Chapter 7
General pharmacology of the inhalation anaesthetics
Minimum alveolar concentration (MAC)
Flammability and chemical stability
Biotransformation and organ toxicity
Actions on vital body functions
Comparison of volatile agents for veterinary clinical practice
Introduction
Since the first use of nitrous oxide (N2O) by Watt in 1844, and of ether by Morton in 1846, a large number of inhalation anaesthetic agents have been investigated. Many of those once used clinically have now been discarded but, surprisingly, the first two are still in use, at least in some parts of the world. Of those covered in earlier editions of this book, chloroform (first used in 1847) was discarded as it results in dose-dependent liver toxicity and also sensitizes the heart to epinephrine-induced arrhythmias. Cyclopropane was withdrawn because it is flammable and explosive when mixed with oxygen. Trichlorethylene, an excellent analgesic, reacted with the carbon dioxide absorbent and had to be given by a non-rebreathing system. The agents discussed individually below, to the best of the authors’ knowledge, all remain in clinical use (in humans and/or in animals) in some areas of the world.
The ideal inhalation anaesthetic should provide good quality anaesthesia, be practicable to give, non-flammable, stable chemically under all circumstances (in and out of the animal), allow a rapid induction and recovery from anaesthesia, have minimal effects on circulation and on the respiratory system, provide analgesia and muscle relaxation, be non-toxic and have no other unwanted side effects. No such agent exists, although xenon is coming close to meeting these targets.
The term ‘volatile anaesthetic agent’ can be applied to agents that are in liquid form at room temperature and 1 atmosphere of pressure and, therefore, require the use of a vaporizer. However, other agents are in gaseous form, and are presented in cylinders. The term ‘inhalation agent’ covers all. The mode of action of inhalation agents has been discussed in Chapter 1. Suffice it to say here that actions at any single receptor, ligand or voltage-gated channel cannot explain all the effects of inhalation anaesthetic agents.
Minimum alveolar concentration (MAC)
The concept of minimum alveolar concentration (MAC), how it is measured and factors which will effect it were discussed in Chapter 1. Variation in measurement of MAC have been reviewed by Shaughnessy & Hofmeister (2013). If a combination of inhalation anaesthetic agents is employed, their MACs are additive; this is the basis of regimens that use N2O (since N2O is not sufficiently potent to be used as a sole anaesthetic agent) together with a volatile agent such as isoflurane (Eger et al., 2003). It is now thought that an inhalation anaesthetic’s effect in preventing movement (and that is what defines MAC) is mediated at the spinal cord (Sonner et al., 2003; Antognini et al., 2005), while higher centres influence amnesia, hypnosis, and perception of pain. However, MAC remains the standard measure of ‘potency’ of an anaesthetic, is the measure by which equipotent levels of inhalation anaesthetics can be compared, and gives an indication of the concentration that, depending on other agents given, will be required in the clinical situation.
Analgesia
The mode by which inhalation agents produce, or do not produce, analgesia is not clear-cut. The two gaseous agents, N2O and xenon, produce marked analgesia, possibly through actions at the NMDA receptor. The volatile agents, ether and methoxyflurane, are not known to act at this receptor, yet have marked analgesic properties, while many other volatile agents do not.
Uptake and elimination
The pharmacokinetics of inhalation agents, the importance of the physical properties and the influence of physiological effects (e.g. cardiac output and ventilation) have been explained in Chapter 3. The important physical properties of agents in current use are given in Table 7.1. Of practical importance are:
•The lower the blood/gas solubility, the faster the induction of anaesthesia
•Induction of anaesthesia can be hastened by giving more than one MAC multiple, assuming that the saturated vapour pressure of the agent allows a sufficient concentration and that it can be given without causing hypoxia (Tables 7.1 and 7.2).
Table 7.1
Physical characteristics of some anaesthetic agents
NB: Atmospheric pressure may be reported in mmHg or millibar. 1 millibar = 1 hectopascal = 0.1 kPa
Order gives the lowest blood/gas solubility first. Solubilities depend on the temperature (most reported are at 37°C) and for oil/gas on oil used, hence variations in reported values. Blood/gas solubility values vary between species (Bergadano et al., 2003; Soares et al., 2012), partly, but not totally related to triglyceride value. Values compiled from Eger (1995), Jones (1990), Steffey (2002), Goto et al. (1998) and Jordan & Wright (2010).
Table 7.2
Some reported minimal alveolar concentration (MAC) values
Anaesthetic | Species | MAC (vol %) |
Nitrous oxide | Humans | 104 |
Xenon | Humans | 62–71 |
Dog | 119 | |
Halothane | Humans | 0.75 |
Dog | 0.86–0.95 | |
Cat | 0.82–1.22 | |
Horse | 0.88 | |
Goat | 1.3–1.4 | |
Isoflurane | Humans | 1.2 |
Dog | 1.28 | |
Cat | 1.2–2.22 | |
Horse | 1.31 | |
Goat | 1.5–1.62 | |
Sevoflurane | Humans | 2.1 |
Dog | 2.09–2.36 | |
Cat | 2.5–4.0 | |
Horse | 2.31–2.84 | |
Goat | 2.7 | |
Desflurane | Humans | 6 |
Dog | 7.2–10.32 | |
Cat | 10.27 | |
Horse | 8.06 | |
Goat | 10.52 |
MAC values depend on measurement methods and conditions (Quasha et al., 1980) hence range reported. MACs over 80% represent a theoretical projection.
Information compiled from the following reviews: Eger (1994), Clarke (1999), Lynch et al. (2000), Alibhai, (2001), Steffey (2002), Shaughnessy & Hofmeister, 2013 and original papers: Barter et al. (2004), Goto et al. (1998), Nickalls & Mapleson (2003), Steffey et al. (2005a,b).
Flammability and chemical stability
Modern anaesthetics must be non-flammable in the range of concentrations and gas mixtures (usually of O2 and N2O) used in clinical practice. Non-flammability is achieved by halogenation – in particular fluorination – of the agent. In most cases, this does not greatly change the flammability limits but it does increase the energy necessary to ignite the agents and it is this which renders these agents non-flammable in normal clinical use.
Although the anaesthetic agent itself may not be easily flammable, its breakdown products may be so. N2O increases the flammability of organic vapours because its exothermic decomposition results in production of an oxygen-rich mixture (33% O2). Breakdown of sevoflurane with the carbon dioxide absorbent, Barolyme, leads to a massive rise in temperature (see chemical stability below), resulting in melting of the anaesthetic circuit, fires and explosions (Fatheree & Leighton, 2004; Wu et al., 2004). Dunning et al. (2007), in in-vitro experiments, have demonstrated that sevoflurane breakdown with both Barolyme and soda lime can result in explosive concentrations of hydrogen, and this may have been a contributory cause of the fires that have occurred.
Anaesthetic agents need to be stable in storage and precautions required to ensure this will be discussed under the specific agents. The greatest concern is the potential for reaction of the agent with the highly alkaline compounds used for CO2 absorption (e.g. soda lime or Barolyme) in closed breathing systems. Until relatively recently, it was thought that the fluorinated hydrocarbons, other than sevoflurane, did not react with soda lime under the conditions likely to occur clinically, but this is now known not to be true.
Sevoflurane, when used in closed systems, produces a number of breakdown products of which ‘Compound A’ (CF3=C(CF3)-O-CH2F) is nephrotoxic in rats. Although toxic values (in rats) usually need to exceed 1000 ppm, under certain circumstances, levels as low as 50 ppm may cause medullary tubular necrosis (Keller et al., 1995). The concentrations of Compound A which occur are greatest at higher temperatures, with dry absorbents, with low flow or closed systems and, not surprisingly, with high concentrations of sevoflurane (Baum & Aitkenhead, 1995). Levels in humans and the dog using closed systems are usually about 20 ppm but, in certain circumstances, reach over 50 ppm in individuals (Smith et al., 1996). Nevertheless, there is no evidence that renal failure has resulted from sevoflurane anaesthesia in humans (Sahin et al., 2011) or during veterinary use in domestic animals.
The passage of some inhalation anaesthetic agents over very dry highly basic CO2 absorbents results in accumulation of carbon monoxide (CO) and formaldehyde within a closed anaesthetic system. Of the inhalation agents in common use, desflurane produces the greatest amount of CO, and halothane probably the least (Fang et al., 1995). It was thought that breakdown of sevoflurane would not release CO until the cases mentioned above. In both those cases, the absorbent was Barolyme, which has since been withdrawn. In Fatheree & Leighton’s case (2004), a low flow (not closed) system was being used. At the end of surgery, they found that the absorbent canister was very hot, and on disconnection, saw white smoke and smelt burning plastic (on later examination there was fused plastic, and burnt rubber). The patient had arterial blood carboxyhaemoglobin of 29%, oxygen saturation of only 69%, developed acute respiratory distress syndrome, but recovered with intensive care. During anaesthesia, the only point of note had been that inspired sevoflurane was low despite a vaporizer setting of 8%. An unexpectedly low delivered sevoflurane concentration appears to be a warning sign of breakdown of the agent. In the report of Wu et al. (2004), there was an explosion and fire in a machine which had been left with oxygen running and the vaporizer turned on between patients.
The problems relating to agent breakdown occur mainly with dry soda lime, and can be reduced by turning off the O2 flow of ‘fail-safe’ machines when they are not in use to avoid the drying effect of continuous gas flow through the system. However, the best avoidance is to use one of the newer absorbents; those without the KOH are less likely to result in breakdown of any inhalation agents; the absorbents which do not contain any of the strong bases (see Chapter 10) reduce agent breakdown even further and are best used for sevoflurane.
Biotransformation and organ toxicity
Damage to organs as a result of inhalation anaesthetic agents may be due to direct toxic effects of the agent, to effects mediated by metabolites, or to hypoxic changes, usually from poor organ blood flow. Except in sensitivity reactions, the toxicity of the unchanged compound is often, but not always, directly related to the concentration present and decreasing the concentration and/or the duration of exposure will decrease toxicity.
Toxic effects of anaesthetic drugs are most commonly seen in the liver and kidneys. Kharasch (2008) has recently reviewed the current understanding of the mechanisms. The most commonly cited example is that of halothane. A small amount of halothane (up to 3%) is metabolized by anaerobic reduction, catalysed by CYP2A6, that results in a small increase in transaminase, which is clinically unimportant. However, large amounts (25% plus) undergo oxidation catalysed predominantly by CYP2E1. Breakdown products include trifluoroacetyl halides which can link to liver proteins. In some cases, antibodies are formed against the halothane-induced antigen, resulting in immune-mediated liver damage (so-called ‘halothane hepatitis’). However, isoflurane and enflurane produce similar breakdown products to halothane to a lesser extent and, although very rare, similar autoimmune-mediated hepatitis may occur (Frink, 1995; Kenna & Jones, 1995).
The renal damage which was reported following the prolonged use of methoxyflurane was originally thought to be due to free fluoride ions formed from hepatic metabolism. This theory is now known to be incorrect. In most species, fluoride alone is nephrotoxic only at very high concentrations. Kharasch (2008) explains that methoxyflurane is O-demethylated to fluoride and to dichloracetic acid (DCAA). DCAA alone is not nephrotoxic, but appears to increase the toxicity of fluoride. Of the fluorinated inhalation agents, only methoxyflurane breaks down both to fluoride and DCAA. The incorrect interpretation of the mechanisms of methoxyflurane nephrotoxicity delayed the introduction of sevoflurane (metabolism of which results in fluoride) for many years.
Actions on vital body functions
In experiments on isolated organs, almost all anaesthetics have a dose-dependent depressant effect on the cardiovascular system but, in an intact animal, these effects may be modified or even controlled by mechanisms such as the effect of increased CO2 or the response to surgery causing sympathetic stimulation. There are major differences between the cardiovascular actions of the agents in spontaneously breathing patients compared to those undergoing intermittent positive pressure ventilation (IPPV), when CO2 can be controlled. Of practical clinical importance for comparison of such effects is, for the cardiovascular system, the effect of equipotent dose effects on heart rate and rhythm (including sensitivity to epinephrine), on myocardial contractility, cardiac output, and on the resistance of peripheral, pulmonary, cerebral and other organ vasculature. Of particular importance is the effect on overall blood flow (rather than just blood pressure). The effect of different anaesthetic agents on overall blood flow to specific organs, in particular brain, kidneys and heart, has been the subject of a great deal of research in human anaesthesia, in order to relate use of a specific agent to a specific type of surgery, or patient disease. For example, isoflurane causes more ‘coronary steal’ than enflurane (Diana et al., 1993); there are many investigations as to the relative effects of sevoflurane and desflurane on this phenomenon. With the exception of N2O, all the inhalation anaesthetics produce a concentration-dependent depression of respiration. Although there is some difference of degree between the agents currently used, the impact on respiration is considered of less importance than cardiovascular effects as IPPV can always be employed. Some anaesthetics are irritant to the airways that may lead to breath holding when they are used for anaesthetic induction or in very lightly anaesthetized animals. Good monitoring (see Chapter 2) enables many of these depressant effects to be detected and treated.
All the halogenated volatile anaesthetic agents, (but not the gaseous agents N2O and xenon) can trigger the condition of malignant hyperthermia. There recently have been concerns that inhalant anaesthesia may lead to prolonged cognitive dysfunction through a direct neurotoxic action (reviewed by Mandal et al., 2009).
Not all effects of inhalation agents are negative. Despite the concern over cognitive dysfunction mentioned above, most anaesthetic agents are thought to have neuroprotective properties (Matchett et al., 2009; Schifilliti et al., 2010) against hypoxic insult, as do many intravenous agents. Inhalation agents also protect the heart against hypoxic insults; most experimental evidence in animal models suggests that this protection is greater than that of intravenous agents and that this is so has been confirmed in clinical practice (De Hert et al., 2005; Landoni et al., 2007; Bein, 2011; Van Rompaey & Barvais, 2011).
Interaction with other drugs
Any drug that has CNS depressant effects, whether sedative, hypnotic or analgesic will add, or in some cases be synergistic with, the effect of inhaled anaesthetic agents. Many such drug combinations are used to reduce the dose of inhalation anaesthetic agent used, and will be described in the relevant species chapters. The basis of use is that if less inhalation agent is used, there will be less cardiovascular depression. There is little or no evidence as yet that this is always the case; blood pressure may be higher but cardiac output, and therefore blood flow, may not be improved over that which would have occurred with the inhalation agent alone. The exception is the use of some of the analgesics together with inhalation agents which themselves give poor analgesia. Local nerve blocks where practicable are particularly effective. Other techniques include infusion of opioids, lidocaine, α2-agonists or ketamine (see Chapter 5). There are species differences between the most suitable methods.
Agents which stimulate the CNS will tend to reverse the effects of anaesthetic drugs. In veterinary patients for anaesthesia, interactions with accidental ingested of recreational drugs (‘uppers or downers’) may have to be considered on rare occasions.
All neuromuscular blocking drugs are potentiated by volatile anaesthetics in a dose-dependent manner (see Chapter 8), but not by the inhalation agent, xenon. Interest has been greatest in relation to anaesthesia of patients with myasthenia gravis (Blichfeldt-Lauridsen & Hansen, 2012). Sevoflurane has been reported to reduce the TOF (train of four) ratio in a dose-dependent manner in both normal and myasthenic patients (Nitahara et al., 2007).
The sensitization of the myocardium to both endogenous and exogenous epinephrine by the inhalational agents has been the subject of much investigation. Straight-chain hydrocarbons (such as halothane) tend to sensitize the heart to catecholamines; the ethers, especially if fluorinated, do not have this effect.
Occupational exposure to inhalation anaesthetic agents
Personnel exposed to trace concentrations of inhalation anaesthetics in the atmosphere inhale and retain these agents in their bodies for some hours or even days, depending on the solubility of the agents concerned. As a result of concerns raised in the 1970s that such exposure might be deleterious to health, legal requirements setting ‘limits’ to such exposure have been introduced in most countries. In the UK, they are governed by ‘The Control of Substance Hazardous to Human Health (COSHH) regulations’ and the Occupational Exposure Standards (OES) set is based on an eight hour time weighted average of 100 ppm of N2O, 10 ppm of halothane and 50 ppm of isoflurane. The limits have not been updated to include sevoflurane and desflurane. In the USA, levels of exposure which should not be exceeded are, for example, by government recommendation 2.0 ppm for volatile agents and 25 ppm for N2O. Other countries differ in their requirements (ISSA, 1996). The limits of exposure chosen by various authorities differ between countries have no good evidence base (ISSA, 1996). The most serious concern was that of effects on fertility and on developmental defects. ISSA (1996) summarizes a meta-analysis of the evidence available in 1996; there is evidence in experimental models that implicates nitrous oxide but there is little compelling evidence of long-lasting harm relating to the other agents. However, there is evidence of concurrent reduction in cognitive function for personnel working in an atmosphere contaminated by inhalation anaesthetics. The result of the concerns led to the introduction of waste gas scavenging, and regular monitoring of its efficacy (see Chapter 10), and the authors are in no doubt that this has improved the working environment in operating theatres.
Individual inhalation anaesthetics
Nitrous oxide (N2O)
N2O is a gaseous anaesthetic agent. Although the first original anaesthetic agent, it is still in use in medical and veterinary anaesthesia, in particular because of its outstanding analgesic effects, which are evident at subanaesthetic concentrations. Its mode of action is thought primarily to be action inhibiting the NMDA receptor (Jevtovic-Todorovic et al., 1998; Mennerick et al., 1998), which may explain its analgesic potency (see Chapter 5). As such, the use of BIS monitors are ineffective at monitoring the depth of anaesthesia with this agent, as are auditory evoked potentials (see Chapter 1) (Yagi et al., 1995).
N2O is a colourless gas with a faint, rather pleasant smell; it is not flammable or explosive but it will support combustion, even in the absence of free O2. Compressed into cylinders (tanks) at 40 atmospheres pressure it liquefies so is presented in cylinders (see Chapter 10). N2O is also available in a 50% mixture with 50% oxygen, as Entanox® or Nitronox.
The MAC of N2O is over 100% so it cannot be used alone to produce anaesthesia and must be administered with sufficient O2 (>25%) to prevent hypoxia. However, the rapid onset and recovery from its effects, coupled with its strong analgesic properties make it a useful adjuvant to an anaesthetic protocol. The low solubility of N2O results in rapid equilibration between inspired and expired concentrations. As a result, when used with oxygen in a low-flow rebreathing circuit, concentrations of N2O tend to increase as, while after equilibration there is no further uptake of N2O, oxygen is still being utilized. To prevent a hypoxic mixture, higher flows or inspired oxygen monitoring are advisable.
N2O moves rapidly into gas-filled spaces in the body at a greater rate than nitrogen can diffuse out. This is of considerable importance in herbivores and in the presence of a closed pneumothorax. The low solubility also results in the phenomena of ‘the second gas effect’ at induction of anaesthesia, and potentially in ‘diffusion hypoxia’ at recovery. When N2O is first administered to a patient, a large gradient exists between the tension in the inspired gas and the arterial blood so that initially the blood takes up large volumes of gas. Its rapid removal from the alveoli by the blood elevates the tension of any remaining (second) gas or vapour such as oxygen, or a volatile anaesthetic agent, and augments alveolar ventilation. Thus, during the first few minutes of N2O administration, anaesthetic uptake is facilitated because the enhanced tension of the second gas ensures a steeper tension gradient for its passage into the blood. This is known as ‘the second gas effect’. There is also a reverse effect speeding elimination of the accompanying volatile agent at recovery, speeding arousal (Peyton et al, 2011). The phenomenon known as ‘diffusion hypoxia’ occurs immediately following anaesthesia when the gas is being rapidly eliminated from the lungs; N2O may form 10% or more of the volume of expired gas, and the outward diffusion of N2O into the alveoli lowers the partial pressure of O2 in the lungs, and therefore in arterial blood. Thus, 5–10 minutes of O2 inhalation when N2O is discontinued at the end of a lengthy procedure is advisable even in healthy animals, and essential in animals suffering from any pulmonary inadequacy.
N2O causes minimal cardiovascular effects. It is non-irritant to inhale, and it causes increased pulmonary ventilation (Hall, 1988). Only 0.004% of N2O is metabolized and it has no direct toxic effects on liver and kidneys. However, exposure to low levels over several days causes bone marrow depression in humans due to interference with methionine synthase giving rise to disturbances of folate metabolism (Hathout & El-Saden, 2011) and megablastosis (ISSA, 1996). It is embryotoxic in experimental animals. It is also a major ‘greenhouse’ gas. The use of N2O within anaesthesia is now declining in popularity, but it still has a major role as an analgesic.
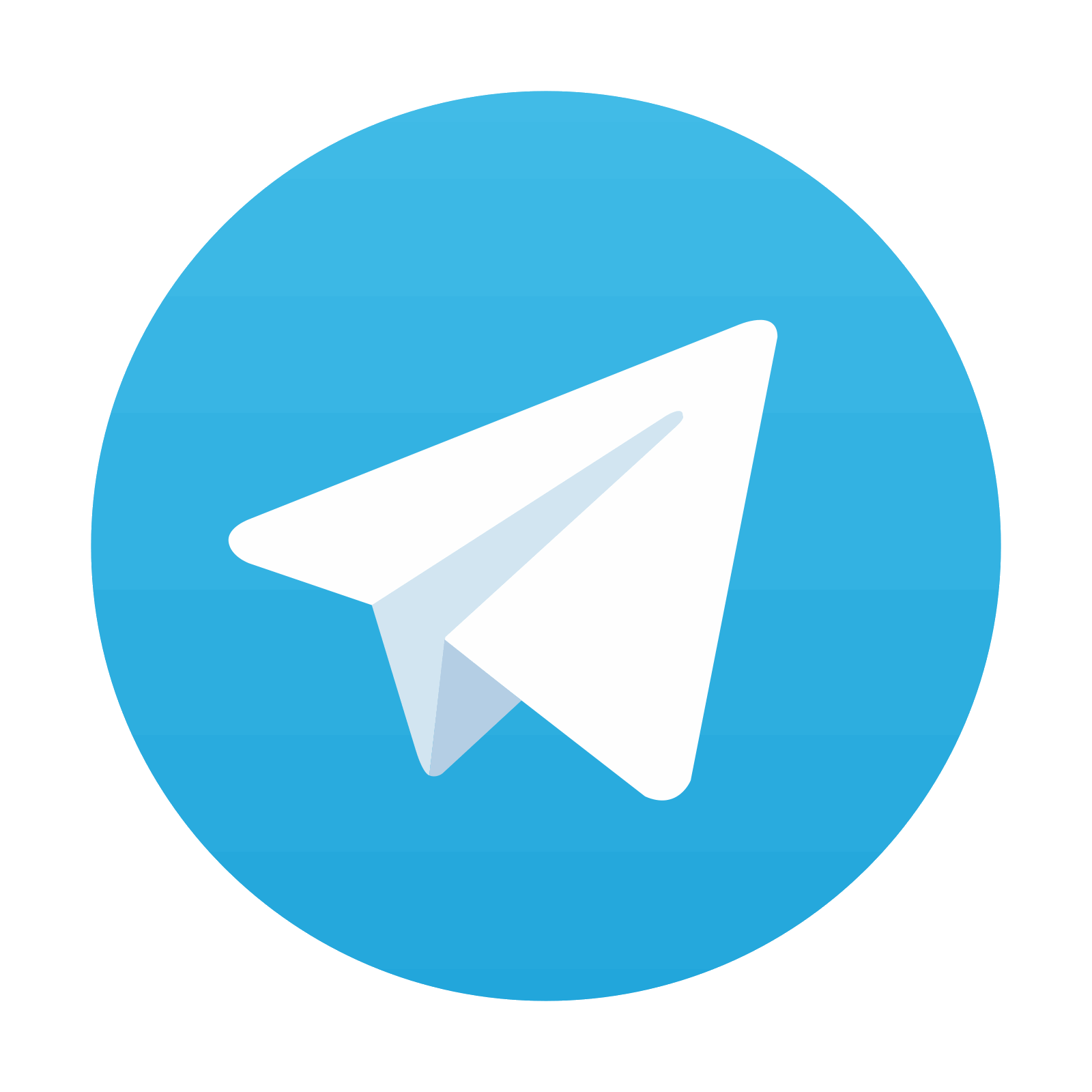
Stay updated, free articles. Join our Telegram channel
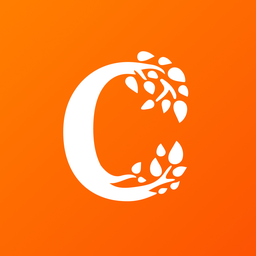
Full access? Get Clinical Tree
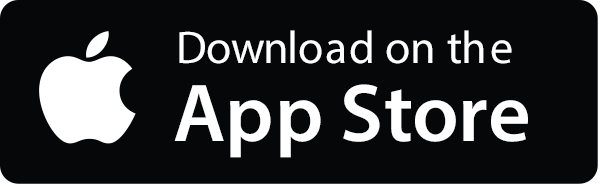
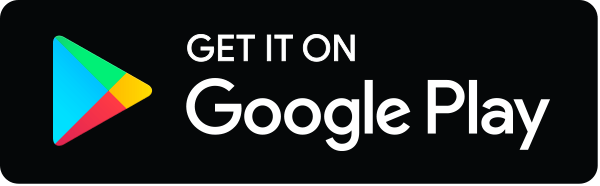