroimaging.
In humans, changes in functional connectivity in specific regions of the brain affected by dementia are investigated with DTI, resting-state, and activation fMRI studies. A multitude of studies exist in patients with AD but also in patients with HD (95,96). However, no such studies were performed on animal models, but some recent papers have exploited the technique in anesthetized control rats (97).
On the other hand, DTI studies to detect white matter changes associated with dementia were done in both patients (98) and in animal models of dementia. DTI studies in animals offer the enormous benefit of providing insight into the underlying mechanism of the observed changes in the DTI parameters being the radial and the axial component. This allows discriminating connectivity loss due to demyelinization or axonal loss (99,100). DTI studies in AD (86) and PD models (101) have been helpful in discerning the specific degenerating fibers in each disease.
3.3 Imaging Structural and Functional Changes Related with Neurogenesis at the Level of the Subventricular Zone or the Dentate Gyrus
The mammalian subventricular zone (SVZ) is the largest germinative zone of the adult brain, which contains a well-characterized stem cell niche with neuronal progenitor cells (NPCs). While most studies highlight the neurogenic potential of SVZ progenitors, recent data indicate that SVZ cells become reactivated in response to different pathological cues, such as trauma, ischemia, inflammation, demyelination, and also neurodegeneration like in PD (102) and HD (103). Similar multiple studies exist and what they have in common is that they assess cell proliferation and differentiation with the gold standard being 5-bromo-2’-deoxyuridine (BrdU) labeling, and subsequent, histology and immunohistochemistry for cell type-specific markers.
However, recently, several in vivo visualization methods were developed, which would ultimately permit to follow neuronal recruitment at the rostral migratory stream (RMS) and olfactory bulb (OB) as a read-out for neurogenesis and/or cell survival in animal models for different brain pathologies. NPCs were labeled, in vivo, in situ in adult rats with fluorescent, micron-sized iron oxide particles (MPIOs), and using MRI, the migrating neural precursors carrying MPIOs were discerned along the RMS to the OB. Immunohistochemistry and electron microscopy indicated that particles were located inside NPCs positive for glial fibrillary acid protein (GFAP+) in the SVZ, inside migrating NPCs positive for polysialylated neural cell adhesion molecule (PSA-NCAM+), in doublecortin positive NPCs within the RMS and OB, and in mature neurons in the OB staining positive for neural nucleus (Neu-N+) (104). This work demonstrates that in vivo cell labeling of progenitor cells for MRI is possible and enables serial, noninvasive visualization of endogenous progenitor/precursor cell migration. Undoubtedly, this method will be used in the near future to phenotype neurodegenerative models and to study the efficacy of endogenous progenitor cells as an attractive alternative to the existing therapeutic options for treating neurodegenerative diseases.
The group of Aigner L. et al. (105) developed a new tool based on the doublecortin promoter, driving the expression of the luciferase reporter gene (DCX-promo-luciferase) in transgenic mice to perform in vivo imaging of neurogenesis. Indeed, the DCX-promo-luciferase mice allowed optical in vivo imaging of the onset of and increase in neurogenesis in developing fetal brains, as well as imaging of neurogenesis in the intact adult mouse central nervous system. This imaging approach allows longitudinal study of neurogenesis in intact animals without the requirement of cellular prelabeling. Moreover, it guarantees that detection is specific for neuronal precursors and restricted to viable cells (106). The group of Baekelandt finally extended this to a truly quantitative method (107,108), as illustrated in mice in Fig. 3.
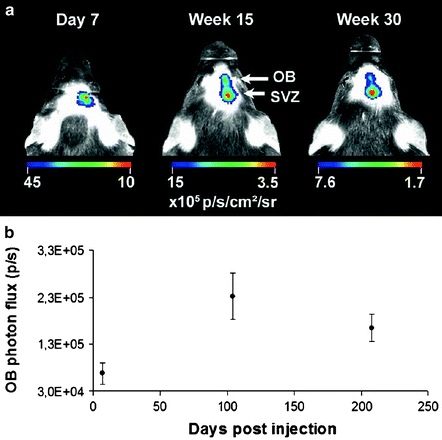
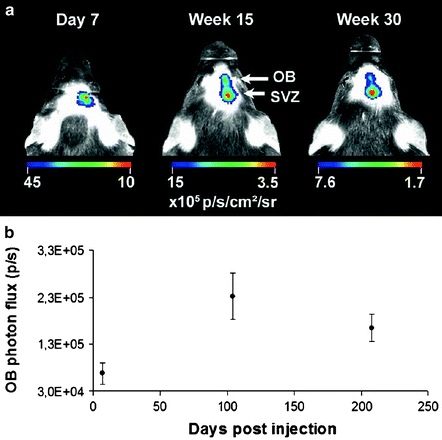
Fig. 3.
(a) At day 7 postinjection (pi), there is a detectable BLI signal only at the site of injection (SVZ). At 15 and 30 weeks pi, an additional focus is detected at the bulbus olfactorius (OB) projection site as well as the original focus at the SVZ. A representative mouse is shown. (b) The graph shows the quantification of the in vivo OB BLI signal from all the animals followed for 30 weeks (n = 14). The OB photon flux at week 15 and week 30 is significantly higher than on day 7 (p: 0.002 and p: 0.045) (Adapted from (108)).
The dentate gyrus is the second germinative zone in the mammalian brain that continuously provides the adult hippocampus with new neurons. Because the germinative layer is in close vicinity to the target region, the above-mentioned methods are not applicable. However, in dementia such as in AD, the neuroproliferative (subgranular) zone of the dentate gyrus seems to be affected, while the physiological destination of these neurons (granule cell layer), and the CA1 region of Ammon’s horn, is the principal site of hippocampal pathology. Jin et al. (109) reported that neurogenesis is enhanced in hippocampus of patients with AD, and they reproduced their findings in a transgenic mouse model, PDGF-APP(Sw, Ind) mice, which express the Swedish and Indiana APP mutations (109). Others have shown impairment in hippocampal neurogenesis in mice carrying targeted mutations in APP, PS1, or both APP and PS1 (110).
A recent study (111) used in vivo MRS to determine the load of NPCs in both human and rat dentate gyrus. Also, this might become an attractive tool for further investigating alterations in neurogenesis capacity in neurodegenerative animal models.
3.4 Imaging Functional Changes (Neuronal Performance)
3.4.1 Probing Brain Functioning with fMRI
fMRI probes secondary changes associated with neuronal activation, such as regional changes in CBV, CBF, and blood oxygenation (BOLD contrast). Such fMRI methods have emerged as a powerful tool for investigating the functional organization in the mammalian brain and are widely applied for mapping human brain function both under normal and pathological conditions. In parallel, experimental biomedical applications of fMRI techniques have been developed at a rapid pace providing relevant information on neuronal function in animal models of human disease covering a wide range of species from mice to primates (112).
It is assumed that functional imaging, including CBV, CBF, and BOLD measurements, might detect subtle alterations of neuronal function before gross anatomical abnormalities become detectable in structural images. In the APP23 transgenic AD mouse model that overexpresses human APP with the Swedish mutation, elevated brain levels and deposits of Aβ occur in an age-dependent manner and are associated with behavioral and learning deficits. Quantitative fMRI was applied to characterize brain function in this mouse model (113). Electrical stimulation of the paw led to CBV increases in the contralateral somatosensory cortex. In APP23 mice, this hemodynamic response decreased with increasing age of the animal and with increasing stimulus amplitude when compared with wild-type animals. The age-dependent dysfunction in APP23 mice may be attributed in part to a compromised cerebrovascular reactivity. These data indicate that quantitative functional brain mapping that uses standardized sensory inputs could allow for assessment of disease progression and therapy response in mice and also in patients. The same mouse model was pharmacologically challenged with the GABA-a receptor antagonist bicuculline, thereby significantly reducing the cerebral hemodynamic response. This is in part attributable to a compromised cerebrovascular reactivity, as revealed by the reduced responsiveness to vasodilatory stimulation by acetazolamide (114).
< div class='tao-gold-member'>
Only gold members can continue reading. Log In or Register a > to continue
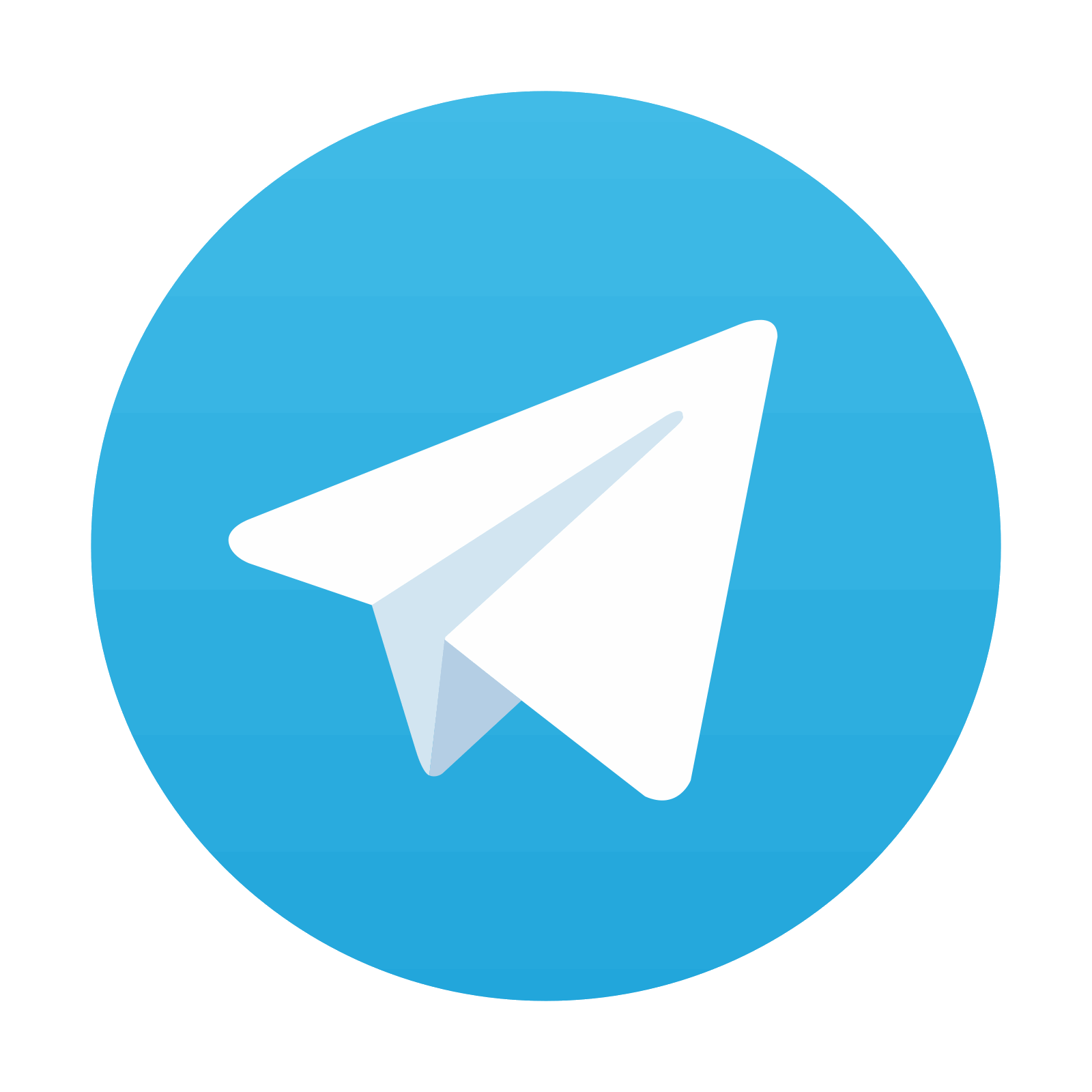
Stay updated, free articles. Join our Telegram channel
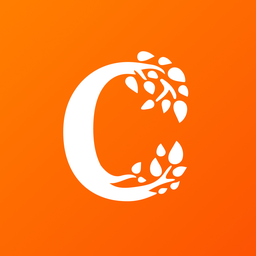
Full access? Get Clinical Tree
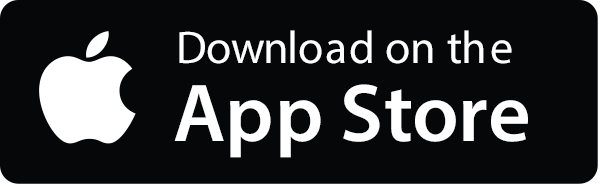
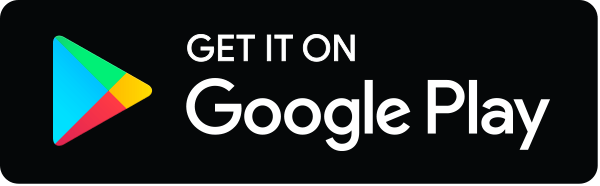