1. A small amount of oxygen is transported in solution in plasma, but most is in combination with hemoglobin. 2. A molecule of hemoglobin can reversibly combine with four molecules of oxygen. 3. The binding of oxygen and hemoglobin is determined by oxygen tension. 4. The oxyhemoglobin dissociation curve can be displayed with percent saturation of hemoglobin as a function of oxygen tension. 5. The affinity of hemoglobin for oxygen varies with blood temperature, pH, carbon dioxide tension, and the intracellular concentration of certain organic phosphates. 6. As hemoglobin is depleted of oxygen, its color changes from bright red to bluish red. 7. Carbon monoxide has 200 times the affinity of oxygen for hemoglobin. 8. Methemoglobinemia occurs in certain toxic states, notably nitrite poisoning. 1. Carbon dioxide is transported in the blood both in solution in plasma and in chemical combination. 1. Oxygen demands of exercise are met by increases in blood flow, in hemoglobin levels, and in oxygen extraction from blood. Even though the amount of oxygen dissolved in plasma is small, it increases directly as the partial pressure of oxygen increases; 0.003 mL of oxygen dissolves in each 100 mL (1 dL) of plasma at an oxygen tension (PO2) of 1 mm Hg (Figure 48-1). The pulmonary capillary blood equilibrates with the alveolar oxygen tension (PAO2) of 100 mm Hg; therefore, 0.3 mL of oxygen dissolves in each deciliter of blood. If an animal breathes pure oxygen its PAO2 increases to at least 600 mm Hg and 1.8 mL of oxygen (600 × 0.003) dissolves in each deciliter of plasma. Mammalian hemoglobin consists of four units, each containing one heme and its associated protein (globin). Globin is a polypeptide composed of 140 to 150 amino acids. Heme is a protoporphyrin consisting of four pyrroles with a ferrous iron at the center. Each ferrous iron can combine reversibly with a single molecule of oxygen. The complete hemoglobin molecule has four hemes each with its associated globin and thus can combine reversibly with up to four molecules of oxygen (Figure 48-2). The type and sequence of amino acids that compose globin are critical to oxygen binding. Without the presence of globin, oxygen would irreversibly oxidize the ferrous iron to ferric iron. The amino acids in globin cradle the heme and limit access of oxygen to the ferrous iron. This prevents oxidation and allows uptake and release of oxygen in response to local PO2. The type and sequence of amino acids that compose globin define the different types of mammalian hemoglobin. Adult hemoglobin contains two alpha (α) and two beta (β) amino acid chains; fetal hemoglobin contains two α and two gamma (γ) chains. Closely related species, such as humans and anthropoid apes, have similar amino acid sequences on the side chains, whereas more divergent species have more differences in amino acid sequences. Each hemoglobin molecule can reversibly bind up to four molecules of oxygen, one with each heme. The reversible combination of oxygen with hemoglobin is shown in the oxyhemoglobin dissociation curve (Figure 48-3). The binding of oxygen is a four-step process, and the oxygen affinity of a particular heme is influenced by the oxygenation of the others. This means that when the first heme unit is oxygenated, oxygen affinity of the second heme unit is increased, and so on. These heme-heme interactions are responsible for the sigmoid shape of the oxyhemoglobin dissociation curve. Figure 48-3 shows that the oxygen content of blood—that is, the amount of oxygen combined with hemoglobin—is determined by PO2. At a PO2 of more than approximately 70 mm Hg, the oxyhemoglobin dissociation curve is virtually flat, which indicates that further increases in PO2 add little oxygen to hemoglobin. At this point, the hemoglobin is saturated with oxygen because each iron atom is associated with an oxygen molecule. The fact that hemoglobin becomes virtually saturated with oxygen at a PO2 of more than 70 mm Hg has important clinical consequences. Many animals live at altitudes considerably above sea level, where the lower barometric pressure results in a low PIO2 (inspired oxygen tension). Although these animals have a lower PaO2 (arterial oxygen tension) than their sea-level–dwelling counterparts, they are still able to transport sufficient oxygen to their tissues because their hemoglobin is well saturated with oxygen. Clearly, at extreme altitudes, hemoglobin begins to desaturate. Percent saturation of hemoglobin is the ratio of oxygen content to oxygen capacity. Hemoglobin is more than 95% saturated with oxygen when it leaves the lungs of an animal at sea level. Percent saturation of mixed venous blood averages 75%; venous oxygen tension ( Changes in carbon dioxide tension (PCO2) and pH also affect the affinity of hemoglobin for oxygen. The shift in the oxyhemoglobin dissociation curve resulting from a change in PCO2 is called the Bohr shift. This shift results in part from the combination of carbon dioxide with hemoglobin, but mostly from the production of hydrogen ions, which decrease the pH. A change in pH alters the oxygen binding by changing the structure of hemoglobin. As a result, a higher, more alkaline pH shifts the oxyhemoglobin dissociation curve to the left, and a lower, more acidic pH shifts the curve to the right (Figure 48-5). The Bohr effect is not constant among species; a given change in pH produces a greater shift in the dissociation curve for small mammals than for large mammals, supposedly ensuring the delivery of oxygen during high rates of metabolic activity, when carbon dioxide production is greatest.
Gas Transport in the Blood
Oxygen Transport
A Small Amount of Oxygen Is Transported in Solution in Plasma, but Most Is in Combination with Hemoglobin
A Molecule of Hemoglobin Can Reversibly Combine with Four Molecules of Oxygen
The Binding of Oxygen and Hemoglobin Is Determined by Oxygen Tension
The Oxyhemoglobin Dissociation Curve Can Be Displayed with Percent Saturation of Hemoglobin as a Function of Oxygen Tension
) averages 40 mm Hg. Although all mammals have similarly shaped oxyhemoglobin dissociation curves, the position of the curve with regard to PO2 varies (Figure 48-4). This can be described by measurement of P50, the partial pressure at which hemoglobin is 50% saturated with oxygen. A higher P50 is generally found in small mammals and allows unloading of oxygen at a high PO2 to satisfy their higher metabolic demands.
The Affinity of Hemoglobin for Oxygen Varies with Blood Temperature, pH, Carbon Dioxide Tension, and the Intracellular Concentration of Certain Organic Phosphates
Stay updated, free articles. Join our Telegram channel
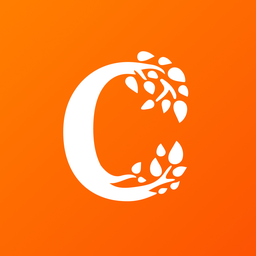
Full access? Get Clinical Tree
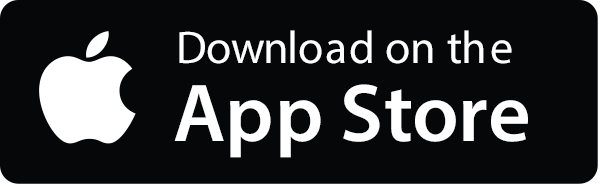
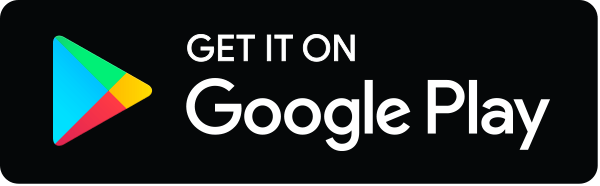