, Heidi N. Boyda1, 2 and Ric M. Procyshyn2, 3
(1)
Department of Anesthesiology, Pharmacology & Therapeutics, University of British Columbia, Vancouver, BC, Canada
(2)
BC Mental Health & Addiction Research Institute, Vancouver, BC, Canada
(3)
Department of Psychiatry, University of British Columbia, Vancouver, BC, Canada
Abstract
Drugs of abuse generate diverse behavioral and physiological effects. One feature common to many abused drugs is the phenomenon of “withdrawal,” which results from abrupt termination of drug administration. The initial phases of drug withdrawal, often referred to as the “crash” phase in humans, are characterized by dramatic psychological changes which may or may not be accompanied by somatic symptoms. The psychological symptoms, including disruptions in affect and cognition, are most commonly observed following psychostimulant withdrawal. This transient state strongly resembles the symptoms of major depressive disorder (MDD) in humans. The majority of rodent paradigms of psychostimulant withdrawal accurately model this human condition, and sophisticated preclinical protocols have been used to quantify psychological changes, such as anhedonia. In the present review, we describe past and current progress in modeling psychostimulant withdrawal in rodents, as well as potential limitations of this model.
Key words
AmphetaminesAnhedoniaBehaviorCocainePsychostimulantRodentWithdrawal1 Introduction
As discussed elsewhere in this book in detail, most drugs of abuse have acute physiological effects in both humans and animals. Many of these effects represent a strong deviation from the body’s natural state of equilibrium, and therefore endogenous homeostatic mechanisms are recruited throughout not only the body, but also the central nervous system, in order to counter and reduce the ongoing effects of the drug (1). In general, the greater the concentration and the longer the duration of drug exposure, the more pronounced the homeostatic counteradaptations (2). At some point during drug taking, either for voluntary or involuntary reasons, drug intake ceases. From that point on, the physiological effects of the drug rapidly decrease as the remaining drug is metabolized and cleared from the body. By contrast, the drug counteradaptations typically do not diminish nearly as rapidly. The body is therefore left in a state where potent homeostatic mechanisms remain essentially unopposed; this represents one aspect of the complex phenomenon described as “drug withdrawal.” The dramatic “somatic” withdrawal symptoms that are frequently associated with termination of central depressants, such as heroin and alcohol, come most vividly to mind when considering the effects of drug withdrawal (3, 4). These include sweating, muscle aches and cramping, nausea, vomiting and diarrhea for opiates, and tremors, seizures, and even death for alcohol. Eventually the counteradaptations subside, but this may require considerable time.
In a similar manner to the overt physiological effects of abused drugs, the acute psychological and pleasurable effects of drugs of abuse are also countered, presumably as a consequence of homeostatic physiological adaptations. During the period of drugs withdrawal, adaptations to prolonged drug exposure contribute to a cluster of psychological symptoms (5, 6). For over three decades, these psychological effects of drug withdrawal have been described within the theoretical framework of an opponent-process theory of motivation (7) (see Fig. 1). Consistent with this theory, the previously pleasurable effects of different drugs of abuse are inevitably followed during withdrawal by emotional states opposite in effect, and of a longer duration, as the body seeks to restore its “hedonic equilibrium.” This dysphoric state, whose psychological, behavioral, and physiological indices represent the operational definition of the term “withdrawal” as used in this synopsis, are most pronounced after abstinence from classical psychoactive drugs, which include both depressants and stimulants. However, in the case of depressant drugs, the marked somatic symptoms of withdrawal represent a potent confound in measuring the more subtle psychological effects, particularly when studying withdrawal effects in animal models (5). This can be due to interference of physical symptoms on dependent measures that are utilized to assess underlying psychological states, or because the physical symptoms themselves are distressing.
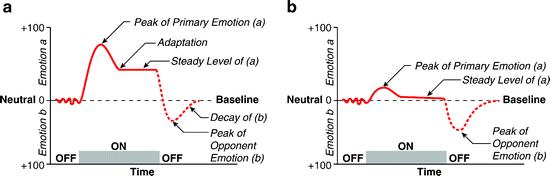
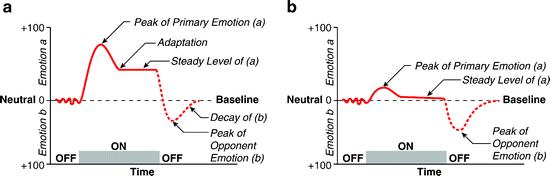
Fig. 1.
The opponent-process theory of motivation, as envisioned by Solomon and Corbit (1974). According to the model, emotional stimuli or experiences evoke one emotional state (a), which then triggers an opponent state (b), which serves to bring the emotional state back to a neutral or baseline point. (a) Initial presentation of stimulus. The first stimulus present elicits a large emotional change (a). The (b) state persists beyond the stimulus presentation, leading to an emotional change that is opposite to the (a) state. (b) Stimulus presentation after repeated exposures. With experience, the (b) process increases in strength and duration such that it is more effective at countering the emotional changes associated with the (a) state. This leads to a larger peak of the opponent emotional change after the stimulus is removed.
For this reason, the most informative work on the psychological effects of drug withdrawal has been performed with animal models of exposure to psychostimulant drugs. This class of drugs represents a range of different compounds, which include milder central nervous system stimulants (typically legal) such as caffeine and nicotine, through to potent stimulants (typically illegal) such as cocaine and methamphetamine. While exposure to higher doses of stimulant drugs, particularly nicotine, can result in some somatic symptoms during withdrawal (8, 9), these effects are much less obvious and severe than with the depressants. Thus, there are far fewer confounding physical symptoms to interfere with measurement of underlying psychological states in psychostimulant withdrawal. Thus, rodent paradigms of psychostimulant withdrawal have allowed the detailed investigation of the complex behavioral and affective sequelae associated with the termination of drug intake.
2 Neurobiology of Psychostimulant Drugs
2.1 Molecular Mechanisms of Psychostimulants
Uncovering the neural substrates and molecular mechanisms of psychostimulant drugs provides insight into the physiological counteradaptations that accompany drug withdrawal. Psychostimulants activate the central nervous system directly, with the more potent drugs increasing the activity of both peripheral and central monoaminergic and cholinergic systems. A subclass of psychostimulant drugs acts on the central nervous system mainly through non-exocytic mechanisms. The mechanism of action of amphetamines, a commonly used class of psychostimulant drug, has been described in extensive detail recently in several excellent reviews (10, 11). The principal mechanisms underlying amphetamines’ physiological properties are outlined in Fig. 2. The large majority of studies have focused on the capacity of amphetamines to modulate dopamine release, due to this neurotransmitter’s crucial role in addiction, and therefore we have a much more detailed understanding of how amphetamines act on dopamine substrates than we do for other monoamines, such as serotonin and norepinephrine.
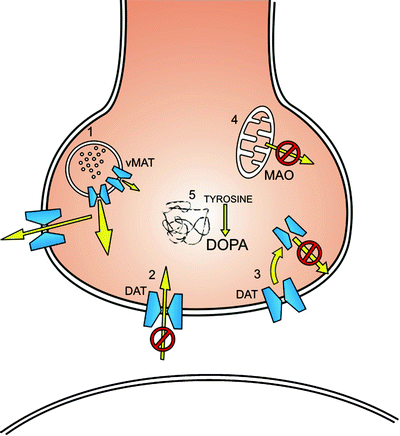
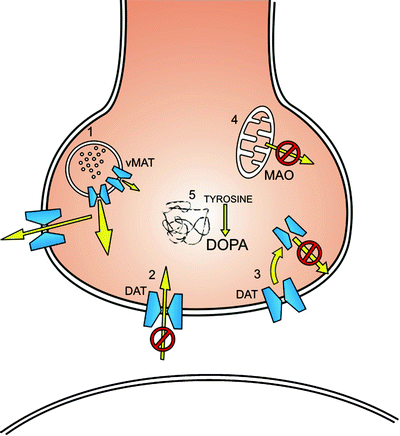
Fig. 2.
Physiological mechanisms by which amphetamines increases synaptic levels of monoamines, principally dopamine. Mechanisms include the redistribution of catecholamines from synaptic vesicles to the cytosol (1), and the reverse transport of neurotransmitter through plasma membrane transporters. In addition, amphetamines, like cocaine, block the activity of monoamine transporters (2) and decrease the expression of dopamine transporters at the cell surface (3). Amphetamines can increase cytosolic levels of monoamines by inhibiting the activity of monoamine oxidase (4), and increase the activity and expression of tyrosine hydroxylase (5). DAT dopamine transporter, MAO monoamine oxidase, vMAT vesicular monoamine transporter.
Earlier studies characterized several biochemical pathways that modified dopamine levels in the brain. Reports in the 1970s and early 1980s observed that treatment with amphetamines enhanced dopamine synthesis by increasing tyrosine hydroxylase activity (12, 13). This effect, though, may be biphasic, with lower to medium levels of drug increasing enzyme activity, but higher levels of amphetamines inhibiting dopamine synthesis. The physiological mechanisms that account for increased tyrosine hydroxylase activity are largely unknown. Amphetamines also increase dopamine levels by inhibiting monoamine oxidases (MAO), the enzymes that are present on the outer mitochondrial membrane that are responsible for amine catabolism in presynaptic terminals. Amphetamines are competitive inhibitors of MAO. Evidence indicates that some amphetamines exhibit a differential affinity for MAO-A versus MAO-B (14).
Recent examination of the mechanism of action of amphetamines has focused on two main molecular substrates on dopamine neuronal terminals: the plasmalemmel dopamine transporter (DAT) and the vesicular monoamine transporter-2 (VMAT-2). The plasmalemmel DAT (15) has been studied most extensively in its relation to the physiological effects of amphetamines, although the results of studies examining the norepinephrine (NET) and serotonin (SERT) transporters are generally consistent with the DAT studies. Considerable evidence suggests that amphetamines are a substrate of the Na+/Cl−-dependent DAT. At a molecular level, based on the exchange-diffusion model (11), amphetamines compete with synaptic dopamine at the extracellular site on the DAT. As concentrations of dopamine are much greater inside the cell than outside, and the DAT can transport dopamine in a bidirectional manner, the binding of amphetamines to the extracellular side of the transporter has the net effect of causing cytosolic dopamine to be reverse-transported outside of the cell. At higher concentrations of amphetamines, these lipophilic drugs diffuse directly across the plasmalemmel membrane and increase cytosolic concentrations of dopamine, meaning that this process may not even require binding to the extracellular site of the DAT. The activity of the DAT in the exchange diffusion model is highly dependent on a number of cell signaling pathways, as this molecular mechanism is closely regulated. Key signaling pathways include phosphatidylinositol 3-kinase (PI3K) and calmodulin-dependent protein kinase II (16, 17).
The DAT is able to cause non-vesicular release of dopamine through a second mechanism in addition to the slow exchange process, which has been characterized in stably expressed heterologous cell systems. This process involves a conformational change of the DAT into a “channel-like mode” that enables brief bursts of a dopamine efflux at a concentration resembling vesicular exocytotic release (18). Importantly, the DAT can also be internalized as part of an endocytotic recycling pathway. This property removes it from the plasmalemmel membrane and thus obviates its capacity to reduce synaptic levels of dopamine. In vitro studies in heterologous cell systems note that amphetamine drugs increase DAT internalization (19). This process remains an area of ongoing study, but there is mounting evidence that amphetamines increase the formation of DAT complexes, which may affect the rate at which the transporter can be recycled back to the cell surface (20).
The second major molecular substrate of amphetamines is the VMAT-2, which is an integral membrane protein that transports monoamines from the intracellular cytosol into synaptic vesicles, using a vacuolar-type H+ pumping ATPase (21). The ATPase produces a pH gradient across the vesicle membrane, with a mildly acidic vesicular internal environment of approximately pH 5.5 (22). According to the “weak base hypothesis” (11), amphetamines cause synaptic vesicles to release dopamine and other monoamines into the surrounding presynaptic cytosol by reversing the proton gradient between the inside and outside of the vesicle. Amphetamines are weak bases, with a pK a of 9.9 (23). It is hypothesized that when these basic amphetamines enter the vesicle in sufficient concentrations, the internal pH is disrupted. This decreases the capacity of the vesicle to store monoamines. Although this theory continues to remain a leading hypothesis for the mechanism of action of amphetamines, there is recent evidence that this effect can only occur at drug concentrations well above those when monoamine uptake by the VMAT-2 is evident (24). Thus, at lower concentrations, amphetamines may compete with the substrate of the VMAT-2 and inhibit, in a dose-dependent manner, the accumulation of the monoamines in the synaptic vesicles (25). Alternately, the role of VMAT-2 in the amphetamines’ action may involve its redistribution from the synaptic vesicle to an as-yet-unidentified cellular location (11). Converging evidence from experiments with rat striatal preparations note that multiple, high doses of amphetamines, designed to mimic human “binge”-like behavior, cause decreased vesicular uptake of dopamine. These studies noted that, while levels of the VMAT-2 were decreased in preparations that included only the vesicles, the concentration of VMAT-2 in entire homogenates remained unaltered (26, 27). These intriguing findings suggest that there was a redistribution of the VMAT-2 to an alternate, unknown, cellular location.
2.2 Action of Psychostimulants at a Systems Level
The effects of amphetamines and most other psychostimulants in the brain, therefore, are largely a result of the stimulation of monoamines. Based on local distribution of monoamine terminals, levels of monoamines increase in a regionally dependent manner. For example, monoamine release is greatest in regions of the densest monoamine terminal innervation, such as dopamine release in the striatum. Preclinical studies in nonhuman primates with procedures such as cerebral microdialysis have enabled us to measure directly extrasynaptic levels of monoamines; these studies indicate that monoamines can be substantially elevated after even single acute drug doses. For instance, a single dose of 1 mg/kg of methamphetamine in adult male rhesus monkeys elevated dopamine levels in the striatum by 1350% compared to baseline within 30 min, and remained close to 500% above baseline 2.5 h later (28). Given the ubiquitous functions and distribution of monoamines in the brain, it is likely that many other neurotransmitters and neuropeptides are altered in a secondary manner following the dramatic elevations in monoamines caused by psychostimulants.
Although it is not a focus for many preclinical studies, psychostimulants also have potent physiological effects on peripheral systems. It is therefore unclear whether peripheral changes contribute to the psychological effects of stimulant drugs and influence drug withdrawal. As an example, amphetamines stimulate epinephrine and norepinephrine release by the medulla of the adrenal glands, and can simulate the effects of activation of the sympathetic division of the central nervous system during a stress response (29). Thus, psychostimulants can acutely cause an acceleration of heart and lung action through vasoconstriction and bronchodilation respectively, while muscle activity is primed via transient hyperglycemia and dilation of blood vessels in skeletal muscle. Nonessential physiological activity, such as stomach and intestinal function, is inhibited by many stimulants. Levels of cortisol (corticosterone in rodents) and adrenocorticotropic hormone, the major peripheral stress hormones, are increased by over 200% following administration of amphetamines (30) and remain elevated for hours after the final exposure to the drug. Chronic overstimulation of the stress response by psychostimulant use may exceed the capacity of the organism to maintain normal health – a term referred to as “allostatic” load (31) – which can result in a range of medical problems, including severe cardiovascular complications related to chronic hypertension and cardiovascular disease. Additional medical problems in human psychostimulant users include respiratory symptoms, infectious disease, dental sequelae, and malnourishment. It is worth remembering that these medical comorbidities are rarely considered in rodent models of drug administration and withdrawal, yet they detract significantly from the quality of life for many stimulant drug abusers, and may be of greater concern for addicts than the less life-threatening psychological effects of drug withdrawal.
3 Effects of Psychostimulants in Humans
In order to model the effects of psychostimulant withdrawal in animals, it is first necessary to understand the phenomenon in humans. Fortunately, the behavioral and psychological effects of drug exposure and withdrawal have been well described for multiple psychostimulant drugs, including amphetamines and cocaine (in its different forms).
3.1 Acute and Chronic Psychological Effects of Psychostimulants
Understanding the initial effects of psychostimulant drugs is important, as the opponent-process theory predicts that the effects of drug withdrawal will be opposite to the initial changes. The type and severity of the acute psychological effects of most psychostimulants depend on a number of variables, including both the method of administration, as well as the amount of drug used. The most rapid onset of psychological effects occurs after either smoking or intravenous injection of the drug. Oral ingestion or snorting of the drug produces a delayed onset of psychological effects and reduces bioavailability of amphetamines, due to first-pass metabolism. Controlled laboratory studies of acute, low-to-moderate doses of psychostimulants show that they cause cognitive changes that include increased arousal, enhanced concentration, and improved attention. In addition to cognitive changes, affective changes include decreased appetite and increased libido, as well as elevated mood and increased confidence (32). With higher acute doses, most psychostimulants may induce intense euphoria, often referred to as a drug “high.” However, when high doses of psychostimulants are administered for extended periods, they may cause dysphoria; symptoms include restlessness and anxiety, and are commonly associated with tremors and dyskinesia. With regards to “binge” drug use, which typically lasts over multiple days, the euphoric effects of the drug usually diminish while dysphoria and compulsive behavior increase throughout the course of the binge – an effect known as “tweaking”; this phenomenon is consistent with the predictions of the opponent-process theory. Chronic, binge users of psychostimulant drugs can exhibit highly focused and repetitive behaviors, known as “punding” (33), such as the stereotyped handling and sorting of objects. Importantly, substantial attention has been paid to the capacity of high and sustained doses of psychostimulants to cause psychological abnormalities that resemble the symptoms of psychosis (34). Psychotic symptoms frequently include sleeplessness, paranoia, hallucinations, and delusions (formication and grandiosity) and have been associated with irritability and unprovoked aggression. These latter physical and psychological symptoms that occur in chronic stimulant users represent a challenge to preclinical models of drug withdrawal at a number of levels. Firstly, they indicate that, with chronic use, the negative effects of drug exposure may be evident even before drug termination, and therefore the timing of withdrawal effects can be critical in animals. Secondly, the capacity of residual psychological symptoms such as psychosis, which can last well after drug termination and through the peak periods of drug withdrawal, may represent a subtle and largely unnoticeable confound in preclinical models, if homologous effects occur in animals.
3.2 Psychological Effects of Psychostimulant Withdrawal
One of the predictions of the opponent-process theory is that, with repeated drug exposure, counteradaptations occur that oppose the primary rewarding effects of the drug. Most experimental work is consistent with this hypothesis in that withdrawal from psychostimulant drugs produces a cluster of distinct psychological effects that contrast acute reward. A seminal paper by Gawin and Kleber (35) described, in detail, the abstinence symptomatology of chronic cocaine users, and determined that the course of the post-binge withdrawal syndrome was categorized into three distinct phases. The first of these, referred to as the “crash”, was characterized by “extreme dysphoria…full anhedonia…irritability, anxiety (and) a subjective sense of confusion,” as well as “temporary suicidal ideation” in 43% of the sample patient group. The initial crash phase lasted for up to 4 days, after which subjects entered the “withdrawal” phase, which was represented by milder dysphoria, substantial anhedonia, and anergia, with increased anxiety and irritability. This “withdrawal” phase continued for up to 10 weeks, whereupon subjects gradually entered the “extinction” phase, which reflected a return to prior functioning. More recent studies have provided relatively little support for the multiphase sequence that Gawin and Kleber originally described, rather noting a gradual, linear decrease in the severity of withdrawal symptoms. However, the types of symptoms that Gawin and Kleber initially reported have remained consistent. When viewed in totality, these combined symptoms bear a striking similarity to those of major depressive disorder (MDD). This interesting similarity creates a more structured framework for describing the effects of psychostimulant withdrawal, and may also help us to understand better the neurobiological basis of drug withdrawal. The strong similarities between both disorders have resulted in the widespread assessment of psychostimulant withdrawal symptoms with psychiatric diagnostic tools that are most commonly used to measure MDD, such as the Hamilton Rating Scale for Depression and Beck Depression Inventory (6).
Depressive disorder is diagnosed based upon the presence of a number of symptoms, as defined in the Diagnostic and Statistical Manual of Mental Disorders (DSM-IV) (see Table 1). Symptoms must include the presence of at least one of two core symptoms, which are severely depressed mood and anhedonia – defined as a loss of interest or pleasure in normally rewarding activities. Both depressed mood and anhedonia are prominent and reliably documented during withdrawal from cocaine and amphetamines. Furthermore, nearly all of the remaining seven diagnostic symptoms are widely noted during psychostimulant withdrawal. Appetite changes during psychostimulant withdrawal are frequently reported as a pronounced hyperphagia, which is opposite to the acute anorectic effects of most psychostimulants (36–38). Also, in contrast to the acute effects of psychostimulants on wakefulness, drug withdrawal is associated with hypersomnia (35, 39, 40). Psychomotor retardation typically occurs during psychostimulant withdrawal, opposite to the acute psychomotor agitation during initial exposure to the drug. Similarly, unlike the increased energy that is experienced when first taking psychostimulants, contrary feelings of fatigue are the norm during withdrawal (41, 42). Cognitive impairments reported during psychostimulant abstinence include decreased concentration and confusion (43, 44). Importantly, increased rates of suicidal thoughts and ideations have been noted during psychostimulant withdrawal (45–47). Indeed, to our knowledge, the only DSM-IV symptom of MDD that has not been reported during withdrawal is “feelings of worthlessness or excessive or inappropriate guilt.” We are unsure whether this is due to their absence, or simply because such feelings have not been evaluated.
Table 1
Similarities between the symptoms and behavioral characteristics of major depressive disorder and psychostimulant withdrawal in humans
Major depression | Psychostimulant withdrawal |
---|---|
Behavioral (DSM-IV criteria) | |
Depressed mood and/or irritability | Severely depressed mood and/or irritability |
Diminished interest/pleasure in daily activities | Loss of interest/pleasure in daily activities |
Large increase/decrease in appetite | Increase in appetite |
Insomnia or excessive sleepiness | Excessive sleepiness |
Psychomotor agitation or retardation | Psychomotor retardation |
Fatigue or loss of energy | Fatigue and/or loss of energy |
Diminished ability to think or concentrate | Poor ability to concentrate/confusion |
Feelings of worthlessness and/or guilt | |
Recurrent thoughts of death or suicide | Significant suicidal ideation |
Behavioral (non-diagnostic) | |
Feelings of restlessness | Restlessness |
Comorbid anxiety | High levels of anxiety |
Carbohydrate craving | Increased craving for carbohydrates |
Elevated drug self-administration | Greater drug seeking and taking behaviors |
There are also additional behavioral and affective symptoms shared by both depression and psychostimulant withdrawal that are not diagnostic symptoms of MDD. People with MDD and those in the later stages of psychostimulant withdrawal display increased cravings for drugs of abuse, possibly as a way to self-medicate the dysphoria (for review, see (48)). Both disorders are associated with increased irritability (37, 49), as well as feelings of internal restlessness (35, 50). Additionally, strong cravings for carbohydrates are common during psychostimulant withdrawal and in MDD (47, 51, 52).
4 Effects of Psychostimulant Withdrawal in Animals
It has long been known that drugs that deplete monoamine stores in the brain can induce depressive-like symptoms. The use of monoamine-depleting drugs represents one of the earliest forms of animal models of depression. After the discovery that administration of the antihypertensive drug reserpine (Serpasil) induced the symptoms of clinical depression in approximately 15% of human patients, a large number of experiments were conducted to establish the nature of this phenomenon. As a consequence, the reserpine animal model of depression was established, in which behavioral alterations such as sedation and ptosis, as well as physiological changes such as hypothermia and hyperalgesia, could be induced by injecting rodents with reserpine. However, as noted above, the presence of various confounding somatic symptoms, as well as the relatively poor pharmacological validity of the model, meant that the reserpine model fell out of favor. During the 1980s, important work on the psychological effects of psychostimulant withdrawal indicated that psychostimulants could be used to develop a better model to understand depressive-like symptoms. Much of the preclinical work that has examined both the physiological and psychological effects of psychostimulant withdrawal has therefore been conducted with this goal in mind. Thus, based on the human similarities of psychostimulant withdrawal to MDD, and the application of psychostimulant withdrawal in rodents as a model of depression, the principle focus of many studies has been to examine behavioral and psychological changes that are largely comparable to the symptoms of MDD in humans. For these changes to be relevant, they must exhibit behavioral changes in the animal model that are homologous, or at least analogous, to the symptoms of the disorder: i.e., have “construct validity” (53). Of interest, behavioral changes during a state of psychostimulant withdrawal are not limited to mammals, and have been characterized in species as diverse as zebrafish (54) and planarians (55). However, as the vast majority of studies of psychostimulant withdrawal have focused on rodents, and mainly rats, we limit the current discussion to these species only.
There are a number of clear benefits to studying psychostimulant withdrawal in rodents as opposed to humans. Firstly, it is possible to engage in more invasive techniques to understand better the underlying neurobiology of the condition; while this is not a focus of the present review, it is worth noting that many excellent studies have advanced our understanding of the physiological changes that take place during psychostimulant withdrawal. Secondly, we are able to obtain a “pure” evaluation of the effects of withdrawal from a specific drug, under known conditions of drug administration. Human studies of drug withdrawal are complicated by numerous factors, which include poly-substance abuse and lack of knowledge about drug dosing, not to mention high rates of physical and psychiatric comorbidity. The use of preclinical models provides the opportunity for a relatively unambiguous view of the withdrawal effects under specified and controlled conditions.
4.1 Dysphoria
While there are two core symptoms of MDD, depressed mood and anhedonia, it has so far proven to be a difficult task for behavioral neuroscientists to model the former in preclinical rodent studies of psychostimulant withdrawal. Undoubtedly, this is because the symptom in humans is determined largely by self-report, and unlike most of the other symptoms of MDD, it is difficult to infer from objective assessment or behavioral observation. Clearly, rodents are theoretically unable to tell us how they “feel,” and so we must depend on indirect measures. However, several interesting studies have reported that rodents express higher rates of ultrasonic distress vocalizations during cocaine withdrawal (56, 57); these types of vocalizations are typically emitted when rodents are exposed to highly stressful situations. At least, these vocalizations are consistent with increased fear and anxiety, and could also reflect other negative affective states. It is worth noting that the evaluation of depressed mood in patients does not have to come exclusively from self-reported feelings. In the DSM-IV, it is noted that not all patients with MDD are able or willing to state explicitly any feelings of dysphoria, and in such a case, “the presence of depressed mood can be inferred from the person’s facial expression and demeanor.” Analogously, it has been reported that rats in psychostimulant withdrawal ((58) and personal observations) may exhibit an altered appearance, such as displaying decreased grooming behavior and a huddled stature, that are suggestive of self-neglect and negative affect.
Nevertheless, a number of creative and thoughtful studies have reported findings that are strongly suggestive of, and consistent with, the presence of internal psychological states that resemble depressed mood or dysphoria. It has been shown that rats which were exposed to multiple doses of amphetamine and then tested during drug withdrawal displayed altered behavior in the forced swim test (59). This procedure, also known as the Porsolt test and the behavioral despair test, is a widely used screen that was initially developed to measure the behavioral effects of compounds with antidepressant properties, in which antidepressant drugs increase the time spent active (inversely, decreasing the amount of time spent immobile) in an inescapable container filled with water. The model has a high pharmacological validity, and when tested with the appropriate control for general locomotion, discriminates well against other classes of drugs. More recently, and perhaps controversially, the test has also been used in rodents to detect the presence of depressive-like symptomatology. Although the face validity of the paradigm as a technique to measure depressed mood is questionable, a number of experimental manipulations in rodents that generate depressive-like states in humans also increase immobility in these tasks (60, 61). Rats tested during amphetamine withdrawal displayed decreased time in active behaviors and more time immobile, which the authors interpreted as “depressive-like” behavior. Similar effects in the same series of studies were also found for mice, which exhibited increased immobility in the tail suspension test. Increased immobility in the forced swim test was also observed following a sub-chronic regimen of thrice-daily cocaine treatment in rats (62). A separate group has reported increased immobility by rats in the forced swim test when tested 9–12 weeks after treatment with the psychostimulant drug 3,4-methylenedioxymethamphetamine (MDMA, “Ecstasy”) (63, 64), although this time frame would appear longer than the effects of withdrawal that are typically observed in rodents.
Using a sophisticated drug discrimination paradigm in which rats were trained to respond on specific levers to identify distinct interoceptive states, rats in amphetamine withdrawal would generalize the condition to treatment with a low dose of the typical antipsychotic drug haloperidol (65). Low doses of haloperidol in normal controls has been described as an extremely dysphoric and unpleasant experience (66), implying that the rodents were able to generalize between this state and that of amphetamine withdrawal. While dysphoria and depressed mood are not equivalent, they are both common to psychostimulant withdrawal in humans. Additional evidence of dysphoria in rodents during drug withdrawal comes from studies that have used the conditioned place preference/avoidance procedure. Several studies have reported that rats will quickly learn the association between an environment paired with cocaine, so that they will freely spend more time in that environment. These same animals will rapidly avoid an environment paired with cocaine withdrawal (67, 68), implying a negative affective state.
We have previously reported that animals tested during withdrawal from a binge-like regimen of d-amphetamine display altered behavior in a successive negative contrast paradigm (69) (see Fig. 3). In this protocol, rats are trained reliably to anticipate a reward of a consistent value; in this particular instance, rats were freely able to consume a 32% sucrose solution for a limited time. If this reward is unexpectedly replaced by one of lesser value (a 4% sucrose solution), animals will normally consume lower levels of the new reward than subjects that have only ever been exposed to this lesser reward will. This phenomenon of successive negative contrast has been widely demonstrated across different species, including rodents, primates, and humans. Multiple explanations have been provided to account for the expression of successive negative contrast, many of which are based on the generation of negative affective states in the animals. Disappointment and frustration are prominent amongst these affect-based theories, which posit that the animal develops such negative feelings as it fails to find the reward that it had predicted to receive and instead finds one of a lesser value. In our study, the rats that were “downshifted” during amphetamine withdrawal exhibited a greater degree of negative contrast, and for a longer duration, than those not in withdrawal, possibly reflecting a greater negative state (70). These data are also consistent with anhedonia (see below), with a decrease in the incentive salience of the lesser reward compared to its salience in unshifted animals, as downshifted animals not only consume less of the reward but also decrease their approach speed toward it.
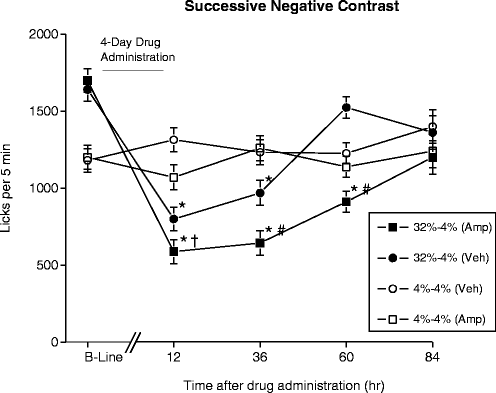
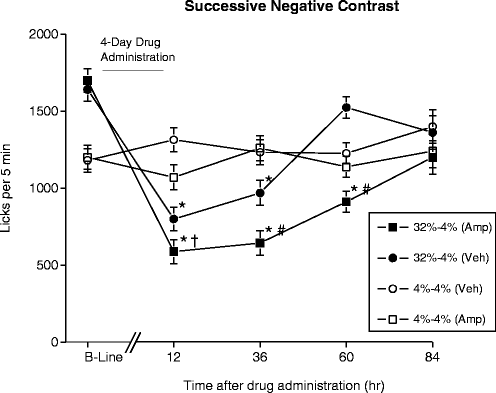
Fig. 3.
Effects of withdrawal from a 4-day regimen of d-amphetamine or vehicle on the number of licks for a sucrose solution. Rats were given 5 min fluid consumption tests at different time points before (B-Line) and after withdrawal from drug administration. Before withdrawal, animals had access to either 4% or 32% sucrose solutions; after withdrawal, all animals had access to a 4% solution. * = significantly different from 4% to 4% (VEH) group, p < 0.05; † = significantly different from 32% to 4% (VEH) group, p < 0.10; # = significantly different from 32% to 4% (VEH) group, p < 0.05.
4.2 Anhedonia
The second core symptom of MDD, anhedonia, is characterized by a decrease or absence of pleasure or interest in normally rewarding activities. As may be expected, it has proven to be significantly easier to model this symptom in rodents, as it is relatively straightforward to train them to respond for a wide variety of both natural and artificial rewards, and also to quantify the interest in such rewards. We have previously reported our findings that amphetamine withdrawal in rats was associated with reduced interest in a sexually receptive conspecific (71), as well as reduced motivation to obtain a sweet sucrose solution (72).
In the former study, d-amphetamine withdrawal was evaluated by using male rats that were well-trained and exhibited high levels of sexual activity before the drug treatment conditions were implemented, thereby maximizing our opportunity to see reductions in motivated responding. Bi-level chambers were used during training in which male rats had once weekly access to females; these chambers effectively create a perimeter “racetrack” area around which the female rat can pace sexual behavior, allowing for more naturalistic mating behavior. An additional advantage to this design is that, with training, male rats engage in anticipatory searches between the levels prior to the introduction of the female, providing a reliable index of sexual interest before copulation begins. Thus, we were able to measure both appetitive and consummatory components of sexual behavior (see Fig. 4). Following extended training, the male rats were tested for sexual behavior during amphetamine withdrawal. Twelve hours after the administration of a 4-day escalating-dose schedule of d-amphetamine, anticipatory locomotor activity, measured as the side changes in the bi-level chamber, in the 5-min period prior to the presentation of an estrous female was reduced, reflecting a decrease in appetitive search behaviors. Furthermore, post ejaculatory intervals were significantly longer in d-amphetamine-treated rats, demonstrating a reduction in an additional component of motivated sexual behavior. These effects were replicated within the same experiment after the animals were exposed to the same drug schedule a second time, and similar results were obtained. We also noted strong trends toward fewer mounts and intromissions in d-amphetamine-treated rats during the period of drug withdrawal. The results of this study indicated that withdrawal from an escalating dose schedule of d-amphetamine decreases certain appetitive components of sexual behavior in sexually experienced male rats, but leaves their copulatory behaviors fundamentally unaltered. This could be interpreted as evidence for anhedonia, as interest is decreased, but the physical capacity to consummate the “reward” is unaltered.
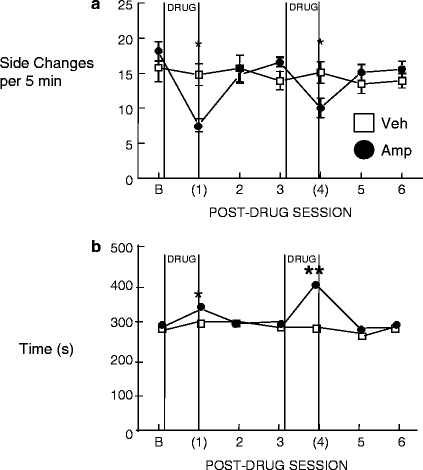
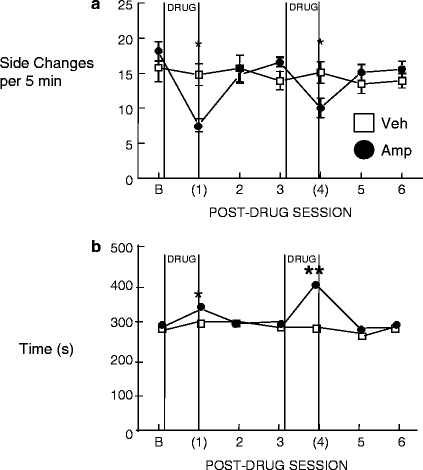
Fig. 4.
Effect of withdrawal from a 4-day escalating regimen of d-amphetamine or vehicle on male sexual behavior. Tests occurred every 5 days with the parentheses indicating a test conducted 12 h after withdrawal from the drug regimen. (a) Anticipatory approaches to the female across sessions. (b) Post-ejaculatory intervals across testing. Stars indicate a significant difference between groups (*p < 0.05, **p < 0.005).
In our second study, we were interested in evaluating how d-amphetamine withdrawal affected the motivation to obtain a rewarding sucrose solution. To quantify this, rats were trained to lever press for a sucrose solution under a progressive ratio schedule of reinforcement. Based on the requirements of the progressive ratio schedule, animals increase the number of lever presses for each subsequent reward (0.5 mL of a 4% sucrose solution), until a point was reached at which they failed to obtain the next reward within the time available: a measure known as the “breaking point” (73–76). After rats had been trained to respond stably for this reward, they were subjected to the 4-day escalating dose schedule of d-amphetamine, and then tested at different time points during drug withdrawal. Rats that were in amphetamine withdrawal exhibited lower breaking points than control animals for at least 72 h after drug termination when responding for the 4% sucrose solution under the progressive ratio schedule (see Fig. 5) (72, 77). This is strong evidence that amphetamine withdrawal induces anhedonia, as the rats displayed reduced motivation to obtain the natural reward. After conducting a more detailed analysis of the data, we noted that both drug-treated and control animals met the required number of responses in approximately the same amount of time at lower ratios of responding. By contrast, the rats in d-amphetamine withdrawal took significantly longer than controls to complete the requirements at higher response ratios, when the amount of effort necessary to obtain a fixed reward was substantially greater. It is not likely that this is due to psychomotor fatigue, as rats in drug withdrawal can exhibit extremely high rates of responding for rewarding electrical brain stimulation (see below) at a much greater rate than the current study. We therefore interpret these data as evidence that motivation to obtain the reward increasingly declines as the demands of the task become greater, or alternately as the ratio of the reward to the effort cost decreases. Thus, anhedonia is manifested more clearly under conditions where greater motivation is required. In support of this hypothesis, we noted that animals in d-amphetamine withdrawal did not differ in their consumption of the sucrose solution when it was freely available.
< div class='tao-gold-member'>
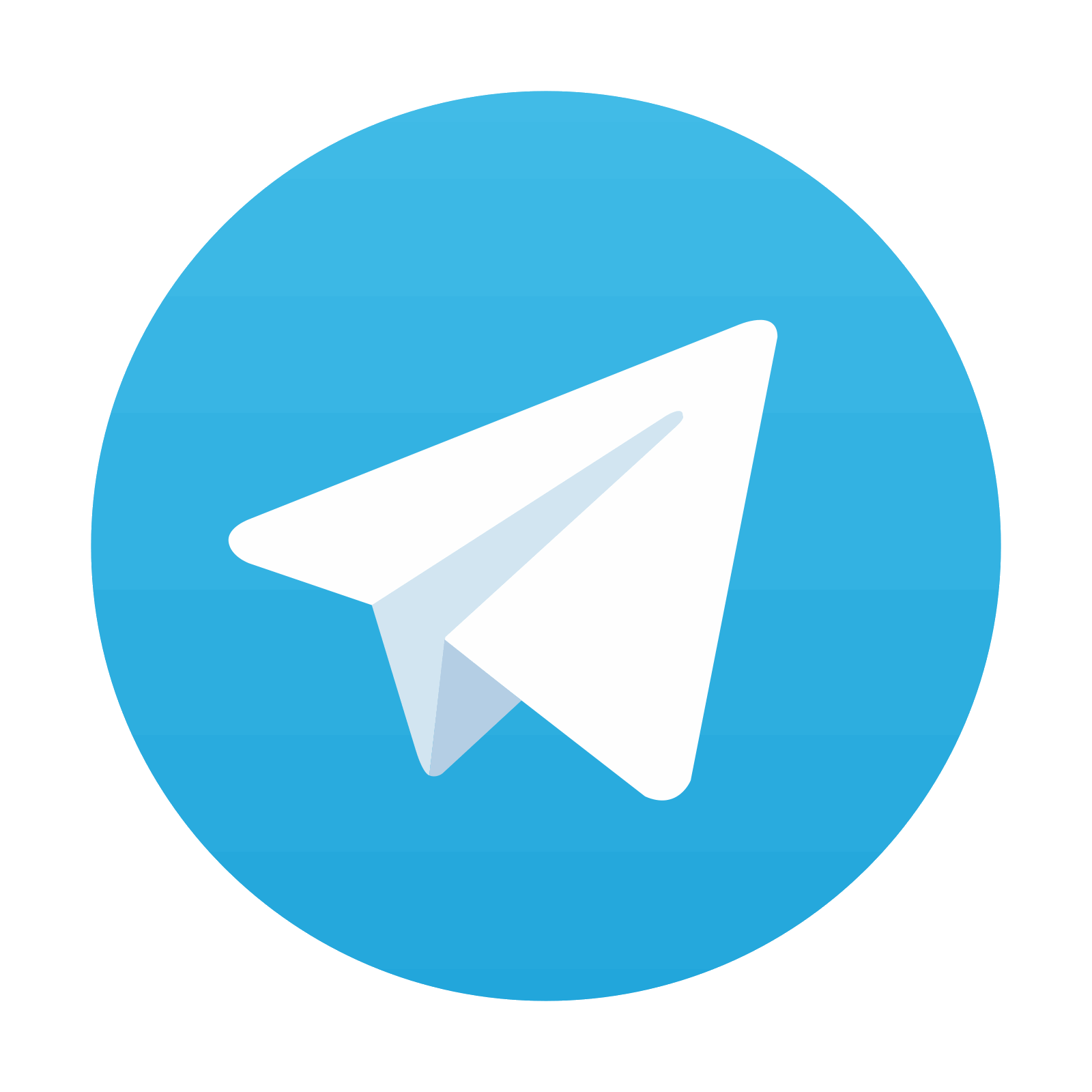
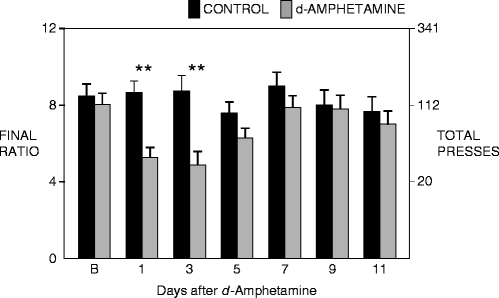
Only gold members can continue reading. Log In or Register a > to continue
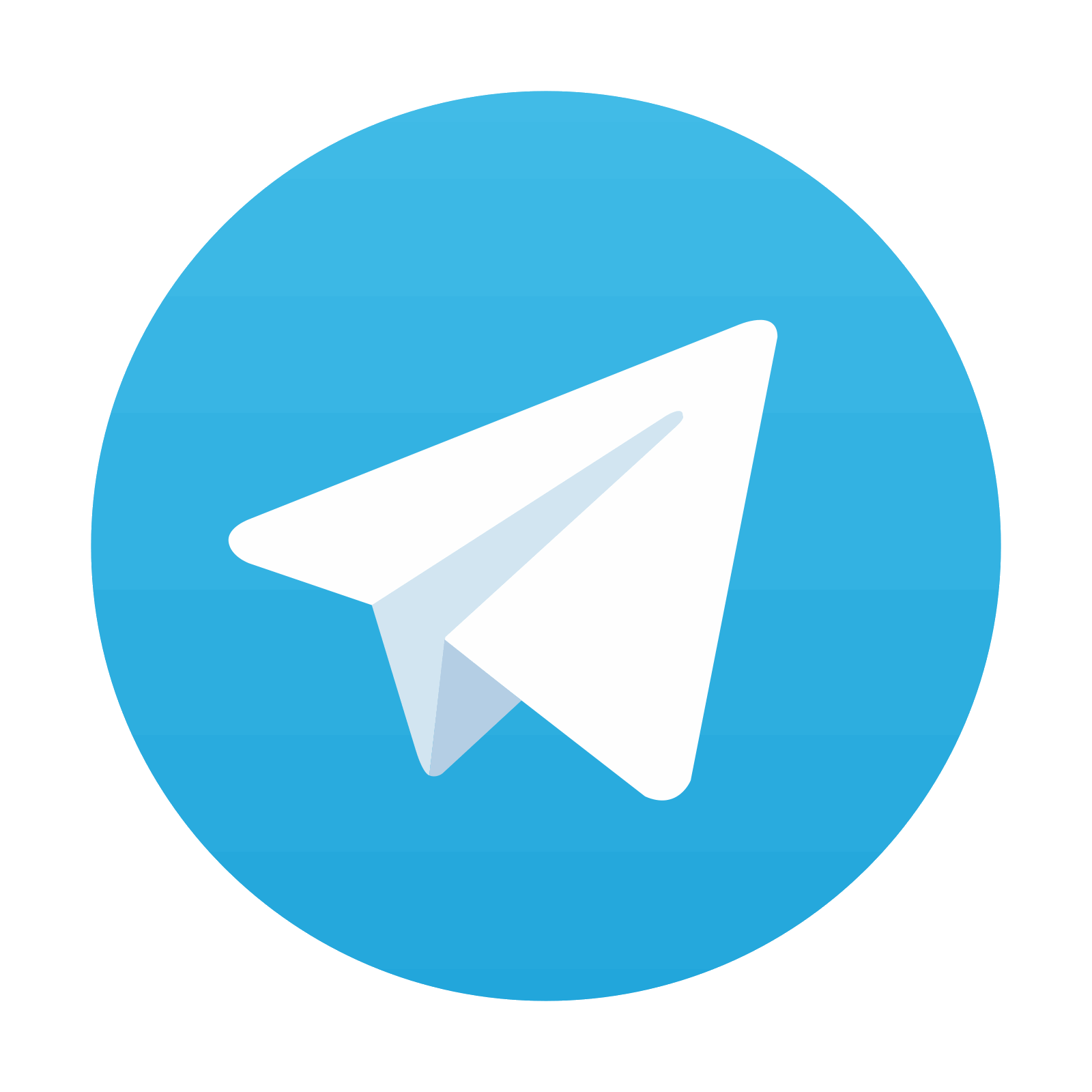
Stay updated, free articles. Join our Telegram channel
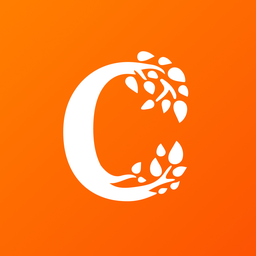
Full access? Get Clinical Tree
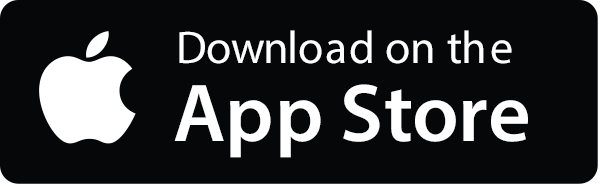
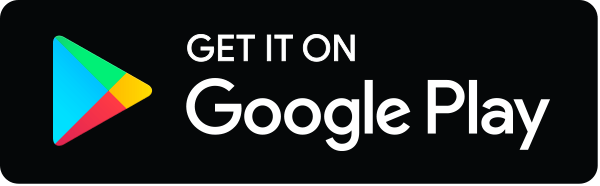
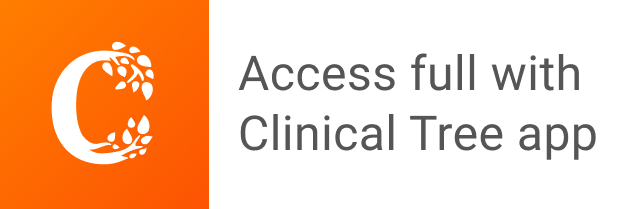