and Maria Isabel Álvarez1
(1)
Instituto Cajal, CSIC, Madrid, Spain
Abstract
No animal spontaneously suffers from Alzheimer’s disease (AD), nor can it be experimentally induced. However, there is a huge research need for models of AD. Lesion-induced vertebrate models of this disease have been, and indeed remain, extremely important in the study of AD pathogenesis and possible treatment. The cholinergic hypothesis of AD has led to the development of a number of animal models for studying the pathogeny of cortical cholinergic involution in vivo. Focal lesions in the cholinergic centers of the basal forebrain, especially of the nucleus basalis magnocellularis of rodents, as well as more general lesions of all the cholinergic neurons of the basal forebrain, have been the most used methods for obtaining these models. Surgical procedures and intraparenchymal or intracerebroventricular (i.c.v.) microinjections of toxic substances, such as quinolic, kainic, N-methyl-D-aspartic, ibotenic and quisqualic acids, the specific cholinotoxin AF64, the immunotoxin 192 IgG-saporin, and amyloid, have all been used to produce such lesions. They have also been used to produce lesion-induced cortical models of AD. Other models have been developed that involve brain implants or microinjections of amyloid as well as the administration, via different routes, of toxic agents such as aluminum, okadaic acid, or alcohol. The research carried out with these models has helped our understanding of AD, especially in the role of cerebral cholinergic innervation in cognitive disorders and their treatments. However, conflicting results are also obtained, and much controversy has developed concerning the role of the cholinergic system and the suitability of these models. It is very important to take into account a wide variety of factors (including e.g., the model protocol, the lesion-inducing agent, the type and concentration of toxin used, and even the morphohistochemical, biochemical, and cognitive methods used to evaluate the changes induced) that could condition the appreciated features of the lesion and its neuropathological and cognitive consequences. This chapter covers the theoretical and practical use of lesion-induced models and examines the main advantages and disadvantages associated with their use. The further use of classic lesion-induced models, and of new models developed in other rodent subspecies, should lead to important advances in our knowledge and treatment of AD and related disorders.
Key words
Alzheimer’s disease (AD)lesion-induced vertebrate AD modelscholinergic modelsbasal forebrainnucleus basaliscortical cholinergic innervationsexcitotoxinscholinotoxinsAD treatments1 Introduction
Alzheimer’s disease (AD) is a devastating neurodegenerative disorder. Over the last 40 years, there has been a huge research effort to determine its cause, understand its pathogenic pathways, and establish potential therapeutic targets to delay its associated neurodegeneration or indeed prevent it. Unfortunately, the successes achieved have been less than hoped for. Experimental models of AD, in particular lesion-induced vertebrate models, have played an important role in many of the advances made. This chapter is devoted to their description, the analysis of their characteristics, and a discussion on their advantages and disadvantages.
As for the majority of diseases, research on AD has been undertaken in two complementary fields. The first has involved the clinical study of patients and the morphological and biochemical analysis of postmortem brains; the second has involved the use of experimental animal models in which it is more feasible to analyze the progress of the disease and its possible modification. Clinical studies began by Alois Alzheimer himself, but experienced an important impulse in the 1960s. Gradually, clinical AD research has revealed the enormous complexity of the alterations in brain function, in the structure and function of the neurons involved, in neuronal circuits and glial cells, and in the relationship between the CNS and the rest of the body (it should not be forgotten that AD is a systemic disease). The mental and behavioral changes seen in most patients with AD dementia are clinical manifestations exclusive to humans – the higher brain functions of man have no equal among animals. The symptoms of brain dysfunction, spontaneous or induced, observed in other mammals in pathological settings supposedly similar to AD cannot be directly equated with human dementia.
The morphological, biochemical, and molecular abnormalities seen in the brains of people with AD are complex; an array of constitutive elements and functional systems involving neurons and glial cells has been implicated, and the number of related pathological abnormalities continues to grow, particularly at the molecular level and with respect to intracellular functional systems. Some factors appear to facilitate the onset of AD (although how is not always clear), such as the possession of certain alleles (e.g., apolipoprotein E4). Certain functional anomalies at critical points in cell systems seem to be closely related to the neurodegeneration that characterizes this disease (e.g., the anomalous processing of amyloid precursor protein (APP) via the amyloidogenic route, the formation of aberrant intracellular deposits of phosphorylated tau protein, the involution of basalocortical cholinergic neurons and cortical regions, and changes in neuroprotective systems) (1–5). Other changes, in contrast, appear to be more generic and are involved in many neurodegenerative diseases, such as gliosis (astrogliosis and microgliosis), increased oxidative stress, and apoptosis (1–5). Each of these complex changes are pieces of a puzzle that is so hard to put together that up-to-date we are still unable to describe a scenario in which one or more cascades of pathological events lead to AD.
Nevertheless, the constant confirmation of abnormalities, sometimes precisely located in particular brain regions, neuronal circuits or even cells, has led to different theories that – at least partially – explain AD pathogeny (1,2). The cholinergic theory (which involves the involution of the basalocortical cholinergic system) (1–3), the amyloid cascade theory (1,4), and the theory that suggests AD to be a variant of the tau diseases (5) are the most important ones. All have been instrumental in major advances in AD-related research made so far. They have also been important in the development of models for the study of AD.
The development of AD models has been somewhat peculiar, since no animal naturally suffers from AD; nor can it be induced by any endogenous or exogenous agent. The typical set of neuropathological alterations seen in human AD simply does not appear in other aging mammals. Only in a few species (e.g., dogs, polar bears, the Argentine rabbit, and primates), similarities with human AD are seen (1). However, the incidence and prevalence of disease in these animals is so low, and the clinical characteristics so different from human AD (the neuropathological symptoms seen in AD are not all present) that no good model is afforded. Clinically, the abnormalities seen in the brain functions of “pathologically aged” members of the above-mentioned species are not fully comparable to the mental and behavioral symptoms suffered by humans with AD. However, while this prevented early researchers using the classic model animals employed to investigate other diseases, the 1970s saw the development of a number of animal models designed to allow the detailed study (over time) of particular AD abnormalities. These also allowed some of the pathogenic theories regarding AD to be tested. Before the development of transgenic animals, the models that allowed the greatest steps forward involved experimentally induced lesions designed to produce cortical and subcortical abnormalities believed important in AD (e.g., involution of the basalocortical cholinergic system, alterations to the cortical and hippocampal circuits, the presence of amyloid protein, and neurofibrillary abnormalities) (6). Although theoretically simple in their design, many of these lesion-induced vertebrate models are still highly valuable. For example, models of this sort have been used to study local alterations in markers of oxidative stress, apoptosis, and synaptic function caused by amyloid β (Aβ) deposits after Aβ protein was injected into the cortex, hippocampus, or nucleus basalis of rats (7–10). Other situations require a more sophisticated design and an in-depth study of the modifications caused by the induced lesion in the different parts of the brain, as well as of its repercussions on different brain functions. For example, the study of hippocampal cholinergic denervation in rats with a selective septohippocampal cholinergic lesion produced by injecting a specific cholinotoxin (192 IgG-saporin) into the septum requires morphological, histochemical, and biochemical studies of the septum, hippocampus, and other regions be performed, as well as behavioral studies involving validated techniques (11–14). The use of these more complex models requires their prior, ample characterization if the results are to be correctly interpreted (6). The results obtained with the techniques for quantifying changes in morphohistochemical and biochemical, neuronal and glial variables in simple lesion models are not usually associated with interpretive difficulties when comparing studies or when extrapolating to humans. However, problems do arise with the more sophisticated models when the intention is to compare changes in mental functions and the behavior recorded when using different types, or when comparisons are made with altered higher functions in human AD brains (6,15–19). Several authors indicate that all tests assessing learning and memory functions, such as the conditioned eye blink reflex in the rabbit (20) and the passive and active avoidance, T-maze, and Morris water maze tests for rats (15,16,19) should be very well characterized, controlled, and validated (15).
This chapter examines the different lesion-induced, vertebrate models of Alzheimer dementia and analyses the characteristics that make them useful in the study of the pathogenic mechanisms of AD, and in verifying the efficacy of possible medications. The characteristics that make them diverge from the disease they try to represent are also discussed.
2 Types of Lesion-Induced Alzheimer Models
2.1 Types of Models
Many types of lesion models exist they can be classified depending on the region of the brain primarily or secondarily affected, the extent of the lesions produced, the intensity and duration of the neuropathological or behavioral alterations induced, the physical mechanism or chemical/biological agent employed to induce the lesion, and the route of administration of such an agent.
The brain regions selectively lesioned for the study of pathogenic mechanisms of AD usually include the basal forebrain region, from which cholinergic nerves diffusely innervate the cortex, or different cortical areas of the cerebral hemispheres and hippocampus. These regions can be lesioned surgically or by the stereotactic injection of different agents. Lesions characteristics depend on the type of agent employed and its capacity to cause selective harm to different types and subtypes of neurons, nerve fibers passing through the affected area, glial cells, and blood vessels. When a neurotoxin-lesion model is designed or selected, the target area must be well known (including its neuronal and glial types, regional neuronal circuits and their connections with other brain areas), as should the mechanism of action of the agent inducing the lesion. Both factors are of capital importance for understanding the primary and secondary induced lesions, i.e., with respect to the anatomical areas involved, the neurons and glia affected, and the markers likely to be affected. A lesion in the basal forebrain could produce changes in different areas of the cortex where basalocortical axons terminate, which of course will depend on the location and size of the lesion induced. The damage will also differ depending on whether a toxin selective for cholinergic nerves (e.g., 192 IgG-saporin) or one that affects both cholinergic and noncholinergic nerves is used (e.g., quisqualic or kainic acids) (6,11,16–19,21). A “local response” will be seen within the damaged basal forebrain region, “proximal responses” in the nondamaged structures neighboring the lesioned basal forebrain area, and “remote responses” in the ipsilateral cortex innervated by the lesioned forebrain area, in other ipsilateral and contralateral cortices, and in the contralateral basal forebrain (21,22).
Other models exist in which a toxic agent induces lesions throughout the brain, leading to mental and behavioral changes reminiscent of AD. Many authors believe that the chronic intake of alcohol or aluminum, or intravenous injections of aluminum, can induce neurochemical and neurophysiological changes in different rodents that are similar to those seen in AD (23–26). These models have been used to clarify a number of pathogenic features of AD and to assess the efficacy of certain anticholinesterase drugs.
Neurotoxins can also induce different lesional models depending on the administration route and protocol. For example, Aβ, colchicine, cholinotoxin AF64, and aluminum provide different models depending on how they are administered (6,12,18,23,24,27–30). When administered intraparenchymally they cause selective lesions, when delivered intracerebroventricularly they induce diffuse lesions in the periventricular regions (although some areas are more affected than others, such as the hippocampus, especially in rodents), and when administered orally, intramuscularly, or intraperitoneally, thereby reaching the brain via the bloodstream, they induce widespread, diffuse lesions in the cerebral hemispheres and/or the basal brain.
The following sections discuss the most widely used models, starting with those that cause selective lesions in defined brain areas. This includes two sections: one on lesions of the anterior basal brain and one on cortical lesions. Discussion is offered on the specific characteristics of these models, which are dependent on the types of neurons damaged and the type of effects the lesion-inducing agent causes, before focusing on models that might be used when administrating toxins via different routes.
2.2 Simple and Combined Models: Models in Aged Animals
Many of these models can be combined to study different combinations of pathogenic mechanisms, e.g., lesion-induced models by double injection of Aβ and oxidative stress enhancers (31), basalocortical cholinergic system lesional models combined with Aβ implantation models (in study in our laboratory) or basalocortical cholinergic system lesional models combined with cortical lesions (32). However, such complex systems have rarely been used, partially because of the greater variability in the results obtained. Many models are modified to increase the neuronal involution, to enhance neuroprotection mechanisms, or to speed recovery from the lesions induced. An example of the first of these is the potentiation of the Aβ neurotoxicity in Aβ implantation models achieved via the reduction of Aβ clearance. This can be brought about by inducing hyperhomocysteinemia through chronic methionine consumption (33). The effects of amyloid or neurotoxin-induced lesions can also be potentiated through ischemia caused by the occlusion of the blood vessels irrigating the part of the brain affected by the lesion (34).
Since no model with all the characteristics of AD is available, it cannot be claimed that any of the models developed is always the first choice in the study of AD pathogeny or in the assessment of potential treatments. However, when pathological changes are being examined, the model that directly or indirectly involves changes most similar to those seen in humans should be chosen. When curative or disease-modifying therapies are to be assessed, the model that reflects changes most similar to those seen in humans should be used. For example, cholinergic lesion models are a priority in the study of the cortical repercussions of cholinergic involution, and for testing the efficacy of anticholinesterase, nicotinic, and nootropic drugs (35,36).
2.3 Factors at the Species and Individual Level
AD models produced by lesions have different characteristics depending on the type of mammal involved, its physiopathological characteristics, and its maintenance (37–41). Lesions induced in the basal forebrain and those induced by toxins in the cerebral cortex in mammals of different orders (rodents, lagomorphs, primates, etc.) differ in their associated neuropathological and behavioral features (42). Even in different rodent species (rats, mice, guinea pigs), nucleus basalis magnocellularis (nbm) lesions induced by Aβ or toxins can produce models with quite different characteristics (38). The rabbit is much more sensitive to aluminum and okadaic acid insult than rodents, and neuropathological alterations more like those of human AD can be obtained in this species. On the contrary, the neuropathological and behavioral changes seen in rats with nbm lesions are more similar to those seen naturally in aged rabbits than in humans with AD.
The characteristics (e.g., age) and maintenance (e.g., housing conditions) of the animals are very important when configuring the intensity of the lesion and its progress (38–46). Older animals are generally more sensitive to lesion induction and experience more intense cortical cholinergic involution than younger animals with the same dose of neurotoxin (21,47–50). Further, many lesion-induction protocols in which short- or long-term recovery occurs in younger animals achieve no such effect in older animals (21,47). Naturally, this is because of the diminished plastic and adaptive capacity of aged neurons (51). It should also be remembered that aged animals show an array of histochemical (e.g., an increase in oxidative stress and apoptosis) and cellular (especially, glial) changes compared with young animals (52–58). When studying aged animals, it should be taken in mind that many of the cellular changes associated with normal aging are also seen in AD.
The term “aged animal” is quite imprecise and needs to be applied correctly in each species. Many studies have considered animals far from the ends of their lives as aged. In many strains of Wistar rat, cortical cholinergic involution and gliosis do not appear until animals are 24–30 months old (their life expectancy is some 36 months). Between the ages of 3 and 20–24 months, no significant age-related differences are seen in the response to excitotoxic lesions produced in the basal brain or the cortex. However, many models involving animals less than 3 months of age have very different characteristics to those involving adult animals. Young animals are capable of far more plastic change than older animals, and, therefore, any extrapolation of observations in young animals should be made with extreme caution.
The model to use should be chosen with the aim of the study in mind, which might be to determine the effects of some variable on a particular anatomical region, on a system, on a neurotransmitter, or on a cellular mechanism. In addition, variables of the model itself should be monitored to confirm that it has behaved correctly; this should allow comparisons with previous studies.
If results are to be valid, the lesioned animals must show homogeneous morphological and functional behaviors. In many models, this is achieved with respect to all the changes induced, while in others it is not. Individual factors that can condition the intensity of the lesion produced and its evolution (including short- and long-term recovery or increasing involution) have been studied scarcely. It would appear logical that they should be related to the individual’s capacity for neuroprotection at the time a lesion is induced. In humans too, each individual has characteristics that affect the progress of AD, rendering the pathological process at work in them slightly different. This can be very important in the pathogeny and treatment of the disease. It would therefore be of great interest to study the causes of these individual variations in models; the results could throw light on the pathogeny of AD, and perhaps open up new therapeutic possibilities. Individual variation is more important in some lesion models than others. A clear example of this is seen in the different long-term outcomes in models with excitotoxic lesions in the basal brain.
Kainic acid, when administered subcutaneously or intraperitoneally to produce models of epilepsy or AD, produces a series of functional and neuropathological changes that vary depending on the dose and on interindividual differences in, e.g., APP and tau content of cells, and in the extent of gliosis and apoptosis (59). The reason for these variations, however, is unknown. In our own work, in which we have followed rats with different nmb lesions for up to 3 years (21), we have observed a number of important relationships between the changes induced in different individuals and their progress. An important characteristic in some models is the fact that, after a short time (hours or days), facilitation of morphological (neuronal reinnervation) and functional (the reassignment of functions among the remaining circuits) recovery may occur. These changes are very important when trying to assess the efficacy of neuroprotective medications or neuroplasticity treatments. In such cases, models in which no spontaneous recovery occurs should be used.
The housing provided can also modify the progress of lesioned experimental animals (even aged lesioned animals). Animals maintained in enriched environments show a greater capacity to recover or at least increase their synaptic contact count in different cortical regions (54).
3 Alzheimer Models Produced by Lesions or Dysfunction of the Basal Forebrain: The “Cholinergic” Models
Different types of lesion or dysfunction of the forebrain cholinergic neurons have been provoked to produce a wide variety of lesion-induced vertebrate models of AD. Some involve highly selective and focal cholinergic alterations in restricted areas of the basal forebrain, while others entail more general (cholinergic and noncholinergic) damage to large areas of this anatomical region. Many studies have been performed on the cellular (neuronal and glial) and molecular modifications promoted by lesions in the basal forebrain (local responses), as well as in selected cortical areas (remote responses), the latter alterations mainly caused by cholinergic denervation following damage to the basal forebrain neurons. Lesions in the septum provoke higher cholinergic hypofunctioning in the hippocampus and amygdala. Neuronal damage in the diagonal band of Broca provokes cholinergic alterations in the gyrus cingularis and other limbic structures. Manipulation of the neurons of the nmb leads to cholinergic changes mainly in the neocortex. Cognitive deficits and altered behavior associated with these models have been studied in many settings. As discussed later, the outcome of these models greatly varies depending on the used methodology. The best known and used types are summarized below after a brief commentary on the characteristics of the basal forebrain region.
3.1 The Cholinergic Centers of the Basal Forebrain
The basal forebrain (Fig. 1) is the anatomical region ranging from the septum to the midbrain, which passes under the anterior commissure and groups together both the telencephalic and diencephalic structures. The special characteristics of the “cholinergic centers” in the basal forebrain are largely responsible for the features of the cholinergic models of AD produced by lesion or dysfunction in this region. To understand the cholinergic impairment generated by different basal forebrain lesions, as well as the morphological, biochemical, and behavioral effects induced in brain cortical areas, in-depth knowledge of the basal forebrain of the model species is required.
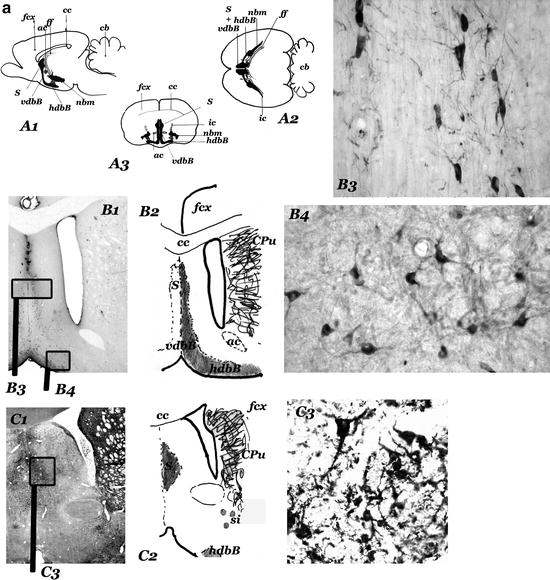
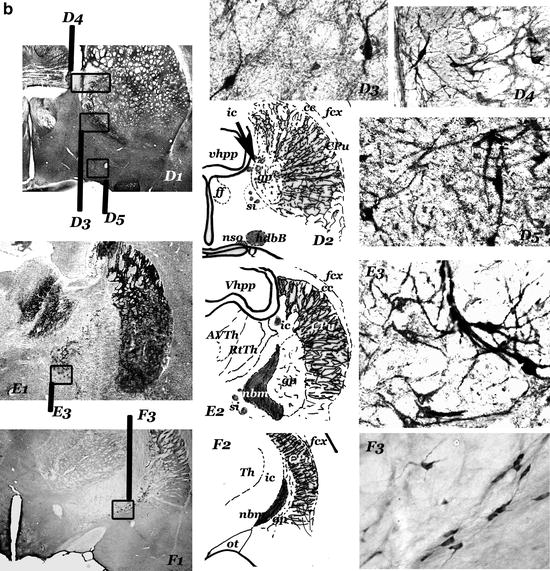
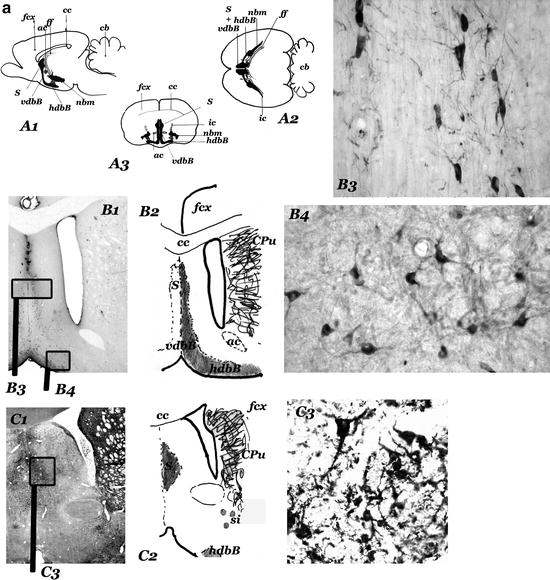
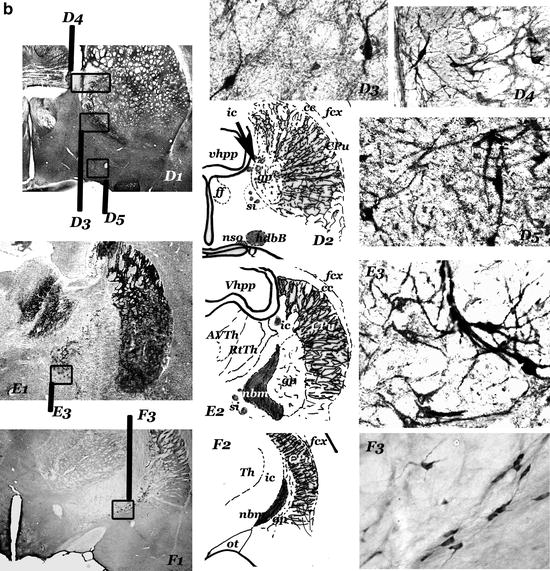
Fig. 1.
(Panels 1a and b) Cholinergic “centers” and neurons of the anterior basal forebrain of rat.
(a) Diagrams showing the sagittal (a1), horizontal (a2), and frontal (a3) projections of the cholinergic centers-septum (s); vertical (vdbB); and horizontal (hdbB) regions of the diagonal band of Broca; nucleus basalis magnocellularis (nbm). In a2, the projection of the anterior part of the fimbria/fornix (ff) is represented in the right hemisphere and the projections of the internal capsule (ic) is represented in the left hemisphere. “Septum” (s) – different groups of neurons located in the septal region of the brain – and diagonal band of Broca (dbB) form a continuum of cholinergic cells (observed in b, c, and d). The nucleus basalis magnocellularis (nbm) is difficult to define. Their cholinergic neurons are located near the internal capsule (ic) and close to the globus pallidus (gp), many of them entering these structures. nbm starts near the anterior commissure (ac) and ends near the optic tract (ot), where the globus pallidus disappears (d, e, f). hdbB and nbm are connected by a “network” of isolated cholinergic neurons located in the “substantia innaminata” (a region ventral to the pallidal structures). (b–f) Panoramic views (b1–f1), diagrams (b2–f2), and cholinergic neurons corresponding to frontal sections at different anteroposterior levels (b = + 0.5 mm; c = – 0.5 mm; d = – 0.7 mm; e = – 1.3 mm; f = – 2.5 mm; the 0.0 mm the plane passes through the bregma–Paxinos and Watson Atlas (229)). At these levels, s and dbB are observed in b, c, and d and nbm (from the most anterior part to the posterior end) in d, e and f. In b, the section has been incubated with a ChAT monoclonal antibody and in f, with a NGFr monoclonal antibody. c, d, and e are AchE histochemical stained sections. b3 and c3 show different types of septal cholinergic neurons. b4 and d5 show cholinergic neurons of the vdbB and hdbB, respectively. In d, different types of cholinergic neurons in neighbouring areas of the nbm can be observed: cholinergic neurons in the substantia innominata (d3), and in the internal capsule (d4, left) and in the globus pallidus (d4, right). The magnocellular neurons of the central regions of the nbm can be observed in e3; they are located in the “ventral pallidum,” entering the globus pallidus (gp) and the internal capsule (ic). Other cholinergic neurons are located in ventral areas (si). The most posterior neurons of the nbm, close to the optic tract can be observed in f3. (a1–3 = × 2; b1–f1 = × 4,5; b3–4, c3, d3–5, e3 and f3 = × 45).
Fig. 1. (continued) ac, anterior commisure; AVth, anteroventral Thalamus; cb, cerebellum; cc, corpus callosum; CPu, caudate putamen; dbB, diagonal band of Broca; fcx, frontoparietal cortex; ff, fimbria/formix; gp, globus pallidus; hdbB, horizontal region of dbB; ic, internal capsule; nbm, nucleus basalis magnocellularis; ot, optic tract; RtTh, reticular nucleus of the thalamus; s, septum; si, substantia innominata; Th, thalamus; vdbB, vertical region of dbB; Vhpp, ventral hippocampus.
Shute and Lewis (60,61) and Krnjevic and Silver (62) were the first to describe the cholinergic component of the forebrain in normal and experimental animals. The cholinergic centers are bilateral areas with a high density of cholinergic isodendritic neurons that show no defined cytoarchitecture or well-defined limits. Classically, three cholinergic groups (“centers” or “nuclei”) from the anterior-medial to the posterior-lateral area of the basal forebrain are contemplated: the medial septal nucleus, the nuclei of the diagonal band of Broca (dbB; with a vertical and a horizontal set of neurons), and the nbm in rodents, or the nucleus basalis of Meynert (nbM) in primates (including man) (63–65). In the anterior area of the basal forebrain, the first cholinergic group of each brain hemisphere is located in the septum, a medial structure of the brain. The two groups, the left and the right cholinergic septal centers, are topographically very close to one another and septal lesions are always “bilateral” in their effects. In posterior areas of the basal forebrain, the centers are more laterally displaced and lesions at these levels will cause “unilateral” effects. However, the neurons of the posterior areas of the dbB and the nbm/nbM are more intermeshed with the neurons of neighboring brain structures. Laterally, they are in close relationship with the amygdala and the medial area of the temporal lobe dorsally with the globus pallidus and the striatum, and ventrally with the hypothalamus. This general arrangement varies to some degree from species to species. The most outstanding of these neuronal centers are those in which large cholinergic neurons appear. In areas of the basal forebrain of some species, the density of certain kinds of neurons is sufficiently great to contemplate the existence of neuronal centers or gray nuclei, although morphological characteristics, such as the poor definition of the borders and the high number of intermeshed neurons and fibers from neighboring anatomical regions, may hamper interpreting some neuronal groups in this way.
The nucleus basalis is extremely difficult to define in both primates and rodents. Cholinergic neurons presumably belonging to this nucleus are located near the internal capsule and close to the globus pallidus, many of them entering these structures. However, other important clusters are also laid down in areas ventral to the pallidum-striatal complex. These anatomical areas are very poorly defined and referred to by many histologists as the ventral pallidum and substantia innominata. The nbm (or nbM), or their cholinergic neurons, can be understood to start near the anterior commissure and to end near the optical tract where the globus pallidus disappears in serial coronal sections.
Histochemical research on the cholinergic groups of the basal forebrain performed since 1980 have provided a new definition of the cholinergic sectors, the demonstration of different sets of cortical projecting neurons with neurotransmitters other than acetylcholine (ACh) intermeshed among the cholinergic neurons, and a better understanding of the cortical targets, as well as the relationships between cholinergic groups and neighboring extra forebrain structures. Mesulan et al. (66,67) proposed an alternative nomenclature for the cholinergic sectors of the basal forebrain in primates and rodents. These authors describe six sectors (Ch1–Ch6) in the basal forebrain. Sectors Ch1 and Ch2 – the medial septal nucleus and the vertical limb nucleus of the dbB in traditional nomenclature – are the major sources of cholinergic innervation for the hippocampus. Ch3, which mainly innervates the olfactory bulb, corresponds to the lateral part of the old horizontal region of the dbB. Ch4 contains the broadest group of cholinergic neurons, since it includes not only the traditional nbm/nbM and both lateral parts of the vertical region plus the medial section of the horizontal region of the dbB, but also the cholinergic groups or isolated cholinergic neurons observed in the neighboring areas (globus pallidus, substantia innominata, and ansa lenticularis) plus the preoptic nucleus. Ch4 neurons innervate neocortical areas and the amygdala. Sectors Ch1–Ch4 provide an extensive and continuous system of cholinergic neurons that give rise to a topographically organized cholinergic regulatory projection system, which innervates the entire neocortical mantle, as well as many limbic and olfactory structures (i.e., “the ascending cholinergic system”) (60,65,66,68). All these projecting neurons show the highest density of p75 NGFr (the low-affinity nerve growth factor receptor) (69,70). In primates, Ch4 can be divided into subsectors depending on the cortical targets, but in rodents this division is less clear. Two other cholinergic sectors, Ch5 (contained mostly within the pedunculopontine nucleus of the pontomesencephalon) and Ch6 (within the periventricular tegmental area), provide cholinergic innervation to the thalamus and cannot be included in the ascending cholinergic system. However, these two neuronal groups are essential components of the ascending reticular activating system and could indirectly regulate cortical activity via a noncholinergic system between the thalamus and cortex. All the cholinergic groups or sectors of the basal forebrain and upper brain stem are reciprocally connected with the thalamus, neocortex, hippocampus, and limbic structures, as well as with one another.
Clusters of noncholinergic neurons are intermingled with cholinergic neurons in certain areas of the basal forebrain (71–78). The best-characterized neurons are those that show immunoreactivity against γ-aminobutyric acid (GABA) (74,78), galanin (alone or in cotransmission with ACh) (72), or peptides such as somatostatin (75,77), neurotensin, or enkephalins (73,75). These noncholinergic neurons have precise projections to different cortical areas such as the GABA set of neurons of the septum, innervating the hippocampus, or the neurotensin basalis group that innervates the frontoparietal cortex. The death or dysfunction of these noncholinergic neurons of the basal forebrain might explain the different symptoms seen in several mental disorders. Some of the cognitive deficits produced in experimental animals with basal forebrain manipulations may also be related to the damage of these noncholinergic cells; this might account for the problematic results returned by some basal forebrain lesion models.
All of the cholinergic and noncholinergic projecting neurons described earlier are considered by Alheid and Heimer (79) as one complex – the “magnocellular corticopetal complex” – of the three morphofunctional complexes into which the basal forebrain as a whole is organized. The other two complexes are the “striatopallidal system” and the “extended amygdala.” The three systems have neurons that are closely intermeshed in the basal forebrain. The striatopallidal system is a set of epidermal growth factor-immunoreactive neurons located in the basal forebrain that form a rostroventral extension of both the caudate-putamen and globus pallidus. The extended amygdala groups all the neurons immunoreactive against angiotensin II from the temporal lobe to the stria terminalis; the highest density of these neurons is seen in the centromedial amygdaloid complex and the bed nucleus of the stria terminalis. The magnocellular corticopetal system, which is composed of all the cholinergic and noncholinergic neurons projecting to the different cortical areas, is interwoven with these other two systems, with no clear boundaries apparent. A very large number of connections among these basal forebrain systems exist. All the corticofugal pathways and corticopetal feedback loops of the basal forebrain systems are thought to be of great importance in neuropsychiatric disorders (79). The rough rostrocaudal topographical organization of the cholinergic projections to the cortex that characterized the first descriptions of the basal forebrain (64,80), has been modified to reflect functional models of this brain region, in which a more diffuse cholinergic innervation from the basal forebrain to the cortical and noncortical regions is contemplated (68,81,82). On the basis of the permanent interrelationship between these cortical and basal structures, several theories on learning and memory have been proposed, including the “hippocampal memory indexing theory” (83). In this theory, the cholinergic centers control the processing and encoding of information stored in neocortical modules indexed in the hippocampus. The diffuse topographical organization of the basal forebrain’s cholinergic projection into the cortex allows both forebrain neurons to randomly innervate different cortical areas and some cortical areas to receive cholinergic terminals from neurons located in different regions of the basal forebrain. This diffuse cholinergic innervation suggests that cortical innervation is widespread but largely undifferentiated; the functional importance and significance of this is, however, unknown.
Most of the axons of the cholinergic neurons in the septal areas run through the fimbria/fornix to the hippocampal formation. The axons of the neurons of the dbB form a diffuse projection to the limbic and neighboring brain regions. The axons of the nbm/nbM project to the lateral part of the corpus callosum and enter it. Different axon collaterals run from this body and penetrate the different areas of the ipsilateral neocortex; a few cross to the contralateral side. Some axon collaterals of the nbm/nbM run to other neuronal subcortical centers. This special architecture allows the surgical manipulation of the main cholinergic efferents of the septum possible, although it is very difficult. The results obtained can be very variable when lesions are induced with physical or chemical agents in other cholinergic centers. Transection of the fimbria/fornix produces not only cholinergic denervation of the hippocampus, but also interrupts the limbic circuits in the connection between the hippocampus and mammillary bodies. This should be remembered when cognitive and behavioral tests are chosen to evaluate cognitive impairment in AD models produced by fimbia/fornix lesions.
A dense network of cholinergic fibers is seen in the cortex (Figs. 2b and i). The cholinergic fiber terminals show specific patterns within each cortical area and layer. Axon terminals end at neurons of both intrinsic and extrinsic cortical circuits and blood vessels. The nicotinic and muscarinic cholinergic receptors also show a laminar distribution. All cortical neurons respond to ACh. All this suggests the existence of a functional cholinergic organization in the cortex. The fibers belong to neurons of different origin, including local cholinergic neurons. The results of different morphofunctional studies have suggested that the cholinergic system is not a selective activating system, but rather a reticular, diffuse system for globally modulating cortical functions (81,84).
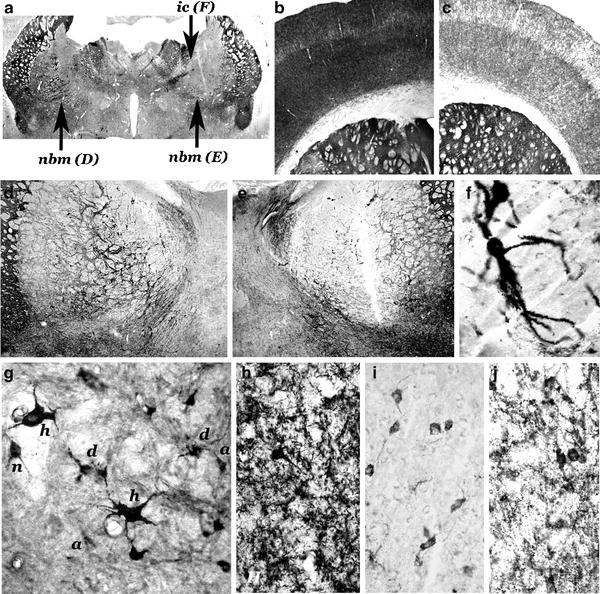
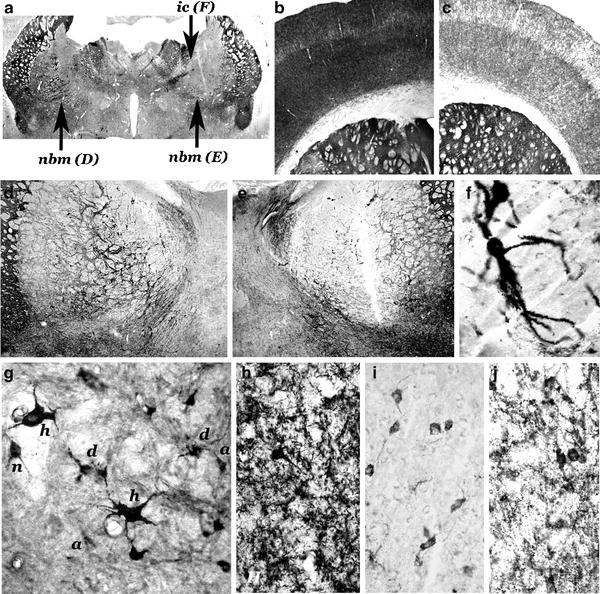
Fig. 2.
Morphohistochemical changes in lesion-induced models of AD in rats.
(a–f) Unilateral lesion induced in the nbm of the right hemisphere by a stereotactic injection of 50 nmols of quisqualic acid; 45 days postlesion induction of the lesion. Coronal sections; AChE enzymohistochemical staining. An intense decrease in AChE-positive neurons is observed in the lesioned side (a, e) in comparison with the non-lesioned side (a, d). A very few number of AChE-positive neurons can be observed in the neighbouring areas of the lesion core, such as the two hypertrophic neurons located in the internal capsule (a, ic; f). Intense AChE-positive network in the motor and somatosensory frontoparietal cortex of the contralateral unlesioned side (b) and weak AChE histochemical staining of the ipsilateral cortex (c). (g) Unilateral lesion induced in the nbm by an injection of 30 nmols of quisqualic acid; 7 days of post-lesion evolution, NGFr immunostaining. Different types of surviving AChE-positive neurons lateral to the “core” of great necrosis: normal (n), hypertrophic (h), atrophic (a), and dystrophic (d) neurons. (h–j) Unilateral lesion induced in the nbm by an injection of 40 nmols of ibotenic acid; 90 days postlesion induction. A dense network of AChE-positive fibers in the layer V of the motor frontoparietal cortex of the contralateral non-lesioned side (h) of one animal, and a negative (i) and a very weak AChE positive (j) areas of the cortex ipsilateral to the lesion of two different animals of this model. AChE histochemical positivity strongly increases in cortical non-pyramidal neurons in the ipsilateral side (f, j). (a = × 1.5; b–c = × 5.5; d–e = × 10; f–j = × 45) (methods in (6,14,28)) (Reproduced from Current Alzheimer Research, 2004, with permission from Bentham Science Pub. Ltd (6)).
In summary, the basal forebrain is an anatomical region in close relationship with pallidal, striatal, amygdalar, and hypothalamic structures, in which a magnocellular corticopetal system exists. In this corticopetal system, the most important sets of neurons regulating cortical functions are cholinergic. These neurons can be grouped into three functional cholinergic groups, similar to, but more diffusely located, than the classic nucleus of the septum, diagonal band, and nucleus basalis. Each group specifically ends in a part of the cortex with good topographic correlation, although diffuse innervation also exists. Intermeshed with these cholinergic neurons are noncholinergic neurons with GABA, neurotensin, somatostatin, and galanin as their neurotransmitters. Selective lesions only affect a restricted part of the cortex in a direct fashion, but different collateral axons can provoke modifications in other basal centers. The cortical effect of a cholinergic lesion or dysfunction (especially when evaluated by cognitive tests) is the result of the direct effects on the cortical innervation and on the innervation related to modifications in connected centers, or of damage to neighboring noncholinergic neurons.
3.2 Main Types of Cholinergic Models Produced by Basal Forebrain Lesion or Dysfunction
3.2.1 AD Models Produced by Fimbria/Fornix Transection (Septohippocampal Cholinergic Denervation) (85–87)
Cholinergic neurons of the septal area of the basal forebrain innervate the hippocampus. Their axons reach the hippocampal formation in the fimbria/fornix, a well-defined anatomical structure easily accessible to surgical manipulation. Different AD models can be obtained after unilateral or bilateral, total or partial transection of the fimbria/fornix. Different degrees of cholinergic impairment in the hippocampus and in the basal forebrain have been obtained, as have different alterations in the performance of selected cognitive and behavioral tasks. These models have been very useful in the study of the different roles of cholinergic inputs on hippocampal function in different species. Moreover, this kind of model has been widely used to study various neuronal involutive and adaptive processes, such as neuronal degeneration and survival after axotomy, the free and induced regeneration of axons, and the ability of adult axons to innervate new brain tissue. Many factors (species, age, neurotrophins, and other lesions) regulate the development of the deficits induced by a fimbria/fornix lesion, and recovery varies in the different models. Morphofunctional cholinergic and noncholinergic involution and recovery, and cognitive impairment and recovery, have not always been recorded as parallel processes. Controversy exists regarding the cognitive functions of the hippocampus and the role of the cholinergic system in the regulation of these functions. Morphofunctional recovery is different in different species. Monkeys studied 1.5 years after fornix transection (88) show essentially the same pattern of hippocampal cholinergic fiber loss as do those that have survived less time. This indicates that the residual choline acetyltransferase (ChAT)-immunoreactive fibers, many of which reach the hippocampal formation through a ventral cholinergic pathway, are incapable of reinnervating the denervated portions of the hippocampal formation. This appears to distinguish the monkey from the rat, for which substantial sprouting and reinnervation of cholinergic fibers has been reported after the induction of similar lesions (87,89). The ability of the lesioned axons to innervate grafts surgically implanted in the fimbria has also been studied (89). After performing unilateral transections in adult rats, foetal hippocampus or autologous peripheral nerves have been implanted into the transection site and the regeneration of the fimbria fibers analyzed (87). Prominent innervations by central acetylcholinesterase (AChE)-staining axons were seen after 2–3 weeks in both types of implant; this was sustained until at least 8 months. Reinnervation of the adjacent host hippocampal terminal zone was also apparent, but was sparse compared with the innervation in the implants.
3.2.2 AD Models Produced by Basal Forebrain Electrolytic Lesions (90–93)
These kinds of lesion-induced models, in which the death of neurons over wide areas of the basal forebrain was produced as well as alterations to fibers passing by the target area, were practically abandoned several years ago. However, some modern studies combining these and other types of lesion have provided results useful for differentiating between cholinergic and noncholinergic effects (90–93). This kind of lesion has been used in newborn animals (93).
3.2.3 AD Models Produced by Basal Forebrain Excitotoxic Lesions (6,21,22,94–103)
In these models, nonselective cholinergic lesions/dysfunctions of the forebrain cholinergic centers, including septum (94,95), dbB (94) and nbm/nbM (6,21,22,96–103) (Figs. 2–5), are induced by stereotactic injections of excitotoxins, which leads to the dysfunction and/or the death of the neurons around the injection site. In selected studies, excitotoxins have been administered by i.c.v. injection; this induces lesions in the basal forebrain of less importance than those seen at cortical level (mainly in the hippocampus). Neuronal damage is produced by the disturbance of ionic homeostasis at the cellular level. Neurotoxic changes induced by abnormal Ca2+ influx also occur (104). Theoretically, this type of basal forebrain lesion does not cause alterations to fibers passing through the target area. The excitotoxins are not cholinergic-selective, but these models are associated with important cortical cholinergic dysfunction or hypofunction with no other apparent important deficits. The most widely used excitotoxins are substances that act as agonists on the different glutamatergic receptors, such as N-methyl-d-aspartate (NMDA) on NMDA receptors, and ibotenic (IBO), quisqualic (QUIS), α-amino-3hydroxy-5methyl-4isoxazole-propionic (AMPA), and kainic acids on non-NMDA glutamatergic receptors. Not all of these excitotoxins have the same effect; great differences in morphological, histochemical, and biochemical cortical alterations, as well as cognitive and behavioral alterations (22,27,36) have been observed in apparently similar models. Further, in individual experimental animals of certain models, the recovery of functional deficits sometimes occurs (6,16,105). The reasons for these differences – most of which have not been well studied – include: (a) the existence of different subtypes of glutamate receptor on cholinergic and noncholinergic neurons in the target basal forebrain area (106), (b) the existence of different subsets of noncholinergic neurons affected by the toxins in the lesion area (or neighboring damaged regions) (73–78), (c) differences in the innervation of brain areas other than the main target zones of the damaged cholinergic neurons (79), (d) poor correspondence between the behavioral task performed and the cognitive function assessed, and (e) the poorly understood role of the cholinergic system in cognitive function (81,107,108). Other neurotoxins (not specifically cholinergic) have occasionally been administered by stereotactic injection into the basal forebrain, such as quinolic acid (109), a natural metabolite of tryptophan (109), colchicine (28,110,111), and other vinca alkaloid substances that bind tubulin and disrupt proximodistal (axoplasmic) intraneuronal transport (112).
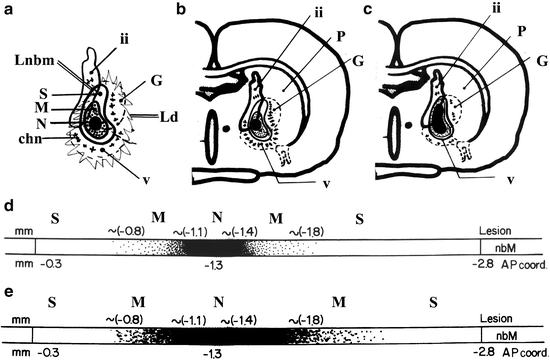
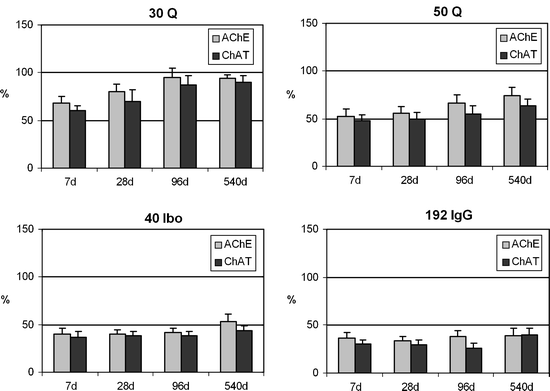
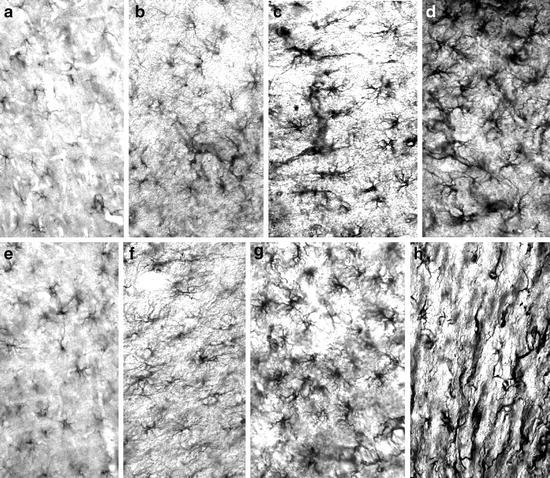
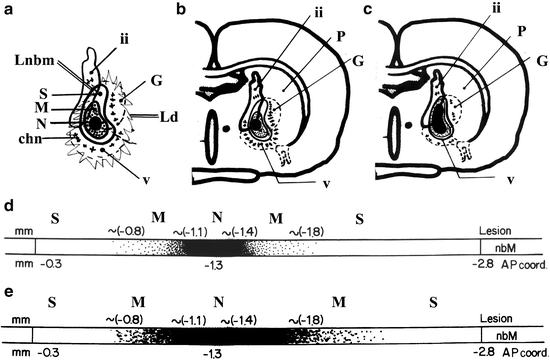
Fig. 3.
Diagrams of the damaged nbm areas after excitotoxic injection.
(2-a) The injection of neurotoxins into the main region of the nbm generated superimposed areas with different degrees of neuronal damage: a “core” of great necrosis, with a very small number of surviving cells (N), a region with mixed necrotic and surviving cells (M), and an area with a high number of surviving cells (S). The dimensions of these areas depend on the type and dose of toxin used and other individual factors (see text). The surviving neurons show different sizes, shape, and reactivities. Surrounding the limits of the nbm (Lnbm), cholinergic neurons are seen in the upper part of the internal capsule, globus pallidus, and infrapallidal areas (chn). In some models, these show hypertrophic/hyperreactive characteristics. Noncholinergic neurons of the basal forebrain and regions other than the nbm are also damaged (Ld = diffuse limit of possible neuronal damage). (2-b, c) Diagrams showing the effect of mild (30Q in B) and serious (60Q in C) nbm lesions in the coronal plane, 1.3 mm. (2-d, e) Anteroposterior representation of cholinergic damage in the nbm for these two models (areas N, M and S). The cholinergic neurons of the anterior and the most posterior part of the nbm are always preserved. Ap coord = anteroposterior coordinates from the Atlas of Paxinos and Watson (229).
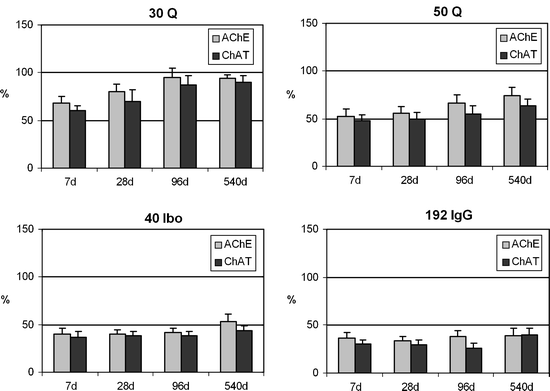
Fig. 4.
Changes (up to 540 days postlesion induction) of biochemically determined cortical AChE and ChAT activities in the frontoparietal cortex of rats after different unilateral lesions in the nbm.
Activities ipsilateral to the nbm lesion are represented as a percentage of the activity on the contralateral unlesioned side. A rapid and almost total recovery in the model obtained by injection of 30 nmols of quisqualic acid (30Q) and a slow and partial recovery after 50 nmols (50Q) are observed. Non-recovery in the models obtained by injection of 40 nmols of ibotenic acid (40 Ibo) or 5 pg of 192-IgG-saponin is shown. The histochemical AChE-positive neuronal loss in the nbm was 60.2 ± 4.2; 72.2 ± 4.0; 74.6 ± 3.6 and 81.1 ± 2.8 in the 30Q, 50Q, 40Ibo, and 192 IgG, respectively. (n = 12 animals/model/postlesion day. Methods described in (28)).
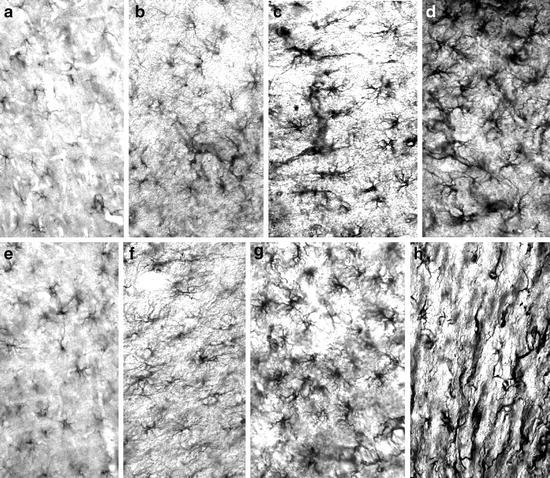
Fig. 5
Photomicrographs of GFAP immunoreactivity in rats of a lesion-induced AD model by unilateral stereotactic injection of 50 nmols of quisqualic acid.
All the sections were simultaneously incubated to show the differences in astroglial GFAP immunoreactivity. (a–c) Frontoparietal cortex, motor area layer V of 4-month-old rats, 30 days (a = unlesioned contralateral side; b = lesioned side) and 540 days (c = lesioned side) after nbm lesion. (d) nbm lesion area, 30 days post-lesion. (e–g) Frontoparietal cortex, motor area layer V of 20-month-old rats, 30 days (e = unlesioned contralateral side; f = lesioned side), and 540 days (g = lesioned side). (h) Cortical scar produced by the needle; 180 postlesion days. × 45. Methods described in (28).
Excitotoxins are microinjected (0.2–0.5 μl) using Hamilton syringes manipulated in a stereotactic apparatus. Both unilateral and bilateral cholinergic-impaired models can be produced after unilateral or bilateral stereotactic injection. Series of injections can provoke different degrees of cholinergic deficits in the cortex. Lesions in the dbB or the nbm on one side of the brain provoke high ipsilateral denervation, but only very small contralateral changes (Fig. 2). However, injections into the medial septum provoke bilateral effects since the toxin acts on the left and right septal cholinergic neurons. Unilateral models have the advantage of an internal control – the undamaged contralateral forebrain system.
Most excitotoxic models have been developed in rodents, mainly rats, but other species have also been used. Rabbits could provide an interesting new model since selective damage to the nbm leads to the deposition of Aβ (113), something not seen in other animals. A very small number of studies have been performed in nonhuman primates. In these mammals, the basal forebrain lesion is generally produced by immunotoxins rather than excitotoxins (114). The main reasons for the limited use of these models have been reviewed by Wenk (115). Briefly, the basal forebrain lesions induced are sensitive to cholinergic antagonists but show no response to agonists. Moreover, the memory deficits are small and do not correlate with the degree of cholinergic cell loss, spontaneous recovery occurs in a very short time, and cognitive impairment is more related to attention deficits. Even so, healthy, aged monkeys are often the first choice for testing drugs (116–118) or when studying amyloid production in neurodegeneration (119).
3.2.4 AD Models Produced by Cholinotoxic Lesions
In these models, selective cholinergic lesions/dysfunctions of basal forebrain cholinergic centers are produced by stereotactic injections of chemical (ethylcholine aziridinium – AF64A-) (115,120–125) or immunochemical (192 IgG-Saporin) (11–13,126,127) cholinergic toxins. These models have been deemed among the best representatives of AD, since they theoretically preserve noncholinergic neurons located in, as well as axons passing through, the targeted cholinergic centers.
The cholinotoxin AF64A binds to the high-affinity choline uptake system (HACU) and impairs cholinergic neurons. Theoretically, this neurotoxin is very selective in its production of cholinergic models, but its use is now restricted because of the high variability of the induced deficits. It has been administered intracerebroventricularly and intraparenchymally and very different results have been obtained at different doses in different brain sites (115,120–125). All neurotoxins show a more or less strict correlation between dose and effect in cholinergic cells, but AF64A has unexpected and variable effects. Certainly, high dose i.c.v. injections (>2 nmol) result in widespread histological damage to cholinergic neurons in many cortical and basal forebrain regions (e.g., neocortex, hippocampus, septum, and nbm/nbM). However, when low doses (1–2 nmol) are administered, only subsets of cholinergic cells with long projection axons are affected (mainly in septum, dbB, and nbm/nbM); the cholinergic interneurons of most regions are not affected. Moreover, cholinergic damage varies in these damaged forebrain centers and their corresponding targets to such an extent that, in different forms of this type of model, cholinergic neuronal death, short-term cholinergic dysfunction, and cholinergic recovery can all be observed. The alterations in the cholinergic septohippocampal system are smaller and reversible after only a short time (127), perhaps because of a septal compensatory effect. After i.c.v. infusion, some models show deficits on certain cognitive tasks designed to test memory, learning, or attention. Memory, however, recovers after a short time (128). Probably, the best behavioral tests for measuring cognitive deficits in these models are those related to spatial cognition (129). Intraparenchymal injection of AF64A (123,124) produces gross tissue disruption and perhaps damage to noncholinergic neurons (in the injection site and neighboring areas) (130). To obtain specific cholinergic models with aziridinium compounds, careful study of the dose-related effects (taking into account that there is a very narrow dose range for producing specific effects (125)) at each of the desired specific targets will be needed. The study of the different subsets of cholinergic neurons or of the different characteristics of the cholinergic system of different neurons in each forebrain region could be studied using these models. When the theoretical and practical problems associated with their use are minimized, they might be used to study different aspects of the effects of cholinergic drugs (131) or neurotrophic factors (132).
The basalocortical cholinergic neurons are heavily dependent on a specific neurotrophic system, thereby allowing selective cholinergic lesion-induced models of AD. The maintenance of the morphological and functional characteristics of the basalocortical cholinergic cells (69,70) is a function of nerve growth factor (NGF); the low affinity NGF receptor, or p75 NGFr, is the key point at which NGF exerts its effect. With this knowledge, a specific immunotoxin (192 IgG-saporin) was constructed by conjugating a monoclonal antibody against p75 NGFr (192 IgG) to a neuronal toxin, the ribosome-inactivating protein extracted from the plant Saponaria officinalis (saporin) (133). This complex molecule selectively and irreversibly binds to p75 NGF receptors. It is then endocytosed and transported to the neuronal cholinergic bodies where its ribosome-toxic effect is exerted and protein synthesis is impeded (133,134). Depending on the dose of immunotoxin provided and the route of administration, important dysfunction or even destruction of the cholinergic basal forebrain neurons can be achieved. The theoretical selectivity of the primary damage induced in cholinergic neurons in 192 IgG-saporin models has led to their widespread use. 192 IgG-saporin has been used to study the short- and long-term morphofunctional and cognitive impairments induced by cholinergic involution in normal and diseased adult animals of both sexes (135), in newborn animals (136) and in aged animals (50). As with the AF64A toxin, this immunotoxin can be injected into the forebrain parenchyma or the target cortical areas, or i.c.v.-administered (137). When injected into different areas of the forebrain, primary septal, dbB and/or nbm/nbM lesions, and secondary cortical alterations can be produced. I.c.v.-administered, primary lesions in all areas of the basal forebrain (of different intensity) have been induced together with intrinsic and extrinsic cholinergic cortical alterations and cerebellar lesions, since Purkinje cells also have p75 NGF receptors (126). Different mammal species, including primate species, have been used to obtain AD lesion models using 192 IgG-saporin. The injection of the immunotoxin into different regions of the basal forebrain produces models with selective cortical cholinergic deficits (11,12,126,127) (Fig. 4). In general, noncholinergic neurons are not affected, but on many occasions nonspecific structural damage occurs, mainly long after the lesion is induced. No local basal forebrain damage other than neuronal cholinergic loss is seen at 1–5 months after lesion induction, but at 11 months, extensive tissue loss is observed at the lesion site(s). Cognitive deficits produced by immunotoxin injection into the basal forebrain have been described as being of variable intensity and long development and to depend on the coexistence of other lesions. In a study comparing the long- and short-term effects of single and combined septum/nbm immunotoxic lesions (38), no important behavioral deficits in spatial learning or memory in the radial arm and water maze tasks were appreciated. Only double lesion (septum + nbm) rats showed behavioral impairment; single lesion (septum or nbm) rats remained behaviorally normal. The authors of this study suggest that deficits appear only when damage is intense and when selective cholinergic and nonselective noncholinergic alterations have been produced. Despite the many studies that have used these immunotoxin models and the importance of their results, many discrepancies exist between the cognitive and morphofunctional deficits recorded. The variability of the cognitive responses recorded after theoretically similar lesions have been induced, is related to the different means of evaluating cognitive impairment and the size of the initial immunotoxic lesion (138–141). The different cortical responses of neocortical and limbic structures (123,124,142,143) are also factors that condition the development of deficits after nbm/nbM and septal lesions. Immunotoxins and AF64A have been also used to assess the morphofunctional changes of selected brain areas (e.g., suprachiasmatic nucleus) after cholinergic denervation (144). As seen after partial or total excitotoxic lesions of the forebrain nuclei, partial or total cortical cholinergic impairments, as well as partial or total recoveries of the cholinergic deficits and cognitive alterations, have been described in these cholinotoxic lesion-induced models (127,142,143). “Partial” models have been useful in studying cortical cholinergic involution, as well as natural and induced regeneration or neuronal reorganization (127,145). The effects of neuronal grafts (146) or drugs (e.g., anticholinesterase drugs (147,148) and NGF-like factors (149)) designed to induce cortical cholinergic or cognitive recovery have been tested with these models. The rat has been the animal most used, but others species have been employed in some studies. Many rodents have been used to compare cognitive differences after nbm lesions (150). Rhesus monkeys have been used to assess primate basalocortical cholinergic function (114,115,151). Only the rabbit has been used to evaluate the relationship between two AD hallmarks, namely cholinergic impairment and amyloid deposits (113). Sheep have been used to evaluate the cholinergic mechanisms in offspring recognition (152). Lesions induced by immunotoxins in striatal and brain stem cells (also reported in these models (153)) are now being examined given their possible importance in studies of AD pathogeny or treatment.
4 Morphofunctional Neuronal and Glial Alterations and Cognitive Impairments in Basal Forebrain Lesion AD Models: Changes in Lesion Patterns over Time
The experimental manipulation of the basal forebrain provokes a wide variety of structural and functional changes in both the basal forebrain itself and the cortical areas innervated by its damaged neurons. These cortical lesions can be induced in different areas of the neocortex, paleocortex and/or archicortex. Different morphological and biochemical variables have been used to assess cholinergic and noncholinergic neuron impairment or death, as well as glial morphofunctional changes at both the basal and cortical anatomical levels. These changes provoke parallel cognitive impairments that can be assessed via behavioral tasks. In every cholinergic AD model, a specific pattern of basal lesion/dysfunction, cortical cholinergic and noncholinergic alterations and cognitive impairment is always observed for some time following the controlled induction of a lesion. From this moment, different involutive and/or adaptive processes or changes occur in the internal mechanisms of the directly or indirectly damaged cells (Figs. 2f, 6.1.3.2G and 3). The repercussions in the neuronal basal forebrain and cortical circuits, as well as in the glial cells of both regions, condition the short- and long-term structural and cognitive outcomes of these patterns. The intensity and duration of changes largely depend on factors related to the degree of basal forebrain damage, the lesion-inducing agent, and how the lesion was produced. On many occasions, the correlation between neuroanatomical alterations and cognitive impairment is very difficult to establish.
4.1 Factors Determining the Lesion Pattern Characterizing Cholinergic AD Models
The most important factors that determine the characteristics of each model are discussed below.
4.1.1 The Method and Protocol Used to Produce the Model
When a protocol is strictly followed to produce a forebrain lesion model, the results obtained by different authors show no great differences. However, those obtained with different (even slightly different) methods or protocols can differ widely. For example, microinjections of different excitotoxins into the same area of the basal forebrain apparently produce the same basal forebrain and cortical lesions yet can provide different models with different degrees of cholinergic dysfunction and associated behavioral impairment depending on the toxin used and its dose (Fig. 4). Ibotenic, quisqualic, or kainic acids are commonly employed to produce similar models, but the animals lesioned with each toxin show strong neuropathological and cognitive differences in in-depth analyses (6,22,105). Such differences are often attributed to the different damage sustained by noncholinergic neurons. When the same model is used but the results obtained differ to those previously published, the reason can lie in the use of different animal strains but also in how the models were actually produced. For example, microinjections using different types of apparatus, at different rates of infusion or pressure, and at different levels of neurotoxin diffusion and local reactivity produce different models. Further, the systems or methods used to evaluate variables (mainly in the performance of tasks) can have a great influence on the actual degree of change detected.
4.1.2 Species and Strain
Different species show specific characteristics with respect to their neural circuits and the cognitive roles played by their basal forebrain and cortical areas. Similar basal forebrain lesions in different species can produce different models with very distinct features. Some species are more sensitive to certain toxins, e.g., the rabbit is very sensitive to aluminum (26). On some occasions, different laboratories have obtained different results when using the same model because different subspecies or strains were used (37,38). The age of the animals is also important (see later). Age-dependent morphofunctional characteristics are also strain-dependent (40). For example, different strains of Wistar and Long Evans rats and of New Zealand white rabbit show different characteristics.
4.1.3 The Location and Extent of Basal Forebrain Lesion
The basal forebrain is a continuum of cholinergic centers with neurons running from the most anterior part of the septum to the most posterior area of the pallidal/subpallidal region. The greatest number of neurons is concentrated at particular levels (the classic cholinergic centers) that can be stereotactically damaged. A single injection may only cause a small lesion and therefore only a small amount of cholinergic damage (Fig. 3). To induce large lesions, two points of toxin injection are often required (99), mainly at the nbm/nbM level. However, a lesion induced in such a way that extensive diffusion of the toxin occurs could cause important cortical changes and behavioral impairments not related to the desired nbm/nbM damage; care should therefore be exercised. The neurons of neighboring structures, such as the striatopallidal and amygdalar complexes, are the main candidates for suffering these side effects. Cortical impairments are topographically related to the forebrain lesion induced; any cognitive impairment would be theoretically closely related to the higher brain functions associated with this area. More intense deficits in cognitive tasks might therefore be expected in all models involving wide basal-forebrain cholinergic damage, and more selective deficits in models with more restricted basal-forebrain lesions. Nonetheless, different relationships among the different directly and indirectly altered basalocortical and cortical circuits, as well as the adaptive neuronal changes that occur, could produce unexpected modifications in some behavioral performances. The behavioral results obtained with different models, including septum and/or nbm/nbM lesions, should be theoretically different, but they can sometimes show unexpected similarities (or even unexpected differences). Clear differences have been reported between general cholinergic forebrain damage and selective septum or nbm/nbM lesion models. However, a small number of studies report similar cognitive impairments after septum or nbm/nbM lesion (154). The reasons for this could lie in adaptive changes and/or in the very different kinds of tasks used to evaluate cognitive impairment. The i.c.v. infusion of cholinotoxins provokes a general cholinergic impairment of all forebrain cholinergic centers in such a way that involution of the neocortex and limbic structures occurs simultaneously. Many authors consider this kind of model to be closely related to AD, but the obtained results have shown very different impairments of the septohippocampal and nbm–cortical systems. A greater ability to adapt or recover from involutive changes in the septohippocampal system might be responsible for these differences.
4.1.4 Type and Concentration of the Neurotoxin Employed (6,16–18,22,98,99 102,105)
Each neurotoxin causes, directly and indirectly, specific neuronal and glial alterations. In general, the lesion pattern and its short-time development are dose-dependent and related to the toxin, but in the mid- or long-term, very different features can arise – generally by this time irrespective of the lesion-inducing agent (Fig. 4). Total recovery, partial recovery or nonrecovery of deficits have been reported, on some occasions with important discrepancies between cortical morphohistochemical features and behavior (6,18,22,25,87–89,96,97,100–102,127,155).
4.1.5 Unilateral or Bilateral Nature of the Lesion (16,22,102,156)
Surgical lesions of and stereotactic microinjections in the basal forebrain can produce models with unilateral or bilateral deficits. Unilateral models have the advantage that each animal has an internal control, i.e., the undamaged contralateral side. Microinjection of excitotoxins is the choice for producing different models with impairments on one or both sides of the brain. However, the manipulation of one side of the septum can lead to some degree of impairment on the contralateral side due to the contralateral diffusion of the toxic agent. Over the long term, some contralateral changes are induced in nbm/nbM lesions (6). Some toxins (e.g., ibotenic acid) are not very useful for producing bilateral nbm/nbM damage since they are associated with high mortality rates.
< div class='tao-gold-member'>
Only gold members can continue reading. Log In or Register a > to continue
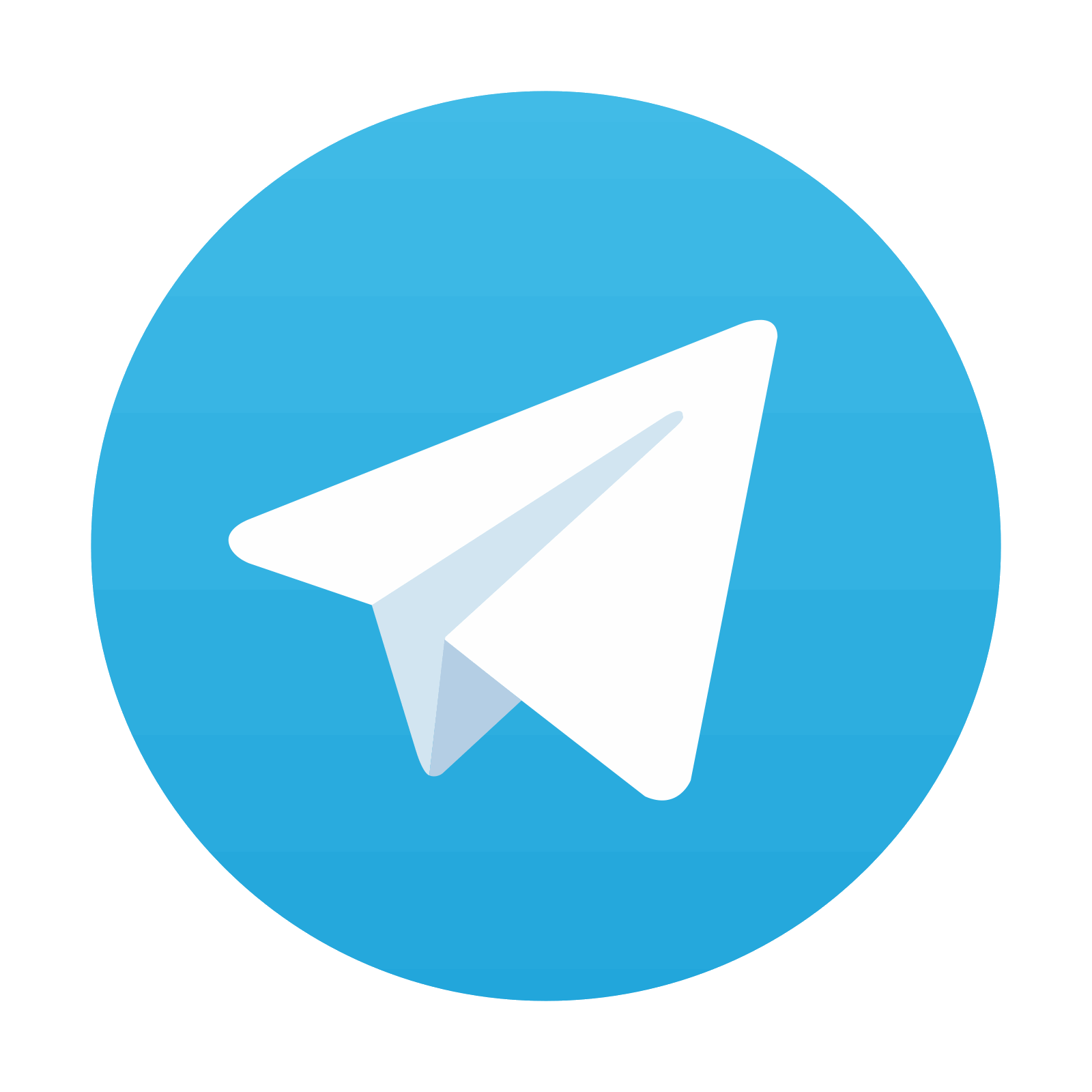
Stay updated, free articles. Join our Telegram channel
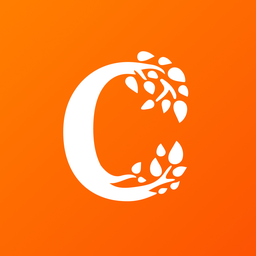
Full access? Get Clinical Tree
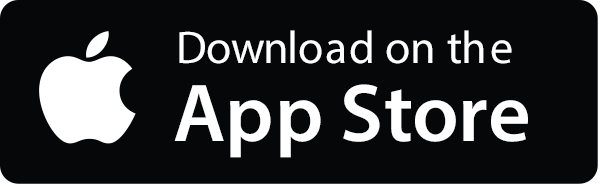
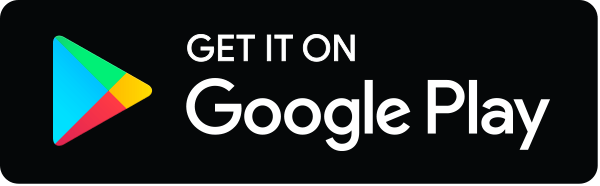