(1)
Laboratory of Neurobiology, Leuven, Belgium
Abstract
Amyotrophic lateral sclerosis (ALS) is a neurodegenerative disorder characterized by the selective death of motor neurons. Although ALS is predominantly a disease of motor system degeneration, cognitive impairments have also been reported. In order to determine the pathogenic mechanisms leading to this neurodegeneration, a large number of different animal models have helped to get insights. These animal models contain mutated genes (spontaneous or induced) or (over)express (mutant) genes, and recapitulate important aspect of this neurodegenerative disease. Although the exact mechanisms are not yet elucidated, animal models have been of great value to delineate potential pathogenic mechanisms that are involved in, and/or are important in causing selective motor neuron degeneration. Moreover, these animal models can be used as first screening assays to select potential therapeutics to treat this fatal disease.
Key words
ALSCognitive impairmentsSuperoxide dismutase 1Neurodegeneration1 Introduction
Selective death of motor neurons in the motor cortex, brain stem, and spinal cord is the cause of amyotrophic lateral sclerosis (ALS). This adult-onset, progressive neurodegenerative disorder is sporadic in 90% of cases, while 10% of patients have a familial variant of the disease. Sporadic and familial ALS are clinically indistinguishable and patients suffer from muscle weakness, atrophy, and spasticity, due to the loss of both upper and lower motor neurons. Ultimately, patients become paralyzed and denervation of respiratory muscles leads to the death of the patient, on average 3–5 years after the onset of the first symptoms. As it is very difficult to study a sporadic disease, most research focused on the familial form of the disease and on the genetic causes of familial ALS (for reviews see (1, 2)).
By far the most important cause of inherited ALS (ALS1) responsible for about 20% of familial ALS are mutations in the gene on chromosome 21q encoding superoxide dismutase 1 (SOD1) (3). The mutations are present in all five exons encoding this protein of 153 amino acids. Other rare genetic causes of the disease are mutations in the genes encoding alsin (ALS2; (4)), senataxin (ALS4; (5)), vesicle-associated membrane protein B (VAPB; ALS8; (6)), angiogenin (ALS9; (7)), and dynactin (8). More recently, several groups identified mutations in the gene encoding the TAR DNA-binding protein (TDP-43; (9–12)) as a cause of ALS. In addition, (rare) sequence variants associated with ALS have been reported in a number of other genes (e.g., neurofilaments, peripherin, vascular endothelial growth factor (VEGF)); although it is not always clear whether these are involved in the pathogenesis of ALS.
Traditionally, it is thought that cognitive functions are spared during ALS. However, evidence exists that ALS patients show a wide range of cognitive impairments (for a review see (13)). Most patients with ALS have mild cognitive impairment with subtle executive deficits, while ∼5% show frontotemporal dementia (FTD) (14, 15). Although the idea that ALS and FTD form a clinical spectrum remains controversial, there is not only clinical overlap between both diseases, but also on the pathological and genetic level. Deposits of TDP-43 are found in both ALS and FTD (16), while genetic variations in the progranulin gene could be the cause of both diseases (17–19).
A large number of animal models have been created in an attempt to unravel the pathogenic mechanisms leading to ALS (Table 1). Moreover, a number of rodent models exist that are characterized by selective motor neuron death (e.g. Pmn, Nmd, Loa, Cra1). These models are also interesting as the (induced or spontaneous) mutations responsible for the phenotype are elucidated. Unfortunately, almost no information is available on cognitive alterations in these animal models. It is not always clear whether there is indeed no effect on other brain regions or whether this aspect was not thoroughly investigated.
Table 1
Overview of animal models for motor neuron degeneration
Disease | Gene product | Inheritance | Animal | Genetic modification | Reference |
---|---|---|---|---|---|
ALS1 | Superoxide dismutase 1 | Dominant Dominant/recessive | Mouse Rat Mouse | genomic hSOD1 G37R genomic hSOD1 G85R genomic mSOD1 G86R genomic hSOD1 G93A genomic hSOD1 L126Z(stop) genomic hSOD1 L126delTT genomic hSOD1 Quad PrP; cDNA SOD1 G37R Thy-1; cDNA hSOD1 G93A genomic hSOD1 H46R genomic hSOD1 G93A genomic hSOD1 G93A genomic hSOD1 D90A | (22) (21) (23) (20) (25) (26) (30) (33) (39) (27) (27) (28) (24) |
ALS2 | Alsin | Recessive | Mouse | KO (exon 3) KO (stop codon in exon 3) KO (exon 3 and 4) KO (exon 4) | (41) (42) (43) (44) |
ALS8 | Vesicle-associated membrane protein | Dominant | Fruit fly | mutant males | (52) |
Dynactin | Dynactin | Dominant | Mouse | G59S p150Glued knock-in Thy-1; G59S p150Glued Thy-1; G59S p150Glued (X Chr) | (54) (55) (56) |
Charcot–Marie–Tooth-2E/1F | Neurofilament-L | Dominant | Mouse | NF-L L394 P | (69) |
NA | Peripherin | NA | Mouse | Overexpression | (72) |
NA | Vascular endothelial growth factor | NA | Mouse | VEGFδ/δ | (87) |
SMARD | Immunoglobulin µ-binding protein 2 | Recessive | Mouse | Nmd mouse | |
HRD/SSS | Tubulin-specific chaperone E | Recessive | Mouse | Pmn mouse | (76) |
FTDP-tau | Tau | Dominant | Mouse | 4R human tau R406W human tau P301L human tau G272V, P301S human tau V337M human tau P301S human tau K257T, P301S human tau | (78) (81) (82) (83) (84) (85) |
In this chapter, we will give an overview of the most important animal models of motor neuron degeneration and we will discuss the pathogenic information, also in relation to cognitive impairments, deduced from these genetic models.
2 ALS1 and Superoxide Dismutase 1
Shortly after the discovery of mutations in the SOD1 gene, a transgenic mouse overexpressing mutant SOD1G93A was created by insertion of multiple copies of human genomic SOD1 into the mouse genome (20). These transgenic mice showed progressive hind-limb weakness leading to paralysis and death. The moment of disease onset and the life span of these mice were related to the level of overexpression of mutant SOD1, while overexpression of non-mutated SOD1 gave no overt phenotype. Transgenic mice that (over)expressed human SOD1 containing other mutations (G37R, G85R, or D90A) or mutant (G86R) mouse SOD1 showed a similar phenotype as the mutant SOD1G93A mouse line (21–24). Moreover, transgenic mice overexpressing a C-terminal truncated SOD1 also showed motor neuron degeneration (25, 26). In addition, transgenic rats overexpressing mutant SOD1 (H46R and G93A) were created that also developed an age-dependent degeneration of motor neurons leading to paralysis and death (27, 28). The advantage of this larger rat model is that it allows studies involving complex manipulations of the spinal fluid and spinal cord (e.g., implantation of intrathecal catheters for chronic therapeutic studies, sampling of cerebrospinal fluid).
Since its creation, the mutant SOD1 mouse models have been extensively studied. These transgenic mice proved that mutant SOD1 caused selective motor neuron death by a ‘gain of function’. Moreover, these mice were used to study both the pathogenic changes that occur during the disease process, as well as to test possible drugs. Mutant SOD1 transgenic mice were also crossbred with other transgenic mice to learn about the pathogenic mechanisms involved. Using these strategies, it was shown that SOD1-mediated oxidative abnormalities were not the primary cause of mutant SOD1 toxicity. SOD1 is an enzyme that requires copper to catalyze the conversion of toxic superoxide radicals into hydrogen peroxide and oxygen. Copper plays a crucial role in the normal and/or aberrant enzymatic activity of the enzyme and copper loading of SOD1 is performed by a specific copper chaperone (CCS). Crossbreeding of transgenic mutant SOD1G93A mice with knockout mice lacking the CCS did not influence the life span of mutant SOD1G93A mice (29). Another argument that oxidative stress is not the initiating factor was presented by a transgenic mouse overexpressing mutant SOD1 in which the four essential histidines that bind copper were mutated (SOD1-Quad). Two of these mutations are known human mutations causing ALS and the SOD1-Quad mice developed age-dependent motor neuron loss despite the lack of copper binding by SOD1 (30).
SOD1 aggregates were found in ALS patients with SOD1 mutations, as well as in the different mutant SOD1 mouse models. They were present both in neurons and in surrounding glial cells (31). The formation of SOD1 aggregates was one of the first pathological signs and the abundance increased as a function of the disease process (32). Furthermore, detergent-insoluble forms of SOD1 could be detected in the brain stem and spinal cord of the different transgenic mouse models (30) and there was a clear correlation between the formation of SOD1 aggregates and disease (33). Despite these results, it is still an open question whether the formation of SOD1 aggregates is the cause of ALS. Alternatively, the formation of aggregates could be a harmless side effect of the presence of mutant SOD1, or it could even be protective by sequestering aberrant SOD1 protein.
Selective expression of mutant SOD1 in motor neurons (34) or in glial cells (35) was not sufficient to induce pathology, indicating that an interplay between different cell types is necessary to get motor neuron death. However, it was difficult to determine whether mutant SOD1 was always expressed to a sufficiently high level in these experiments. As a consequence, the most important argument for the non-cell autonomous character of motor neuron death was provided through the generation of chimeric animals. In these animals, neighboring cells that did not express mutant SOD1 delayed degeneration and significantly extended survival of mutant SOD1 expressing motor neurons (36). The opposite was found as well: normal motor neurons (not expressing mutant SOD1) surrounded by cells that did express mutant SOD1 were damaged and contained ubiquitin-positive inclusions (36). The identification of the non-neuronal cell type contributing to mutant SOD1-induced motor neuron death was done using a floxed mutant SOD1 gene that can be excised by the action of Cre recombinase. Selective reduction of the amount of mutant SOD1 in microglia and peripheral macrophages had a dramatic effect and delayed significantly the progression of the disease (37). Moreover, reduction of mutant SOD1 expression in astrocytes also affected disease progression. This selective silencing of mutant SOD1 expression in astrocytes was obtained by crossbreeding transgenic mice containing floxed mutant SOD1 with mice expressing Cre driven by the glial fibrillary acidic protein (GFAP) promoter (38). The disease progression in these double transgenic mice was significantly slowed and the effect on survival was comparable to that of the selective removal of mutant SOD1 from postnatal motor neurons (37, 38). Although these data showed that the surrounding cells clearly contribute, it was recently shown by Jaarsma et al. (39) that exclusive neuronal expression of mutant SOD1 can be sufficient to cause selective motor neuron degeneration and paralysis. The motor neurons in these transgenic mice showed clear cytosolic SOD1 aggregates as the dominant pathological feature (39).
There is no clear indication that mutant SOD1 mouse models develop age-dependent cognitive impairments. However, it was reported that overexpression of mutant SOD1 induces changes in prefrontal cortex connectivity and function before the onset of motor disturbances (40). Mutant SOD1G93A mice exhibited a paradoxical selective enhancement of reactivity to spatial changes compared to mice overexpressing wild-type SOD1. It was hypothesized that the high levels of glutamate present in the brain of presymptomatic mice could mediate site-specific and molecular and synaptic changes providing favorable conditions to spatial information processing (40).
3 ALS2 and Alsin
ALS2 is a rare autosomal, recessive form of ALS that is characterized by a juvenile onset of progressive spasticity in the limbs, facial, and pharyngeal muscles. In families of Arabic origin, mutations in the ALS2 gene on chromosome 2 were discovered (4). At present, at least 11 different mutations in the ALS2 gene have been reported leading either to ALS, to primary lateral sclerosis (PLS), or to infantile onset ascending hereditary spastic paraplegia (IAHSP) with only upper motor neurons involved (for a review (1)). All patients are homozygous for the mutant gene and all reported mutations lead to premature termination of the transcript and as a consequence to a truncated protein.
Several groups have generated an alsin knockout mouse (41–44), but these mice had no clear motor phenotype. Primary motor neuron cultures from the first line of alsin knockout mice were more susceptible to oxidative stress (41). The second transgenic mouse model showed an age-dependent, slowly progressive loss of cerebellar Purkinje cells and a disturbance of spinal motor neurons associated with astrocytosis and microglial cell activation, indicating a subclinical dysfunction of the motor system (42). The third mouse model showed mild age-independent hypoactivity and smaller cortical motor neurons. In addition, disturbances in endosomal transport were reported (43). In the fourth transgenic model, slowed movement without muscle weakness and progressive axonal degeneration in the lateral spinal cord was observed (44). In general, it can be concluded from these observations that knocking out alsin in mouse is insufficient to cause major motor deficits, while subtle behavioral changes and a clear loss in cerebellar Purkinje cells were reported.
4 ALS4 and Senataxin
ALS4 is a rare, childhood- or adolescent-onset, autosomal dominant form of ALS, characterized by slow disease progression, limb weakness, severe muscle wasting, and pyramidal signs associated with degeneration of motor neurons in the brain and spinal cord. Typical for ALS4 are the long duration of the disease and the sparing of bulbar and respiratory muscles. Different missense mutations in the gene coding for senataxin (SETX) were found in three unrelated families in Austria, Belgium, and the United States (5). Interestingly, the distal hereditary motor neuropathy (dHMN) phenotype in the Austrian and Belgian families is strikingly similar to the original ALS4 family (45), suggesting that ALS4 and dHMN with pyramidal tract signs may be one and the same disorder (46).
The discovery of missense mutations in the senataxin gene of patients suffering from ALS4 suggests that defects in RNA metabolism are involved in motor neuron death as the protein contains a C-terminal motif typical for DNA/RNA helicases. The autosomal dominant pattern of inheritance suggests that mutations in senataxin result in a gain of function. Moreover, loss-of-function mutations were identified in patients with ataxia-ocular apraxia 2 (AOA2), an autosomal recessive ataxia associated with impairment of eye movement (47).
A common denominator of a number of motor neuron diseases is indeed that proteins playing a role in RNA metabolism are involved. As a consequence, it was suggested that lower motor neurons are selectively vulnerable to defects in RNA metabolism (48). This is illustrated by the neuromuscular degeneration (Nmd) mouse. This mouse line contained a spontaneous autosomal recessive mutation giving rise to neuromuscular degeneration (49) and developed a progressive motor neuron disease, in which muscle atrophy was secondary to motor neuron loss. Homozygous Nmd mice became progressively paralyzed and rarely survived longer than 4 weeks after birth, while no behavioral or cognitive impairments were found.
A mutation that creates a cryptic splice donor site was found in intron 4 of gene encoding the immunoglobulin µ-binding protein 2 (Ighmbp2) (50) that co-localizes with the RNA-processing machinery. The consequence of this mutation is that the majority of the Ighmbp2 transcripts are aberrantly spliced, and that a truncated Ighmbp2 protein is formed. This mouse model became highly relevant after the discovery of Ighmbp2 mutations in patients suffering from spinal muscular atrophy with respiratory distress (SMARD) type 1 (51). SMARD is an autosomal recessive motor neuron disease that affects infants. Patients present with respiratory distress due to diaphragmatic paralysis and progressive muscle weakness with predominantly distal lower limb muscle involvement.
5 ALS8 and VAPB
Originally, a missense mutation in the VAPB gene was found in a Brazilian family (6). This mutation results in different clinical manifestations including late-onset spinal muscular atrophy and late-onset atypical ALS with slow progression. To date, there are eight families of which seven are of Portuguese-Brazilian and one is of African-Brazilian origin. There are no mouse models for VAPB, although the protein is highly conserved in the mouse. In Drosophila, hemizygous mutant males exhibit severe motor deficits and a compromised microtubule assembly (52).
6 Dynactin
A G59S mutation located in the microtubule-binding domain of dynactin p150Glued was described as the cause of an autosomal dominant, late-onset motor neuron disease in a large family of European descent (8). Initial in vitro microtubule binding studies indicated that mutant p150Glued exhibited a reduced binding efficiency to microtubules (8), which is consistent with a loss of function. In addition, cell culture experiments demonstrated that mutant G59S p150Glued perturbs dynactin function and causes protein aggregation (53). Recently, two mouse models were created, indicating that motor neuron loss is caused by a dominant negative mechanism (54, 55). Lai et al. (54) generated a mutant G59S p150Glued knock-in mouse. Early embryonic lethality was observed in the mutant G59S p150Glued homozygous knock-in mice, while the heterozygous mutant G59S p150Glued mice started to display a motor neuron disease-like phenotype at 10 months of age. This was accompanied by excessive accumulation of cytoskeletal and synaptic vesicle proteins at the neuromuscular junctions, loss of spinal motor neurons, increase of astrogliosis, and shortening of gait (54). Laird et al. (55) and Chevalier-Larsen et al. (56) overexpressed mutant G59S p150Glued under the control of the neuron-specific Thy-1 promoter. These mice also displayed a motor neuron disease phenotype resulting in muscle weakness, paralysis, and/or death, while no cognitive or behavioral abnormalities were reported.
Further evidence that disturbances in axonal transport can cause motor neuron loss is provided by the characterization of two other mouse lines. These mutant mouse lines, Legs at odd angles (Loa) and Cramping 1 (Cra1), arose in two independent mutagenesis experiments in the offspring of N-ethyl-N-nitrosourea (ENU)–treated mice. These mice manifest progressive motor neuron disorders and show remarkable similarities to specific features of human pathology, including Lewy body–like inclusions containing SOD1, neurofilaments, and ubiquitin. Two different point mutations in dynein were found in the Loa and Cra1 mice (57). Dynein is a motor protein complex that is involved in retrograde transport and moves in the minus-end direction along microtubules.
Similarly, disruption in postnatal motor neurons of the dynactin complex, an activator of cytoplasmic dynein that makes it more processive (58), produced a late-onset, progressive motor neuron disease in mice. This disruption was obtained by overexpression of dynamitin, the p50 subunit of dynactin, leading to neurofilamentous swellings in motor axons and inhibition of retrograde axonal transport (59).
Impairment of retrograde axonal transport could also be involved in ALS as indicated by the observation that this process is disturbed in mutant SOD1 transgenic mice (60, 61). Unexpectedly, crossbreeding of the Loa mice with mutant (G93A) SOD1 mice significantly extended the life span of the mutant SOD1G93A mice by 28% (60). Defects in retrograde transport were no longer found in motor neurons cultured from these double transgenic mice (60). These experiments indicate that axonal transport is restored by combining the dynein mutation with an SOD1 mutation, although the exact explanation for these intriguing findings is not yet clear.
7 Neurofilaments and Peripherin
Neurofilaments (NFs) are the most abundant intermediate filaments in neurons and consist of three subunits: NF-L, NF-M, and NF-H. Accumulation of NFs and concomitant slowing of slow anterograde axonal transport were reported in the mutant SOD1G93A mouse model (62). Moreover, NF accumulations were found in both familial and sporadic ALS cases (63–65). In ALS patients, mutations in the KSP phosphorylation domain of NF-H were found in a limited number of ALS patients (66). Over the years, a large number of transgenic mice with modifications related to NFs were made, in order to determine the role of these intermediate filaments in the pathology of motor neurons (for reviews (67, 68)).
Knockout mice for these different subunits alone or double transgenic mice deficient in two NF subunits did not show a clear phenotype, although in some of these mice a loss of motor axons was detected. Also overexpression of the different NF subunits did not induce motor neuron death. In some of these transgenic mice, NF accumulations in neuronal cell bodies were found, but this did not induce motor neuron death.
However, NF abnormalities can induce selective motor neuron death in vivo as indicated by a transgenic mouse expressing a mutant NF-L. In these mice, leucine at position 394 was mutated into a proline and this caused a dominant motor neuron disease (69). These transgenic mice showed massive, selective degeneration of motor neurons accompanied by accumulations of NFs, although no effect on the life span of these mice was reported. This mouse model became highly relevant after the discovery of NF-L mutations as the cause of a dominantly inherited motor neuropathy: Charcot–Marie–Tooth disease, type 2E (70, 71).
Another intermediate filament protein that could be involved in selective motor neuron death is peripherin. It is mostly expressed in the peripheral nervous system and is upregulated both in the peripheral and central nervous system after injury and by inflammatory cytokines. Transgenic mice that overexpress wild-type peripherin developed motor dysfunctions very late in their life (after 2 years). This phenotype was accompanied by the loss of motor axons and by the appearance of peripherin inclusion bodies in the motor neurons. The onset of motor dysfunction and axonal loss was dramatically accelerated by the absence of NF-L, as revealed by cross-breeding of peripherin overexpressing mice with NF-L knockout mice. These double transgenic mice also showed a dramatic loss of motor neurons (72). The exact mechanism underlying this peripherin-mediated neurodegeneration is not yet clear. Upregulation or suppression of peripherin expression had no effect on disease onset, mortality, and loss of motor neurons in mutant SOD1G37R mice, indicating that peripherin is not a contributing factor to motor neuron disease in this mouse model (73). However, this does not necessarily exclude a role for peripherin in ALS as illustrated by the discovery in ALS patients of a frameshift deletion and a mutation in the peripherin gene (74 75).
8 Tubulin-Specific Chaperone E Gene and Tau
The tubulin-specific chaperone E gene (Tbce) has an effect on microtubule stability and/or on the polymerization dynamics of microtubules. In the Pmn mouse, point mutations were identified in the Tbce gene on chromosome 13 (76). However, in humans, a deletion in Tbce is responsible for hypoparathyroidism-retardation dysmorphism syndrome (HRD or Sanjad–Sakati syndrome, SSS) or autosomal recessive Kenny–Caffey syndrome (AR-KCS). Although neurological symptoms are part of the HRD/SSS phenotype, this syndrome is clinically variable, involves multiple tissues, and is quite different from the disease observed in the Pmn mouse.
< div class='tao-gold-member'>
Only gold members can continue reading. Log In or Register a > to continue
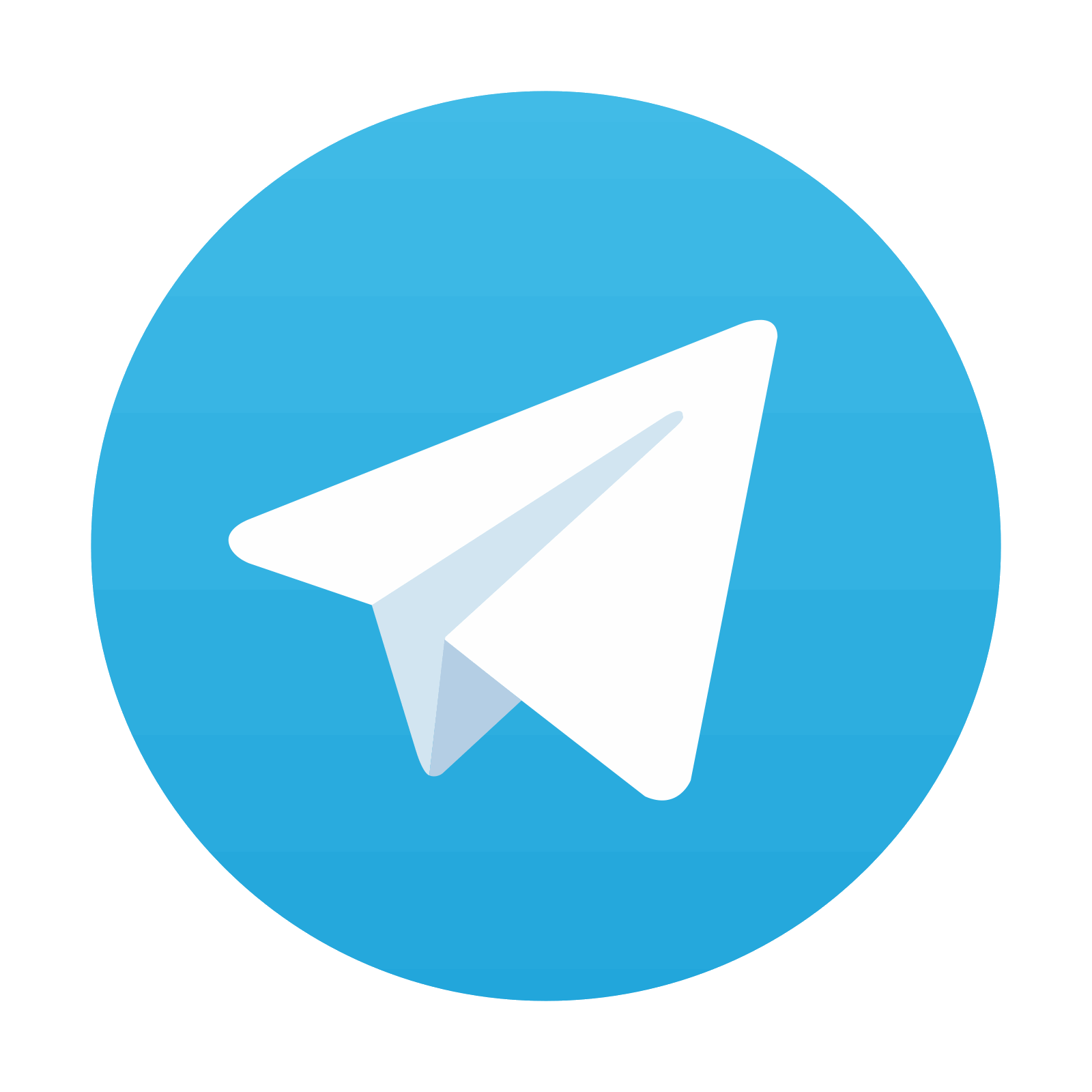
Stay updated, free articles. Join our Telegram channel
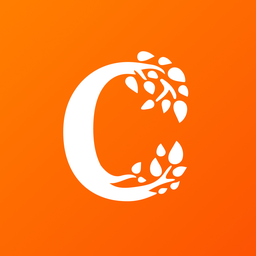
Full access? Get Clinical Tree
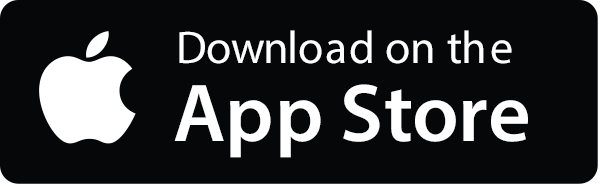
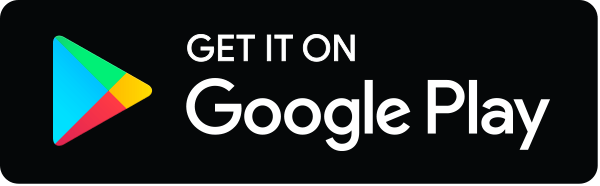