and Daniel T. Laskowitz1
(1)
Division of Neurology, Duke University, Durham, NC, USA
Abstract
Frontotemporal dementia (FTD) is a multifaceted syndrome with a high degree of clinical and neuropathological variability, an extensive genetic contribution, and involvement of multiple proteins. FTD accounts for up to 50% of dementias with the onset prior to age 60. The heterogeneous genetic, clinical, and pathological manifestations of FTD have created challenges in generating clinically relevant animal models with which to test new therapeutic approaches. Nevertheless, tau transgenic models have been developed in mice, Drosophila melanogaster, and Caenorhabditis elegans in the past decade. These models have played an important role in elucidating a number of mechanisms associated with tau FTD-related neurodegeneration, and it is likely that these preclinical models will help to facilitate new therapeutic strategies. It is clear that both wild-type and mutated tau protein are sufficient to elicit tauopathy, although mutated tau increases the severity of the pathology. Furthermore, the aberrant expression and/or incorrect temporal/developmental expression of tau may also cause tau pathology. Importantly, these tau models have clarified some long-held theories pertaining to tau and neurodegeneration. For example, it has been shown that oxidative stress plays a crucial role in FTD and that tau pathology reactivates the cell cycle machinery. Conversely, tau aggregates are not necessary for tau neurotoxicity. However, new models representing other forms of FTD need to be developed and much work still remains before the disease is clearly understood and disease-modifying therapies become available.
Key words
FTDAnimal modelsTransgenicTauProgranulinTDP-43Neurodegeneration1 Introduction
Frontotemporal dementia (FTD), previously known as Pick’s complex, is a multifaceted syndrome with a high degree of clinical and neuropathological variability, an extensive genetic contribution, and involvement of multiple proteins. FTD accounts for up to 50% of dementias with the onset prior to age 60 (1). The heterogeneous clinical and pathological manifestations of FTD have created challenges in generating clinically relevant animal models with which to test new therapeutic approaches. Nevertheless, much has been learned from current transgenic models of mice, Drosophila melanogaster (Drosophila), and Caenorhabditis elegans (C. elegans) in the past decade. Some molecules and mechanisms that play crucial roles in FTD-related neurodegeneration have been delineated; however, much work still remains before the disease is clearly understood and palative therapies are available.
1.1 Background of FTD
The original name for FTD was Pick’s disease (PiD), which has led to some confusion in nomenclature. In 1882, Arnold Pick first described PiD as a clinical picture of frontotemporal atrophy (2). Subsequently, Aloysius Alzheimer described this case of PiD as distinct from Alzheimer’s disease based on histology characterized by the presence of large, dark-staining aggregates of proteins (Pick bodies) that were present in the absence of plaques or tangles (3). However, we now know that all cases with frontotemporal atrophy do not present with Pick bodies. Eventually, the general clinical syndrome was defined as FTD. Diagnoses of PiD are now reserved for only a subset of FTD that presents postmortem with Pick bodies.
Pathologically, all FTD include frontotemporal lobar atrophy, neuronal loss, gliosis, and superficial spongiosis. Although rarely present at the onset of the disease, cognitive impairment is a constant feature of FTD (4–7). The onset of FTD, however, can present with a variety of clinical manifestations (please see Table 1
) with the most common variant presenting first with progressive deterioration of behavior and personality (FTD-bv) (8). FTD often initially presents with language dysfunction, Primary Progressive Aphasia (PPA), where a loss or impairment of the power to use or comprehend words occurs, and semantic dementia (SD), which presents with a comprehension deficit of the meaning of nouns and objects. Finally, the onset of FTD may first present with extrapyramidal symptoms such as progressive supranuclear palsy (PSP; characterized by bulbar symptoms) and corticobasal degeneration (CBD, associated with apraxia). However, after onset, patients may present with one or more of the other initial clinical syndromes causing the distinction between these clinical phenotypes to be unclear. Additionally, several different FTD symptoms may appear in one family, and even vary within a family where a single mutation has been identified, thus emphasizing the fact that other genetic and environmental factors play significant roles in the disease.
Table 1
Clinical manifestations of frontotemporal dementia
Syndrome at onset | Description | Pathology a |
---|---|---|
FTD-bv | Patients present with disinhibition, apathy, loss of judgment and insight, negligence of personal hygiene, verbal and physical aggressiveness | MNDI, DLH, Pick bodies, CBD |
Frontotemporal dementia – behavioral variant | ||
PPA | Patients become increasingly nonfluent with increasing aphasia (impairment in the use and comprehension of words) and logopenia (word-finding difficulty) | MNDI, CBD, PICK’S BODIES |
Primary progressive aphasia | ||
SD | Patients remain fluent while developing progressive deterioration of understanding and recognizing words (meaningless speech) | |
Semantic dementia | ||
CBD | Patients develop unilateral rigidity, apraxia, alien hand | CBD, PSP |
corticobasal degeneration | ||
PSP | Patients develop vertical gaze palsy, falling, axial rigidity, pseudobulbar palsy | |
Progressive supranuclear palsy |
Table 2
Postmortem pathology in frontotemporal dementia
Histological sub-types of FTD pathology | Syndrome-specific pathology | Histo and immuno reactivity |
---|---|---|
PD | Pick bodies (PB) – round or oval compact intracytoplasmic neuronal inclusions, microvacuolation | Bielschowsky but not Gallyas, tau immunoreactivity |
Pick’s Disease | ||
CBD | Ballooned achromatic neurons, intracytoplasmic neuronal inclusions of variable morphology, pervasive gray and white matter threads, astrocytic plaques | Tau positive – intracytoplasmic inclusions, threads, oligodendrocytes and glial plaques |
Corticobasal degeneration | ||
Bielschowsky and Galyas positive intracytoplasmic inclusions and threads | ||
PSP | Predominantly subcortical distribution of intracytoplasmic neuronal inclusions of variable morphology, tufted astrocytes | Tau-positive tangles and tufted astrocytes |
Progressive | ||
MNDI | Neuronal intracytoplasmic and nuclear inclusions, cortical microvacuolation, in fascia dentate, and cerebral cortex | Ubiquitin-positive neurons and dystrophic neurites, gliosis, TDP-43-positive neuronal inclusions |
Motor neuron type inclusions | ||
DLDH | Cortical microvacuolation, neuronal loss, and gliosis | Tau and ubiquitin negative, gliosis |
Dementia lacking distinctive histology |
Given the clinical heterogeneity of FTD, a more complete understanding of its neuropathology has played a central role in the characterization of the disease process, and ultimately, in the creation of relevant animal models. Neurofibrillary tangles (NFTs) containing hyperphosphorylated aggregates of the microtubule-associated protein tau in cell bodies are abundant in FTD (Table 2). However, some patients with FTD have tau-negative, ubiquitin-positive lesions and some patients have no identifiable lesions at all (Table 2). Therefore, FTD is now further subclassified according to histochemical and immunocytochemical properties of the material accumulated in neuronal cells: (1) Tau-positive variant of FTD (FTD-T), (2) Ubiquitin-positive, tau-negative variant of FTD termed frontotemporal lobar degeneration with ubiquitin inclusions (FTLD-U), and (3) a variant with no identifiable accumulation termed dementia lacking distinctive histology (DLDH).
1.2 FTD Genetics
Great progress has been made over the past decade in defining the genetic basis of FTD. To date, mutations associated with FTD have been identified in the following three genes: MAPT, which encodes for tau (9, 10); PRGN, which encodes for progranulin (11, 12); and VCP, which encodes for the valosin-containing protein (13). In 1997, a consensus conference designated a subset of FTD as FTDP-17 (frontotemporal dementia and parkinsonism linked to chromosome 17 (14), which was soon followed by seminal publications identifying both exonic and intronic mutations in the MAPT gene (9, 10, 15). Of the 40-plus known MAPT mutations (16), several have been expressed in transgenic mice, Drosophila, and C. elegans, confirming that tau dysfunction is causal in neurodegeneration and dementia.
Biochemically, tau was one of the first proteins identified within the intracellular inclusions in FTD. Tau accumulation can be somal, in dystrophic neurites, and/or in glia and it is the hallmark of several other diseases besides FTD, collectively called tauopathies. Tau protein in these cellular lesions can be hyperphosphorylated, ubiquitinated, and carboxy-terminal truncated.
Although most cases of FTLD-U are sporadic, the efforts of several groups led to the identification of familial disease resulting from the loss-of-function mutations in PRGN implicating a haploinsufficiency mechanism (11, 12, 17). While it is poorly understood why a partial loss of PGRN leads to FTLD-U, the marked reduction of PGRN protein in this disease points toward a function of PGRN in neuronal survival. However, as with most FTDs, the age of onset in families segregating with the same PRGN mutation varies widely as do the symptoms suggesting the existence of further modifying factors (18).
Interestingly, unlike tau protein, mutated PGRN does not accumulate in the neuronal cells of FTLD-U patients. Instead, the TAR DNA sequence-binding protein (TDP-43) accumulates in ubiquitin-positive inclusions in FTLD-U patients with PGRN mutations (19). Similar to accumulated tau protein in cells, TDP-43, in cell lesions, is hyperphosphorylated, ubiquitinated, and carboxy-terminal truncated (19, 20). TDP-43 is encoded by the TARDBP gene located on chromosome 1 and is a highly conserved ubiquitously expressed nuclear protein implicated in repression of gene transcription, inhibition of exon splicing, and interactions with splicing factors and nuclear bodies. Although accumulated TDP-43 is found in approximately half of all FTD cases, no mutations have been associated with the disease. However, mutations in the TARDBP gene have been identified in both familial and sporadic amyotrophic lateral sclerosis (ALS) (21–24).
It is important to note that TDP-43 inclusions were also discovered in the autosomal dominant inclusion body myopathy associated with Paget disease of the bone with frontotemporal dementia (PDB-FTD) (25, 26). Although not classified as typical FTD, patients with this disease develop dementia that is typical of FTD and is characterized by language and/or behavioral dysfunction with relative preservation of memory (27, 28). Although no mutations were associated with the TARDBP gene in PDB-FTD, mutations in the valosin-containing protein (VCP), which is an essential component of the endoplasmic reticulum-associated degradation process, were associated with this disease (13). No VCP transgenic animal models have been reported to date.
It is likely that the disruption of synaptic function leading to neuronal death is the common neuropathological feature leading to the diverse clinical manifestations associated with FTD. However, there are many pathways that can lead to the final common denominator of synaptic dysfunction, perhaps explaining the wide variety of genes involved. The distinctive patterns that characterize the cognitive and behavioral deficits may reflect the neural circuitry that is affected.
An increased understanding of the neuropathology and genetic influences associated with FTD has aided the development of relevant animal models, which can be used both to enhance our fundamental understanding of disease pathogenesis and to develop screens that aid in the development of new therapeutic strategies. Since it is not possible to perform screens in humans, animal models have become the foundation for studies designed to advance our understanding of disease progression. In the case of FTD, many animal models have been created ranging from the simple C. elegans, zebrafish, and Drosophila to the more complex mammalian mice and rats. Using these models, abnormal accumulation and misfolding of synaptic and cytoskeletal proteins are being extensively explored as a key pathogenic event leading to neurodegeneration in FTD. Additional models have also been generated using FTD familial mutations.
2 Murine Tau Models
Biochemically, tau was one of the first proteins identified within the intracellular inclusions in FTD. Hence, many of the animal models of FTD to date have been engineered to overexpress either normal human tau or FTDP-17 mutated human tau. Tau protein is a neuron-specific protein that binds and stabilizes microtubules that are involved in axonal transport (29–34). The alternative splicing of exons 2, 3, and 10 of tau is developmentally, physiologically, and spatially regulated. In the fetal brain, only the shortest isoform lacking exons 2, 3, and 10 is expressed. As the brain matures to adulthood, differential splicing of exons 2, 3, and 10 results in the generation of six tau isoforms (mRNA and protein) (35–37). These tau isoforms are typically distinguished by the presence of either three (3R) or four (4R) tandem repeats of the microtubule binding domain. The 4R tau isoforms contain additional amino acids encoded by exon 10 that are absent in the 3R isoforms (38, 39).
NFTs are the intracellular lesions found in the tau-positive subset of FTD and are composed of filaments of hyperphosphorylated tau. NFTs correlate well with cognitive deficits and neuron loss, and have been implicated in mediating neurodegeneration and dementia in FTD and other tauopathies. The association between NFTs, neuron loss, and brain dysfunction in humans has led some to posit that NFTs invariably cause brain dysfunction and neurodegeneration. As a result, the gold standard of tauopathy models has been the generation of NFTs.
2.1 Murine Models Expressing Normal Human Tau
Initially, starting as early as 1995, transgenic mouse models of FTD were created by expressing human tau protein from cDNA constructs of various tau isoforms under the regulation of heterologous promoters. The original theory was that the overexpression of normal tau protein would model clinical neuropathology by generating tau NFTs. Thus, mice expressing the longest (including exons 2, 3, and 10) or shortest (without exons 2, 3, and 10) human tau isoforms were engineered. Human tau protein was expressed under the regulation of the Thy-1 promoter by different groups. The model of Gotz et al. (1995) (40) displayed tau protein expression 10% of endogenous mouse tau levels, whereas the models of Spittaels et al. (1999) (41) and Probst et al. (2000) (42) exhibited tau protein expression 150–400% and 130% of endogenous mouse tau, respectively. Other models were created that expressed human tau under the regulation of (1) the hydroxy–methyl–glutaryl CoA reductase (HMG-CR housekeeping gene) promoter, with protein expression levels of approximately 200% of endogenous mouse tau developed by Brion et al. (1999) (43), and (2) the murine prion protein (PrP) promoter, protein expression 500–1,500% of endogenous mouse tau developed by Ishihara et al. (1999) (44).
All the models developed somatodendritic relocalization of tau protein, which was believed to be the pretangle stage of tau pathology. Immunopositivity with phosphorylation and conformation-specific antibodies in neurons and astrocytes were also seen in all these models; however, it is important to note that most of these antibodies are also immunoreactive to some extent to normal tau. As with all tau overexpressing models, caution should be taken that observed changes are a result of tau pathology and not a fortuitous artifact of overexpression. Of these models (Spittaels (41), Probst (42) and Ishihara (44)), the ones expressing high levels of human tau also presented with axonal dilations associated with abnormal tau and neurofilament spheroids in the spinal cord. These lesions were functionally associated with motor deficits.
Spittaels et al. further extended these results and developed several transgenic lines with increasing amounts of tau protein overexpression ranging from 150 to 400% of endogenous mouse tau (41). In these lines, a clear correlation was observed between the degree of tau overexpression and increased pathology. Axonopathy in brain and spinal cord were transgene dosage-related but no other characteristics of FTD such as tangles, astrogliosis, ubiquitination, or motor deficits correlated with the level of tau expression. Similarly, the Ishihara et al. model expressing the highest levels of human tau protein not only developed axonopathy, but also motor dysfunction, weight loss, and exhibited a decreased rate of survival (44). Although this model recapitulated many of the clinical features observed in patients with ALS, it failed to model many of the cardinal cognitive and extrapyramidal features of FTD. However, these models clearly demonstrated that increasing levels of tau protein were detrimental to the nervous system.
Interestingly, mice expressing human tau protein from human tau promoters, from the human tau gene at approximately 300% endogenous mouse tau levels (45, 46), and from a cDNA of the longest tau isoform at approximately 100% endogenous mouse tau levels (47) resulted in minimal tau pathology. It would be expected that the expression of tau protein under the regulation of the human tau promoter would be in a more physiological manner temporally and developmentally than when tau protein is expressed from heterologous promoters. It is therefore possible that the tau pathology in mouse models with heterologous promoters may in large part be attributed to nonphysiological patterns of expression. A conclusion that can be drawn from these early models is that the overexpression and/or incorrect temporal/developmental expression of normal tau is by itself causal in tauopathy.
2.2 Murine Models Expressing Mutated Human Tau
Mutations in the tau gene, MAPT, were identified in patients with FTDP-17 in 1998 (9, 10, 15). These mutations and their consecutive expression in transgenic animals confirmed that tau dysfunction can cause neurodegeneration and dementia. The FTDP-17 associated tau mutations are located predominantly in the microtubule-binding region, indicating that this region is highly sensitive to disease-causing mutations. Mutations in this region would disrupt the microtubular network with downstream effects on a variety of intracellular processes, such as intracellular transport, metabolism, mRNA trafficking, protein sorting, and targeting. These, in turn, could have potential consequences for the maintenance of neuritic structures and synaptic plasticity. The mutations in the TAU gene fall under two categories: exonic mutations that directly alter the structure and function of tau protein by amino acid alterations, and intronic or exonic mutations that indirectly alter the structure and function of tau protein by altering the splicing of exon 10. Mutations that alter the splicing of exon 10 consequently affect the relative ratio of 4R to 3R microtubule-binding repeats and hence the tau/microtubule interactions.
2.3 Biochemical Mutations
Although NFTs are one of the main pathological hallmarks of tau FTD (48), they were not reproduced until the expression of human tau protein carrying a biochemical FTDP-17 mutation was introduced into animal models. The first model that reproduced NFTs and extensive hyperphosphorylation was the JNPL3 mouse that expressed the P301L mutation under the regulation of the prion promoter (49). The JNPL3 mice express human tau protein at approximately 300% the level of endogenous mouse tau and develop NFTs, neuropil threads, motor deficits, and neuronal cell loss and invariably develop severe motor deficits that lead to hind limb paralysis and death. Furthermore, the mice also develop decreased grooming habits, a behavior that may be indicative of FTD. However, by the time the grooming deficits occur, the mice are in poor physical shape and it is therefore difficult to discern whether this lack of grooming is due to cognitive deterioration or physical deficits. These mice have been widely utilized for understanding some of the mechanisms of FTD tauopathy. Several other models using the same or different FTDP-17 mutations were also engineered, all reproducing similar results. Based on the success of these models, a second generation of models were generated with varying degrees of tau expression by a number of investigators, namely Gotz et al. 2001 (50) (P301L, 70% of endogenous tau), Gotz et al. 2001 (51) (G272V, 1% of endogenous tau), Allen et al. 2002 (52) (P301S, 200% of endogenous tau), Tanemura et al. 2002 (53) (V337M, 100% of endogenous tau), and Tatebayashi et al. 2002 (54) (R406W, 14% of endogenous tau). All the mice developed varying amounts of tau pathology generally in the form of abnormal, tau-reactive nerve cell bodies and dendrites and large numbers of pathologically enlarged axons containing tau-reactive spheroids. A high percentage of the mice also develop glial tau pathology similar to that seen in PSP and CBD. The majority of the tau mutant mice developed motor deficits, ranging from hind limb clasping, to balance beam, rotarod, and in some cases cognitive deficits. A few of the later models also developed severe motor deficits similar to the JNPL3 mice.
Some of the tau pathology and behavior deficits are similar to what is seen in human wild-type tau transgenic mice; however, the mutations accelerate these phenotypes. As in the wild-type transgenic models, the levels of mutated tau overexpression increase the tau pathology and related behavior deficits as homozygous transgenic mice generally show an earlier onset of tau accumulation and motor deficits. All the above-mentioned models express tau protein under the regulation of nonhomologous promoters. It is therefore important to note that our laboratory has generated individual cDNA mutant transgenic mice with all of the above mutations (P301L, G272V, V337M, R406W) expressing human tau at 20–80% of endogenous mouse tau under the regulation of the human tau promoter, without reproducing any of the tauopathy (unpublished data). Thus, as suggested in the discussion of the wild-type transgenic models, it is very likely that tau protein is more physiologically expressed from the human tau promoter than from heterologous nontau promoters and it may be crucial for normal tau function to be expressed in the correct temporospatial pattern.
< div class='tao-gold-member'>
Only gold members can continue reading. Log In or Register a > to continue
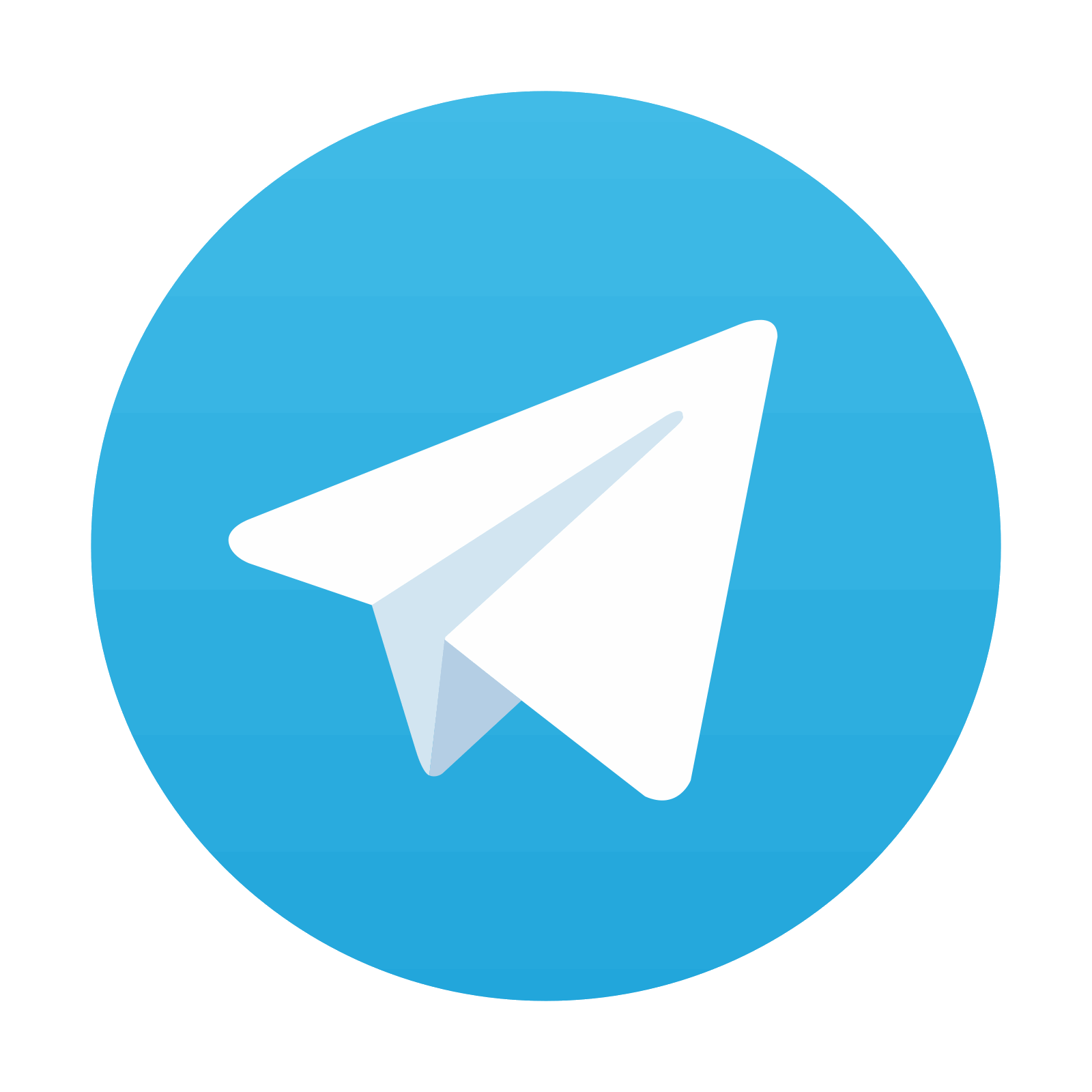
Stay updated, free articles. Join our Telegram channel
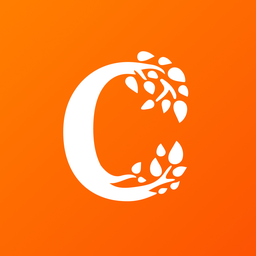
Full access? Get Clinical Tree
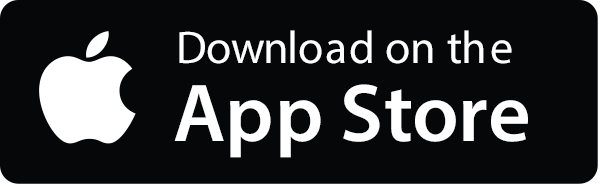
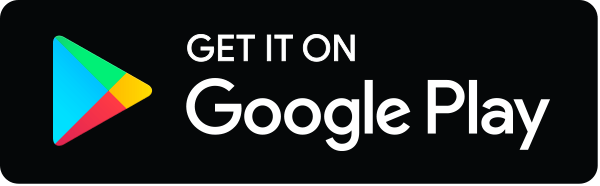