1. Digestion and absorption are separate, but related, processes. 2. The small-intestinal mucosa has a large surface area and epithelial cells with “leaky” junctions between them. 3. The intestinal surface microenvironment consists of glycocalyx, mucus, and an unstirred water layer. 1. Breaking down food particle size by physical action is an important part of the digestive process. 2. Chemical digestion results in the reduction of complex nutrients into simpler molecules. 3. Luminal-phase carbohydrate digestion results in the production of short-chain polysaccharides. 4. Luminal-phase digestion of carbohydrates applies only to starches, because sugars are digested in the membranous phase. 5. Proteins are digested by a variety of luminal-phase enzymes. 6. Membranous-phase digestive enzymes are a structural part of the intestinal surface membrane. 7. Membranous-phase digestion occurs within the microenvironment of the unstirred water layer, intestinal mucus, and glycocalyx. 8. A specific membranous-phase enzyme exists for the digestion of each type of polysaccharide. 9. Complete digestion of peptides to free amino acids takes place both on the enterocyte surface and within the cells. 1. Specialized nutrient transport systems exist in the apical and basolateral membranes. 2. Secondary and tertiary active transport mechanisms utilize the transcellular sodium ion electrochemical gradient as their source of energy. 3. Passive transport occurs either through specialized channels in cell membranes or directly through the tight junctions. 4. The products of membranous-phase digestion are absorbed by sodium co-transport. Absorption of water and electrolytes 1. There are at least three distinct mechanisms of sodium absorption. 2. There are three major mechanisms of chloride absorption. 3. Bicarbonate ion is secreted by several digestive glands and must be recovered from the gut if body acid-base balance is to be maintained. 4. Potassium is absorbed primarily by passive diffusion through the paracellular route. 5. The major mechanisms of electrolyte absorption are selectively distributed along the gut. 6. All intestinal water absorption is passive, occurring because of the absorption of osmotically active solutes. Intestinal secretion of water and electrolytes 1. Passive increases in luminal osmotic pressure occur during hydrolytic digestion and result in water secretion. 2. Active secretion of electrolytes from the crypt epithelium leads to intestinal water secretion. 1. Water and solute movement between the lateral spaces and villous capillaries is subject to the same forces that govern water and solute movement between the extracellular and vascular fluids in other tissues. 2. Absorbed nutrients enter the capillaries by diffusion from the lateral spaces. 3. A countercurrent, osmotic-multiplier system may increase the osmolality of blood at the tips of the villi, further promoting absorption of water into the blood. 4. Disturbances in the venous drainage from the intestine can greatly affect the mechanisms of capillary absorption in the villi. Digestion and absorption of fats 1. Detergent action as well as enzymatic action is necessary for the digestion and absorption of lipids. 2. Lipids are absorbed through the apical membrane by carrier proteins and simple diffusion. 3. Bile acids are reabsorbed from the ileum by a sodium co-transport system. 4. Absorbed lipids are packaged into chylomicrons before leaving the enterocytes. Growth and development of the intestinal epithelium 1. The length of intestinal villi is determined by the relative rates of cell loss at the tips and cell replenishment at the base. 1. During the first few hours of life, proteins are not digested but are absorbed intact. 2. The major intestinal disaccharidase switches from lactase to maltase with maturity. 1. Diarrhea occurs when there is a mismatch between secretion and absorption. Digestion is the process of breaking down complex nutrients into simple molecules. In contrast, absorption is the process of transporting those simple molecules across the intestinal epithelium (Figure 30-1). The two processes are the result of different biochemical events occurring within the gut. Both processes are necessary for the assimilation of nutrients into the body; absorption cannot occur if food is not digested, and the process of digestion is fruitless if the digested nutrients cannot be absorbed. Contact between the small-intestinal mucosa and the luminal contents is facilitated by an extensive intestinal surface area. Three levels of surface convolutions serve to expand the surface area of the small intestine (see Figure 27-2). First, large folds of mucosa known as plicae circulares add to the intestinal surface area of some animals, but these are not present in all species. Second, the mucosal surface is covered with fingerlike epithelial projections known as villi. These structures are present in all species and increase the intestinal surface area by tenfold to fourteenfold compared with a flat surface of equal size. Third, the villi themselves are covered with a brushlike surface membrane known as the brush border. The brush border is composed of submicroscopic microvilli that further enlarge the surface area (Figure 30-2). At the base of the villi are glandlike structures known as crypts of Lieberkühn (Figure 30-3). The villi and crypts are covered with a continuous layer of cellular epithelium. The epithelial cells covering the villi and crypts are called enterocytes. Each enterocyte has two distinct types of cell membranes (Figure 30-4). The cell surface facing the lumen is called the apex and is covered by the apical membrane. The apical membrane contains the microvilli. Under the light microscope, the microvilli give the cell surface its brushlike appearance. This appearance has led to the term brush border, which is synonymous with apical membrane. Attached to the apical membrane are many glycoproteins. These specialized proteins are synthesized within the enterocytes and transferred to the apical membrane. They are the enzymes and transport molecules responsible for the digestive and absorptive functions of the intestinal epithelium. Under the intense magnification of the electron microscope these proteins, which extend into the intestinal lumen, give the microvilli a fuzzy appearance (see Figure 30-2). This rich area of glycoproteins on the surface of the apical membranes is given the name glycocalyx. The apical membrane is a complex cellular membrane with an unusually high protein content. The overall process of digestion is the physical and chemical breaking down of food particles and molecules into subunits suitable for absorption. Physical reduction of food particle size is important not only because it allows food to flow through the relatively narrow digestive tube, but also because it enlarges the surface area of the food particles, thus increasing the area exposed to the actions of digestive enzymes. Physical reduction of food particle size begins with mastication (chewing) but is completed by the grinding action of the distal stomach. In the distal stomach the physical action of grinding is aided by the chemical actions of pepsin and hydrochloric acid. The chemical actions of these stomach secretions break down connective tissue and thus aid in breaking apart food particles, especially foods of animal origin. The reduction of food particle size by physical means is essentially complete when food leaves the stomach, as described in Chapter 28 in the discussion of motility of the distal stomach. Chemical digestion of each major nutrient is accomplished by the process of hydrolysis, the splitting of a chemical bond by the insertion of a water molecule. Glycosidic linkages in carbohydrates, peptide bonds in proteins, ester bonds in fats, and phosphodiester bonds in nucleic acids are all cleaved by hydrolysis during digestion. Figure 30-5 illustrates hydrolytic splitting of these various chemical bonds. Hydrolysis in the digestive tract is catalyzed by the action of enzymes. There are two general classes of digestive enzymes: those that act within the lumen of the gut, and those that act at the membrane surface of the epithelium. Enzymes acting within the lumen originate from the major GI glands, including the salivary gland, gastric glands, and especially the pancreas. The secretions of these glands become thoroughly mixed with ingesta and exert their actions throughout the lumen of their associated gut segments; thus the actions they catalyze are referred to as the luminal phase of digestion. In general, luminal-phase digestion results in the incomplete hydrolysis of nutrients, resulting in the formation of short-chain polymers from the original macromolecules (Figure 30-6). Carbohydrates are nutrients containing carbon, hydrogen, and oxygen atoms arranged as long chains of repeating simple-sugar molecules. Dietary carbohydrates originate primarily from plants. There are three general types of plant carbohydrates: fibers, sugars, and starches. Fibers, the structural parts of plants, form an important energy source for herbivorous animals; however, plant fibers are not subject to hydrolytic digestion by mammalian enzymes and therefore cannot be digested directly by animals (see Chapter 31). Sugars are energy-transport molecules in plants. Sugars, or saccharides, may be simple (made up of a single molecular unit, monosaccharides) or complex (made up of two or more repeating saccharide subunits, polysaccharides). Glucose, galactose, and fructose are the most important simple sugars in animal diets. These monosaccharides are present, preformed in small quantities, in normal diets; however, most monosaccharides absorbed from the gut arise from the enzymatic hydrolysis of more complex carbohydrates. Complex sugars are referred to as disaccharides, trisaccharides, and oligosaccharides, depending on the number of repeating simple-sugar subunits. Oligosaccharides contain several monomer units, usually between 3 and 10. Important complex sugars in animal diets are lactose, or milk sugar, and sucrose, or table sugar. Lactose is a disaccharide composed of glucose and galactose, whereas sucrose is a disaccharide composed of glucose and fructose. Other important complex sugars are maltose, isomaltose, and maltotriose; these three sugars are composed of two or three repeating glucose units (Figure 30-7). They are seldom present preformed in the diet but rather are formed in the gut as intermediate products of starch digestion. Starch is an energy-storage carbohydrate of plants that forms the major energy-yielding nutrient in the diets of many omnivorous animals, such as pigs, rats, and primates. There are two chemical forms of starch, amylose and amylopectin. Both are long-chain glucose polymers, but amylose is a straight-chain molecule containing glucose monomers linked by α[1-4] glycosidic linkages. Amylopectin also contains glucose chains joined by α[1-4] glycosidic linkages, but the amylopectin chains are branched, having an α[1-6] linkage at each branch point (see Figure 30-7). Although the chemical structure of starch is limited to these two molecular types, the physical structure and encapsulation of starches vary among plant sources. This variation results in the unique characteristics of starches from different sources, such as wheat, corn, and barley. The enzyme involved in luminal starch digestion is α-amylase, which is actually a mixture of several similar molecules. This enzyme arises from the pancreas in all species, as well as from the salivary glands of some species (see Chapter 29). The α[1-4] linkages of either amylose or amylopectin are attacked by α-amylase. Characteristic of luminal-phase digestion, α-amylase does not break off, or cleave, single glucose units from the ends of the chain. Rather, the starch chains are broken in their midsections, resulting in the production of polysaccharides of intermediate chain length, known as dextrins. These chains continue to be attacked until disaccharide (maltose) and trisaccharide (maltotriose) units are formed. This digestive process proceeds for amylopectin in the same way as it does for amylose, except that the α[1-6] linkages at the chain branch points of amylopectin are not hydrolyzed. Because these branch points are not hydrolyzed, branch-chain oligosaccharides, known as limit dextrins, as well as an α[1-6]-linked disaccharide, known as isomaltose, are formed (see Figure 30-7). The end result of luminal-phase carbohydrate digestion is the creation of many disaccharides, trisaccharides, and oligosaccharides from large starch molecules. These complex sugars are not hydrolyzed further in the luminal phase. The major luminal-phase proteolytic enzymes are listed in Table 30-1. Most proteolytic enzymes are endopeptidases, meaning that they break proteins at internal points along the amino acid chains, resulting in the production of short-chain peptides from complex proteins. Endopeptidases produce essentially no free amino acids. Two exopeptidases, which release individual amino acids from ends of peptide chains, are secreted also from the pancreas and are active in luminal-phase digestion. TABLE 30-1 Luminal-Phase Enzymes of Protein Digestion The proteolytic enzymes are secreted from the stomach glands or pancreas in the form of inactive zymogens (see Chapter 29), which are activated in the stomach or intestinal lumen, respectively. These enzymes must be secreted in an inactive form; otherwise, the active enzymes would digest the cells in which they are synthesized. Activation of the zymogens occurs in the gut lumen. The proteolytic zymogens of the stomach, pepsinogen and chymosinogen, are activated by hydrochloric acid (HCl) in the stomach lumen. Pepsinogen is also activated by pepsin in an autocatalytic feedback loop. Trypsinogen from the pancreas is activated by enterokinase, an enzyme elaborated by duodenal mucosal cells. The active enzyme, trypsin, then serves as an autocatalytic agent to activate additional trypsinogen as well as the other pancreatic protein-digesting enzymes. Figure 30-8 illustrates the cascade of intraluminal zymogen activation. As previously described, the unstirred water layer, mucus, and glycocalyx form a diffuse zone separating the mucosal surface from the lumen of the intestine. The membranous-phase digestive enzymes project from the apical membrane into this surface layer. The quiet surface layer forms a microenvironment in which membranous-phase digestion occurs. Peptides and polysaccharides in the intestinal lumen must diffuse into the surface layer before membranous-phase digestion can take place. Furthermore, most of the products of membranous-phase digestion never diffuse away from the surface environment back into the lumen of the intestine; instead, they are absorbed, soon after formation, into the underlying epithelial cells. This arrangement is efficient because it ensures that the final products of carbohydrate and protein digestion are formed near their site of absorption, avoiding the need for long diffusion distances (Figure 30-9). The membranous-phase enzymes of carbohydrate digestion have as their substrates dietary complex carbohydrates, such as sucrose and lactose, as well as the polysaccharide products of luminal-phase starch digestion, including maltose and isomaltose. The specific membranous-phase enzymes are named according to their substrates and include maltase, isomaltase, sucrase, and lactase. The sole product of maltose and isomaltose digestion is glucose, whereas in addition to glucose, fructose and galactose are produced from the digestion of sucrose and lactose, respectively. All polysaccharides are digested to monosaccharides before absorption (Figure 30-10). Membranous-phase digestion of peptides is, in some respects, similar to that of carbohydrates; peptide-digesting enzymes, or peptidases, are present on the enterocyte surface membrane and extend into the glycocalyx. These enzymes hydrolyze the peptide products of luminal-phase protein digestion, yielding free amino acids. Some of the longer-chain peptides are incompletely digested, yielding dipeptides and tripeptides. A large portion of dietary amino acids is absorbed directly in the form of dipeptides and tripeptides. This mode of absorption contrasts with that of carbohydrates, in which only monomeric, simple sugars may pass the apical membrane. Dipeptides and tripeptides that are absorbed intact are subsequently hydrolyzed by the action of intracellular peptidases, which results in the formation of free amino acids that are then available for passage into the blood. Thus the final digestion of peptides to free amino acids may occur at either of two sites: on the surface membrane of the enterocyte or within the cell. In either case, the final product of protein digestion is free amino acid (Figure 30-11). Absorption refers to the movement of the products of digestion across the intestinal mucosa and into the vascular system for distribution. To better understand the physiologically eloquent and clinically important processes of intestinal absorption, the reader might need to review the processes of diffusion across membranes, the difference in composition of intracellular and extracellular fluid (see Chapter 1), the electrical polarity across cell membranes, the function of the sodium-potassium (Na+,K+) adenosine triphosphatase (ATPase) pump, and the function of selective ion channels (see Chapters 1 and 4). Active transport involves the direct consumption of metabolic energy. During active transport, energy stored as ATP is expended to move ions or molecules across membranes against an electrical or chemical gradient. In the large and small intestine, the active transport pathway of greatest importance is the Na+,K+-ATPase pump. This protein pathway lies on the basolateral membrane and uses energy from the hydrolysis of one molecule of ATP to drive three ions of sodium out of the cell, in exchange for the entry of two potassium ions into the cell. This important transport pathway exists in a wide variety of cells, in addition to enterocytes. The Na+,K+-ATPase pump is the mechanism by which (1) the interior of cells is kept electrically negative with respect to the extracellular fluid and (2) the concentration of sodium is kept very low in the intracellular fluid (see Chapter 1).
Digestion and Absorption
The Nonfermentative Processes
Digestion and Absorption Are Separate, but Related, Processes
The Small-Intestinal Mucosa Has a Large Surface Area and Epithelial Cells with “Leaky” Junctions Between Them
Digestion
Breaking Down Food Particle Size by Physical Action Is an Important Part of the Digestive Process
Chemical Digestion Results in the Reduction of Complex Nutrients into Simpler Molecules
Luminal-Phase Carbohydrate Digestion Results in the Production of Short-Chain Polysaccharides
Luminal-Phase Digestion of Carbohydrates Applies Only to Starches, Because Sugars Are Digested in the Membranous Phase
Proteins Are Digested by a Variety of Luminal-Phase Enzymes
Enzyme
Action
Source
Precursor
Activator
Pepsin
Endopeptidase
Gastric glands
Pepsinogen
Hydrochloric acid, pepsin
Chymosin (rennin)
Endopeptidase
Gastric glands
Chymosinogen
?
Trypsin
Endopeptidase
Pancreas
Trypsinogen
Enterokinase, trypsin
Chymotrypsin
Endopeptidase
Pancreas
Chymotrypsinogen
Trypsin
Elastase
Endopeptidase
Pancreas
Proelastase
Trypsin
Carboxypeptidase A
Exopeptidase
Pancreas
Procarboxypeptidase A
Trypsin
Carboxypeptidase B
Exopeptidase
Pancreas
Procarboxypeptidase B
Trypsin
Membranous-Phase Digestion Occurs Within the Microenvironment of the Unstirred Water Layer, Intestinal Mucus, and Glycocalyx
A Specific Membranous-Phase Enzyme Exists for the Digestion of Each Type of Polysaccharide
Complete Digestion of Peptides to Free Amino Acids Takes Place Both on the Enterocyte Surface and Within the Cells
Intestinal Absorption
Specialized Nutrient Transport Systems Exist in the Apical and Basolateral Membranes
Stay updated, free articles. Join our Telegram channel
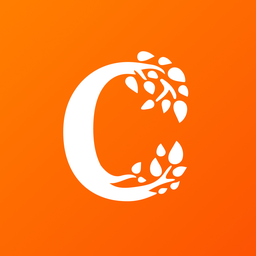
Full access? Get Clinical Tree
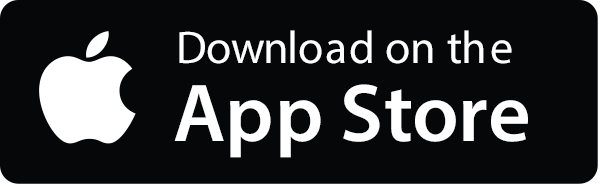
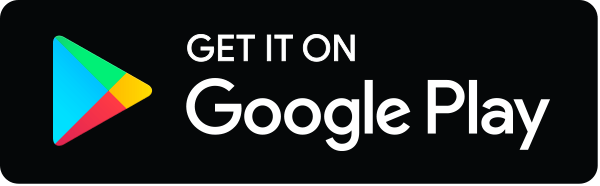