Chapter 23 Vitamins I. INTRODUCTION AND BRIEF HISTORY II. DEFINITION, GENERAL PROPERTIES, AND OVERVIEW OF FUNCTIONS A. Vitamins Important to Redox: Ascorbic Acid, Niacin, and Riboflavin C. Vitamins Involved in Single-Carbon Metabolism: Biotin, Folic Acid, and B12 B. Other Vitamin-Like Compounds The concept that food components are linked to tissue growth and repair was evident in the writings of Greek philosophers as early as the fifth century B.C. In human medicine, nutrition is a topic found in the Hippocratic collection. The first disease to be recognized as nutrition related may have been night blindness. An ancient Egyptian medical text, the Papyrusebers (written about 1550–1570 B.C.), prescribed “beef liver, roasted, squeezed, placed against the eye” for various eye diseases, including night blindness. In China, liver applied topically to the eye was also used as a treatment for night blindness. By the mid-1700s, the curative effects of certain foods had been linked to a number of diseases. James Lynn, a physician in England, assembled his classic treatise that fresh fruits and vegetables seemed effective in curing scurvy. By the 1800s, the association of corn to pellagra (niacin deficiency) was made; by the 1900s, Eijkman, a Dutch physician working in Java, reported that consumption of polished rice was related to polyneuritis, associated with the nutrition disease beriberi. These studies are also noteworthy because they are among the first to utilize experimental animals to produce a vitamin deficiency in a controlled setting. However, the concept that specific diseases could be caused by the lack of a dietary component did not evolve until the beginning of the 20th century. Because of the success of Pasteur and the “germ theory,” many diseases now recognized as nutritional in origin were initially attributed to infectious agents. It was widely held that only the gross constituents of the diet (i.e., carbohydrates, protein, fat, and minerals) were needed for complete nourishment. As F. G. Hopkins, one of the founders of nutrition as a science, noted in his 1929 Nobel lecture, “the quantitative character of the data obtained and the attractive circumstance that such data appeared to supply … induced a feeling that knowledge concerning these needs had become highly adequate and was approximating even to finality … and a feeling that knowledge concerning nutrition was adequate and complete” (Hopkins, 1930). Nevertheless, the concept that a small amount of certain factors seemed necessary for optimal growth and development soon evolved (Goldblith and Joslyn, 1964). The pursuit to define the nature of vitamins was first directed at lipid substances that were demonstrated to be essential in the diet of animals. McCollum and Davis at Wisconsin confirmed that butter or egg yolk, but not lard, supplied a lipid soluble factor that was necessary for growth in rats. As a consequence, the first fat-soluble substance with growth promoting properties (designated as vitamin A) was reported in the early 1900s, a time that most considered the beginning of the “age” of vitamin exploration (Goldblith and Joslyn, 1964). FIGURE 23-1 Vitamin absorption. Vitamins in foods are often present as cofactors or in highly modified forms. Pancreatic and intestinal cell-derived enzymes are required to initiate normal uptake in absorption. Nucleosidases, phosphatases, and peptidases are key factors in processing cofactors to vitamins. Transport of given vitamins can be receptor mediated and occur via pericellular-related processes, passive transport (usually at high luminal concentrations), active transport (requires energy), or facilitated processes (requiring a transporter or chaperone). Now there is constant awareness and sensitivity to the possibility of dietary vitamin deficiencies (and excesses). Nutritional deficiencies are not uncommon in animals, particularly animals fed diets of a limited (or restricted) number of dietary ingredients. A number of subsidiary and contributory factors may also lead to vitamin-related diseases. These factors include interference with normal food intake, loss of appetite (anorexia), impaired absorption or utilization, increased excretion, and the presence of antagonists. Stressful physiological states that increase nutrient demands (e.g., lactation) may also perturb the vitamin status of animals. No definition for vitamins is totally satisfactory. Vitamins have been defined as organic substances present in minute amounts in natural foodstuffs that are essential to normal metabolism, the lack of which causes deficiency diseases. This definition, however, is not specific and can apply to a number of compounds derived from the secondary metabolism of amino acids, simple sugars, and fatty acids. Suffice to say that in most mammals they represent essential organic compounds, not easily classified with the macronutrients. Some may be synthesized, but in insufficient amounts to meet normal needs during critical developmental periods. Vitamins can be further classified according to chemical and physical properties, such as whether they are soluble in aqueous solution or lipid solvents. Those vitamins that are soluble in lipid solvents (vitamins A, D, E, and K) are absorbed and transported by conventional lipid transport processes. For water-soluble vitamins, respective solubility coefficients are major factors that dictate the availability and ease of absorption. Within physiological ranges of intake, active processes are usually involved in the absorption of water-soluble vitamins. Although for some, at high concentrations (10 times or more the typical requirements), passive processes may also be involved. In this regard, the diversity and complexity of vitamin metabolism and processing should be appreciated at the onset. Vitamins in foods are often present as cofactors or in highly modified forms. Pancreatic and intestinal cell-derived enzymes are required to initiate normal uptake in absorption. Nucleosidases, phosphatases, and peptidases are key factors in processing cofactors to vitamins (Fig. 23-1). Vitamins serve a broad range of functions. For example, some of the actions of vitamin A and vitamin D are consistent with the actions of steroid hormones; derivatives of vitamin A and also vitamin E can act as signal transduction mediators; vitamin K acts principally as an enzymatic cofactor; vitamin E can act as an agent that scavenges free-radical containing lipids and oxidants, independent of a direct association with an enzyme, although recent information indicates possible roles in cell signaling. Regarding the water-soluble vitamins, most serve as cofactors or co-substrates for enzymes or in cell signaling. TABLE 23-1 Requirements for Selected Water Soluble Vitamins Expressed as mg/1000 kcal or 4.2 Mjoulesaa These varied functions of vitamins have also complicated the development of a simple system of classification or nomenclature. When the vitamins were originally discovered, they were isolated as fractions from selected foods, and as their exact chemical composition was seldom known, a system of letter designations was developed. However, this system became complicated when it was discovered that some functions originally ascribed to vitamins were due to other substances, such as one of the essential amino acids. Consequently, the designation of vitamins by letters was not systematically pursued. Similarly, the lack of chemical composition data resulted in a complex system of expressing dosages as arbitrarily defined units. Regarding requirements, when expressed on an energy basis, vitamin requirements are often of the same order from one species to the next. Some examples are given in Table 23-1. Differences in dietary requirements between species for given vitamins (in contrast to physiological or metabolic requirements) are usually due to presence of unique pathways for their production, degradation, or disposal. Good examples are ascorbic acid and niacin, which cannot be made in some animals and therefore are true vitamins for such animals. Taurine is another example of a nutrient (although not a true vitamin as classically defined) where continual disposal or loss from the body results in a nutritional need, even though taurine can be synthesized. Further, young and growing animals may have a relatively higher nutritional need for some nutrients. Many species during neonatal periods have requirements for certain compounds, which later in life may be sufficiently produced (e.g., choline, carnitine, or inositol). There are also numerous possibilities for deleterious interactions that can have physiological consequences and affect given requirements (Committee on Animal Nutrition, 2001a, 2001b; McDonell, 2001; Rucker and Steinberg, 2002; Subcommittee on Laboratory Animal Nutrition, Board on Agriculture, National Research Council, 1995). Observations by Hopkins, Stepp, and others that a growth-stimulating factor could be extracted from milk by means of lipid solvents, concentrated, and tested in experimental animal models were seminal steps that eventually led to the identification of vitamin A (Goldblith and Joslyn, 1964). This growth-promoting factor was also described as being present in egg yolk, butter, and cod liver oil. In later studies, “Factor A” or vitamin A was shown to be present as lipid esters in animal tissues and in the form of a “provitamin A” in plants (e.g., compounds in the carotenoid family). The structures and recommended names of naturally occurring and commercial forms of vitamin A and carotenoids are shown in Figure 23-2. Once chemical features for the carotenoids and retinoids were resolved in the 1940s and 1950s, studies of their biological function were undertaken and commercial synthesis of vitamin A and vitamin A-like molecules proceeded rapidly. Carotenoids comprise a group of more than 700 compounds (most often red, yellow, and orange pigments in their isolated states) found in many fruits and vegetables (Stahl and Sies, 2005). To act as a provitamin A, a carotenoid must contain a β-ionone structure (i.e., the ring structure shown in Figure 23-2 containing a single double bond and three methyl groups). The carotenoids represent an unusual class of biological pigments. Carotenoids are rich in conjugated double bonds and are designed to interact with light. Green plants are the main sources of carotenoids in the diet of most animals. FIGURE 23-2 Structures of retinoids and carotenoids related to vitamin A. The structures are for (A) retinol, (B) retinal or retinaldehyde, (C) retinoic acid [all-trans], (D) retinoic acid [11-cis], (E) retinoic acid [13-cis], (F) retinyl ester [palmitate], (G) retinoyl β-glucuronide, and (H) β-carotene. β-carotene is a precursor to retinal, which in turn may be reduced to retinol or irreversibly oxidized to retinoic acid. In animal cells, retinol is “stored” as retinyl ester. In plants and prokaryotes, carotenoids serve as mediators of photo-energy-related processes by capturing energy from light (Stahl and Sies, 2005). Carotenoids are also readily destroyed by intense light, particularly UV light. From a chemical perspective, this is important given the wide range of functions involving carotenoids and vitamin A. Carotenoids can also quench singlet oxygen and may act as both antioxidants and prooxidants. The resulting products of such reactions may also have unwanted side effects, a problem that is not often appreciated. When hays are stored for long periods (e.g., a year or more), the carotenoid content may be markedly reduced or modified because of chemical or physical (UV light) oxidation. Moreover, in plants, carotenoids occur in association with chloroplasts complexed with protein and other lipids and provide the main source of provitamin A for animals. In nonruminant animals, poor digestion of complex organelle structures, such as chloroplasts, in turn may lead to poor digestibility of carotenoid components. Grains, with some exceptions (e.g., corn) are minor sources of provitamin A. Among the legume grains, chickpeas, green and black grams are the best sources of provitamin A. The richest source of carotenoid is red palm oil, which contains 500μg of mixed α– and β-carotene per milliliter. Of the carotenoids, six are known to be biologically important: α-carotene, lycopene, lutein, zeaxanthin, cryptoxanthin, and β-carotene (e.g., because of its role as a precursor to vitamin A). The structures of lycopene, lutein, and zeaxanthin are shown in Figure 23-3. The following sections focus mostly on β-carotene and vitamin A followed by short descriptions for the other carotenoids, which serve as important biofactors, although with no specific or known vitamin functions. FIGURE 23-3 Structures of carotenoids without vitamin A activity. Lutein is found in green leafy vegetables and is employed as an antioxidant and for blue light absorption. Lutein covalently bound to one or more fatty acids is present in some fruits and flowers, notably marigolds. As a pigment, lutein and other xanthophylls (e.g., zeaxanthin) are used as natural colorants (e.g., in chicken feed to provide the yellow skin color). Lutein is also found to be present in a concentrated area of the macula, a small area of the retina responsible for central vision. As a consequence, there is interest in lutein and diseases of the eye, such as age-related macular degeneration. Lycopene is a bright red carotenoid pigment found in tomatoes and other red fruits. Following ingestion, retinyl esters in animal products are hydrolyzed to retinol by pancreatic hydrolases (esterases) or lipid hydrolases localized on the surface of the brush border of intestinal cells (Harrison, 2005). Bile and dietary lipids facilitate the absorption process, as retinyl esters must be a part of a lipid micelle to be absorbed. The micellar structures enhance fusion into the microvillus of intestinal cells. Similarly, lipid micelles enhance the uptake of carotenoids into intestinal cells. The bioavailability and digestion of vitamin A and carotenoids are affected by the overall nutritional status and the integrity of the intestinal microvillus. Absorption of physiological doses of vitamin A in most animals is 70% to 90%, but the efficiency of absorption for carotenoids added to diets is 40% to 60%, depending on the type of carotenoid. Carotenoids contained in plant chloroplasts, however, are often poorly absorbed (less than 10%), because of the low digestibility of chloroplasts and release of carotenoids. FIGURE 23-4 Absorption and cellular metabolism of carotenoids and retinoids. In the intestinal mucosal cell, some carotenoids are oxidized to both carotenals and retinals. Retinal can be reduced by alcohol dehydrogenases (RolDH) to retinol and re-esterified by lethichin retinol acyl transferase (LRAT). Retinol and associated esters are then incorporated into chylomicra, which are released into the lymph. Retinol can also be released from retinyl esters by action of retinyl ester hydrolase (REH). Moreover, retinol can be oxidized to retinal by short-chain dehydrogenases/reductases (SDR). Retinoic acid is formed from retinal by the action of retinal dehydrogenase (RalDH). Retinoic acid is sufficiently polar so that movement is directed to plasma. In contrast, owing to their nonpolar nature, given carotenoid pigments and retinyl esters are partitioned into chylomicrons for delivery into lymph. Retinol transport and carotenoid transport differ. ROL enters intestinal cells by diffusion and effluxes in part by a basolateral transporter in the ABCA1 transport family of protein transporters. Carotenoid uptake is mediated by the apical transporter SR-B1, and carotenoid efflux occurs exclusively via secretion in CM. In the intestinal mucosal cell, some carotenoids are oxidized to both carotenals and retinals (Fig. 23-4). Most of the retinal is next reduced by alcohol dehydrogenases to retinol and re-esterified. Retinol and associated esters are then incorporated into chylomicra, which are released into the lymph. Chylomicra particles in lymph are carried to the liver where about 75% of the retinol-derived products are cleared in most animals. In liver, there is active exchange of retinyl and other retinoids between stellate (also known as Ito cells) and parenchyma cells. To buffer such cells from an excess of vitamin A, it may be “stored” in lipid vacuoles. The storage form is as retinyl esters, where retinyl palmitate is usually the most predominant form. As the body needs vitamin A, retinyl ester in the liver is hydrolyzed and released as retinol bound to retinol-binding protein (RBP), which binds one molecule of vitamin A as retinol per molecule of RBP (Fig. 23-5). When released into circulation, RBP exists as a complex not only with vitamin A, but also with another protein, transthyretin, which binds thyroxin. The RBP and the transthyretin complex transport not only vitamin A, but also thyroxin to targeted cells. The primary target cells for vitamin A are epithelial in nature (e.g., fetal epidermal cells, the cells of the gastrointestinal mucosa, the reproductive tract, pulmonary secretary cells, and the salivary gland) (Debier and Larondelle, 2005; Harrison, 2005). In regard to uptake and entry into targeted cells, such as epithelial cells, the exact role of RBP at the cell surface is unclear. The association constant (Ka) between retinol and the transthyretin-RBP complex is relatively low, approximately 106 M/l. For example, this association constant is about the same as that for the binding of retinol to other proteins (e.g., albumin), which does not imply a high degree of specificity. In cell cultures, RBP is not essential for retinol uptake. However, the interaction of retinol within RBP’s hydrophobic binding domain protects retinal from oxidation. The complex is also not cleared by the kidney, which helps to sustain retinol levels in circulation (Harrison, 2005). Inside the targeted cells, vitamin A, as retinol, interacts with cellular-binding proteins that function to control its subsequent metabolism (e.g., oxidation to retinal and to retinoic acid). Retinal metabolites do not exist “free” in cells but are associated with specific binding proteins. The binding to and release from such proteins is rapid. Because the binding proteins are contiguously associated as a part of the cellular scaffolding, it is possible for given retinoid metabolites to move vectorially along given paths to specific locations in the cell. FIGURE 23-5 Steps in vitamin A processing in liver Stellate and parenchymal cells. Retinoids and carotenoids are transported from the intestine in chylomicron particles and are cleared primarily by the liver. The stellate cell is designed to sequester lipid-like compounds until needed. Fluids in the liver sinusoids derived from blood and lymph bathe stellate cells. The stellate cells are in communication with liver parenchymal cells. As the body needs vitamin A, retinyl esters and β-carotene sequestered in lipid vacuoles are released and eventually converted to retinol. The next steps involve the binding of vitamin A to retinol-binding protein (RBP). When released into circulation, RBP exists as a complex not only with vitamin A but also with another protein, transthyretin, which binds thyroxin. The RBP and the transthyretin complex transport not only vitamin A but also thyroxins to targeted cells. The primary target cells for vitamin A are epithelial in nature (e.g., fetal epidermal cells, the cells of the gastrointestinal mucosa, reproductive tract, pulmonary secretory cells, and salivary gland). Regarding the retinoid metabolites, retinoic acid is the most important, serving as a ligand for nuclear receptors (Velazquez and Fernendez-Mejia, 2004; Velazquez et al., 2005). These receptors are a part of a family of transcription factors that include nuclear receptors that also interact with glucorticoids, thyroxin, and the so-called peroxisomal proliferation activator agonists or ligands. Retinoic acid influences the transcriptional regulation of at least 600 known genes. Both excesses and deficiencies of vitamin A can markedly influence the expression. The catabolism of excess retinal may be initiated by one of several alcohol dehydrogenase isozymes with subsequent oxidation via peroxisomal enzymes. Microsomal enzymes (cytochrome P450 hydroxylases) are also involved. Examples of some of some of the events and products are given in Figure 23-6. Important interactions involve agents that can induce cytochrome P450 hydroxylases (or monooxygenases). For example, phenobarbital can cause depletion of liver retinol by induction of a microsomal oxidase system that promotes retinoid oxidation. The major roles of vitamin A are in cellar differentiation, tissue growth, and vision. In vision, vitamin A, as a component of rhodopsin, facilitates the efficient transfer of energy from photons of light to electrochemical signals. The series of events leading up the propagation of this signal are as follows: vitamin A as 11-cis retinal, forms a protonated Schiff base by binding to a lysine residue in the protein opsin to yield the visual pigment, rhodopsin (Lamb and Pugh, 2004). When a photon of light strikes rhodopsin, cis–trans isomerization occurs and the process results in a highly strained form of rhodopsin, bathorhodopsin, which is converted to metarhodopsin with subsequent deprotonation (Jang et al., 2000). The deprotonated metarhodopsin interacts with transducin, one of the proteins in the transmembrane G-protein family. This interaction causes a subunit of transducin to bind GTP and stimulate cGMP phosphodiesterase activity. This results in a decrease in cGMP, which constitutes a significant amplification of the initiating event, the conversion of light-derived energy through 11-cis to transisomerization of retinal and specific changes in protein conformation (Fig. 23-7). Next, the local change in cGMP concentration results in changes in cation flux (Na and Ca ions) across rod cell membranes (Lamb and Pugh, 2004; McCabe et al., 2004). This initiates an electrochemical event, the firing of cells of the optic nerve. Further, metarhodopsin is phosphorylated during these final steps and interacts with a protein designated as arrestin. The metarhodopsin-arrestin complex inhibits the transducin response and causes the release of all-trans retinal and the return to rhodopsin (opsin), thus completing the cycle. FIGURE 23-6 Steps in the metabolic conversion of vitamin A. The catabolism of excess retinol/retinal may be initiated by one of several alcohol dehydrogenase isozymes with subsequent oxidation via peroxisomal enzymes. Microsomal enzymes (cytochrome P450 hydroxylases) are also involved. Shown are some of some of the events and products. Some of these products may become sufficiently oxidized so that they are excreted by the kidney. Others, such as the glucuronide, are deposed by transport and eventual delivery into bile. In quantitative terms, only a small fraction of the total vitamin A requirement is involved in the visual process because of extensive recycling of retinal. With vitamin A deficiency, there is an inability to appropriately saturate opsin with 11-cis retinal to form rhodopsin and its subsequent complexes. This decreases the sensitivity of the visual apparatus, so that light of low intensity is not perceived leading to nyctalopia or night blindness. An important note, which underscores the importance of having some knowledge of vitamin A chemistry and physiology, is that night blindness is not uncommon in cattle or sheep that have been grazing on dry weathered pasture for long periods such as during prolonged drought (Barnett et al., 1970; Booth et al., 1987). Although most cattle in feedlots are supplemented with vitamin A, if vitamin A is accidentally left out of the ration and stored hay is fed that has lost its carotene content or grain (other than yellow corn), night blindness can occur. As work on vitamin A progressed, it became appreciated that although retinol and retinal were important to vision, the retinoic acid would not correct night blindness but was essential to growth and normal development (Debier and Larondelle, 2005). Within cells all-trans retinol associates with cytosolic retinol-specific binding proteins, and the resulting complex become vehicles for subsequent processing. For example, all-trans retinol may be oxidized and isomerized to all-trans, 9-cis, or 13-cis retinoic acid, which subsequently binds to retinoic acid-specific binding proteins that act as transcription factors in protein regulation and cellar differentiation (Fig. 23-8). The details of such interactions are beyond the scope of this chapter. However, it is important to appreciate that in response to very low doses of retinoids, epithelial cells undergo a “terminal differentiation.” Epithelial cells lose their normal columnar shape, become flattened or squamous, and increase their cytosolic content of keratin (stabilized by transglutaminase catalyzed cross-links). FIGURE 23-7 Vitamin A and vision. Retinal, as a component of rhodopsin, facilitates the transfer of energy from photons of light to electrochemical signals. The series of events includes the cis-trans isomerization, which results in a highly strained form of rhodopsin that is converted to metarhodopsin with subsequent deprotonation. The deprotonated metarhodopsin interacts with transducin, one of the proteins in the transmembrane G-protein family. This interaction stimulates cGMP phosphodiesterase activity, which results in a decrease in cGMP and signal amplification. The local changes in cGMP concentration result in turn in changes in cation flux (Na and Ca ions) across rod cell membranes to initiate firing of cells of the optic nerve. FIGURE 23-8 Vitamin A and cell regulation. Within cells all-trans retinol associates with cytosolic retinol-specific binding proteins, and the resulting complex become vehicles for subsequent processing. For example, all-trans retinol may be oxidized and isomerized to all-trans, 9-cis, or 13-cis retinoic acid, which subsequently binds to retinoic acid-specific binding proteins that act as transcription factors in protein regulation and cellular differentiation. The nature of the metabolic control has many facets including the regulation of specific receptor concentrations and their combination to form specific signaling complexes. The figure shows duplexes for the retinoic acid receptor (RAR) with (1) an isomeric form, (2) complexes with peroxisomal activation receptors, and (3) receptors under the control of vitamin D derivatives. For any given animal, the requirement for vitamin A depends on age, sex, rate of growth, and reproductive status. For optimal maintenance, the allowance for many animals ranges from 100 to 200 international units per kilogram of body weight per day (one international unit is equal to 0.3μg of retinol). In young growing animals, a more precise method of expressing the vitamin A requirement is on an energetic basis. In animal feeds, 4000 to 10,000 international units per kilogram of feed is considered adequate in the United States to provide vitamin A requirements for most animals. Pathological conditions that influence vitamin A status include malabsorption, including pancreatic insufficiency and cholestatic disease, cystic fibrosis, liver disease, and kidney disease. Many forms of liver disease interfere with the production or release of RBP, which results in a lower plasma level of vitamin A. Renal failure can result in loss of RBP in urine. Factors that impair lipid absorption and transport might also be expected to influence vitamin A status. The vitamin A status of animals may be evaluated on the basis of physiological, clinical, and biochemical procedures. Clinical testing for night blindness and the elevation of CSF pressure has been used to indicate vitamin A status. The concentration of retinol and its esters is readily measured in biological samples by HPLC using various detectors and indicates the vitamin A status. As the concentration of retinol in plasma is well maintained until liver reserves are depleted, plasma retinol is not an index of vitamin A reserves. The latter is best provided by analysis of liver biopsy samples. In many carnivores, the plasma contains, in addition to retinol, equal or greater concentrations of retinyl palmitate and retinyl stearate bound to albumin, the immunoglobulin fraction, or to VLDL. Plasma retinol concentrations in excess of 30 μg/dl generally indicate that vitamin A is not limiting. In most species, liver concentrations of 100 μg of retinol/g liver are generally adequate. Vitamin A and various retinoids are used increasingly to treat skin disorders (acne and psoriasis) and certain forms of cancer. A vitamin A responsive dermatosis in cocker spaniels is well recognized and has been previously described (Scott, 1986). Retinyl-β-glucuronide and hydroxyethyl retinamide are commercial preparations of retinoids that have such activity but are less toxic than retinoic acid. The mechanisms by which these agents function most probably relate to the complex pathways involved in epithelial and epidermal cell differentiation. Vitamin toxicities may be classified under three broad categories: acute, chronic, and teratogenic. When a single dose of vitamin A (greater than 100 mg) is injected into animals (20 to 50-kg weight range), symptoms such as nausea, vomiting, increased cerebral spinal fluid pressure, and impaired muscular coordination result. A lethal dose of vitamin A (100 mg) given to young monkeys has been reported to cause coma, convulsions, and eventual respiratory failure. Chronic toxicity may be induced by intakes of vitamin A in amounts 10 times the normal requirements. Doses of vitamin A in this range can lead to alopecia, ataxia, bone and muscle pain, and purities. Although cats have a high tolerance to excessive intakes of vitamin A, hypervitaminosis A occurs in cats that are given a diet largely of liver. Affected cats exhibit skeletal deformations, particularly exostoses of the cervical vertebra, which precludes effective grooming. Vitamin A is also a powerful teratogen. A single large dose during pregnancy (in the 50- to 100-mg range) for an animal weighing 20 to 50 kg can result in fetal malformations. Chronic intakes (exceeding 10 times the requirements for given animals) can also be teratogenic. Carotenoids, unlike retinoids, are generally nontoxic, and many animals routinely ingest gram amounts of carotenoids on a daily basis with no deleterious effects (old world primates, herbivores, etc.). In addition to β-carotene, of the other carotenoids, the most information is available for α-carotene, lycopene, lutein, zeaxanthin, and cryptoxanthin. To reiterate, these carotenoids along with hundreds of others are the natural pigments in plant tissues and give them color (Fig. 23-3). Carotenoid pigments attach themselves to proteins or fats and can produce blue, green, purple, or brown pigments in addition to yellow, orange, and red. If an animal’s skin or feather color comes from carotenoids and it is not available in food, some or all of the color fades. For example, many birds develop bright red, orange, or yellow carotenoid pigmentation that they use presumably to attract mates. Because animals often obtain several different carotenoids from plant and animal food sources, it is possible that these pigments are accumulated at different levels, which results in the ultimate color expression of individual animals. As an example, when finches are fed a lutein-zeaxanthin mix, proportionally more zeaxanthin was found than lutein than occurred in the diet (i.e., there is preferential accumulation in the body). In fish, pigmentation is influenced by diet and sex. Presumably, males absorb/retain more pigments than females. Often consumers of various products (notably egg yolk, eggshell, broiler skin, and salmon flesh) prefer a specific type and degree of coloration. Although some birds can be sexed by visual inspection of their genitalia, mating resulting in sex-associated color phenotypes is becoming more in use. The genetic markers involved affect the color of the plumage and the cloning of genes involved in pigmentation offers the prospect of deciphering the genetic control of animal pigmentation and modifying it to meet specific pigmentation needs (Castaneda et al., 2005; Johnson et al., 2000). Regarding specific carotenoids, α-carotene is one of the most abundant carotenoids in the diet and can be converted to vitamin A, but with only one-half the activity as β-carotene (contains only one β-ionone ring in contrast to two for β-carotene). Other differences in biological activity have also been reported. The α-carotene is a better inhibitor toward certain growth factors (e.g., N-myc activity) than β-carotene. N-myc is in the oncogene family of growth factors. Because of its abundance, α-carotene is also an excellent biomarker of intake of fruits and vegetables (Stahl and Sies, 2005). Another carotenoid, lycopene, is a red pigment found in fruits and vegetables. In human epidemiological studies, its consumption in modest amounts is weakly associated with a reduced risk of certain cancers. Lutein and zeaxanthin are carotenoids found in green, leafy vegetables and algae and have been considered recently for potential benefits to sight and vision, particularly a decrease in the risk of cataracts. Cryptoxanthin has even been reported to decrease bone loss in ovariectomized rodents. Thus, there are a wide range of health effects, which may have nutritionally and pharmacological potential (Stahl and Sies, 2005). Sir Edward Mellanby in 1921 reported the induction of rickets in dogs through dietary manipulation. He discovered that the disease could be corrected with cod liver oil. McCollum in 1922 reported the curative factor in cod liver oil was not vitamin A and appeared to be another fat-soluble substance. This substance was later identified as vitamin D, based on the ability to inactivate the vitamin A factor in cod liver by mild oxidation with the retention of antirachitic activity (Goldblith and Joslyn, 1964). The D vitamins are a family of 9,10-secosteroids that differ only in the structure of the side chain attached to carbon-17. The two forms of vitamin D significant in veterinary medicine are ergocalciferol (vitamin D2) and cholecalciferol (vitamin D3). The differences in the side chain result in the vitamins having disparate potencies with some species of animal and differing in toxicity when consumed in large amounts. These two forms of vitamin D are produced in a two-step reaction when their respective sterols ergocalciferol and 7-dehydrocholesterol absorb ultraviolet radiation and undergo photolysis, which is then followed by thermal isomerization (Fig. 23-9). Excessive ultraviolet radiation of the sterols produces inactive compounds. Under most instances, animals can synthesize sufficient quantities of cholecalciferol if they receive adequate exposure to ultraviolet light of wavelength 280 to 320 nm (Hendy and Goltzman, 2005; Hendy et al., 2006; Xue et al., 2005). This is particularly true when the calcium and phosphorus requirements of the animal are met. As vitamin D is produced at one site and acts at other sites including bone and intestine, it fulfills the definition of a prohormone. In most animals, 7-dehydrocholesterol is abundant in skin, being the ultimate precursor for cholesterol, which is synthesized from acetate. However, the skin of cats and dogs and possibly other carnivores contains only small quantities of 7-dehydrocholesterol, which does not permit adequate synthesis of vitamin D. These animals are solely dependent on the diet for this vitamin. With the exception of animal products, most natural foods contain low vitamin D activity. Fish, in particular saltwater fish, such as sardines, salmon and herring, and fish liver oils contain significant to large quantities of vitamin D. Many plants also contain hydroxylated ergosterol derivatives, some of which have potent vitamin D activities (Wasserman, 1975). Initially, it was speculated that vitamin D might serve as an enzymatic cofactor for reactions that served to maintain calcium and phosphorus (as phosphate). When isotopes of calcium became available, it was soon appreciated that there was considerable lag between the administration of vitamin D and its effect on calcium-related metabolism. This lag was shown to be due to the conversion of vitamin D to an active form. Investigations throughout the 1960s and 1970s led to the sequence of events that is outlined in Figure 23-9. For example, the kidneys were identified as the site of 1,25-dihydroxycholecalciferol (calcitriol or 1,25-(OH)2-D3) production. This discovery, together with the finding that 1,25-(OH)2-D3 was in the nuclei of intestinal cells, suggested that vitamin D was functioning in a manner analogous to that for steroid hormones. The production of calcitriol is normally closely regulated through feedback control and the influence of parathyroid hormone (PTH) on the activities of the 1α- and 24-OH-hydroxylases. A fall in plasma calcium triggers the release of PTH from the parathyroid gland, which stimulates 1α-hydroxylase production and leads to an increase output of calcitriol from the kidney. A separate hydroxylase, which catalyzes 24,25-(OH)2-D3 production, is activated under eucalcemic and hypercalcemic states. Whether 24,25-(OH)2-D3 serves an essential function is controversial. However, there is evidence that 24,25-(OH)2-D3 is required for some of the biological responses attributed to vitamin D (Dusso et al., 2005; Norman et al., 2002). Norman and coworkers (Norman et al., 2002) have shown that hatchability in chickens markedly improves if both 1,25-(OH)2-D3 and 24,25-(OH)2-D3 are administered into eggs containing viable embryos from hens rendered rachitic (vitamin D deficient) before egg production. The two major sites of action of calcitriol in relation to calcium homeostasis are bone, where it acts rapidly in concert with PTH in response to hypocalcemia, and at the intestine, where the response time is longer. In addition to 1,25-(OH)2-D3 and 24,25-(OH)2-D3, more than 20 other hydroxylated intermediates and end products have been identified. Most of these are probably routed into elimination pathways, although some may be potentially functional (e.g., 1,24,25-trihydroxycholecalciferol, which has some vitamin D activity). FIGURE 23-9 Vitamin D metabolism. Vitamin D is formed in the skin of most animals after exposure to ultraviolet radiation. Vitamin D can also come from the diet. It is hydroxylated in the liver to 25-hydroxyvitamin D, and in the kidney to 1,25-dihydroxyvitamin D, which is the active form. The production of 1,25-dihydroxyvitamin D is normally regulated through feedback control and the influence of parathyroid hormone (PTH) on the activities of the 1α-OH or 25-OH-vitamin D hydroxylase. A fall in plasma calcium triggers the release of PTH from the parathyroid gland, which stimulates 1α-hydroxylase production and leads to an increase in output. A separate hydroxylase, which catalyzes 24,25-(OH)2-vitamin D3 production, is activated under eucalcemic and hypercalcemic states. The major sites of action in relation to calcium homeostasis are bone and intestine. The immune system and the pancreas are also sensitive to changes in vitamin D status. Calbindin, a calcium binding protein, is a major product synthesized in intestinal cells in response to calcitriol. Calbindin influences the movement of calcium across the intestinal cell. Binding of calcium to this protein allows the intracellular concentration of calcium to be elevated. The hormone forms of cholecalciferol also stimulate the production of the calcium, sodium-dependent ATPases, which reside on the luminal surface of the intestinal cell. This facilitates the vectorial movement of calcium out of the cell into circulation. In addition, evidence also indicates that 1,25-(OH)2-D3 can stimulate secondary messenger systems (e.g., protein kinase and adenyl cyclase-controlled dependent messenger systems) (Dusso et al., 2005). In addition to intestinal cells, the osteoblasts of bone are another target of vitamin D metabolites and play a major role in short-term calcium homeostasis. In addition, 1,25-(OH)2-D3 is required for normal bone mineralization during skeletal growth and remodeling of bone. Vitamin D receptors (VDR) in bone are located in osteoblasts and progenitor cells of bone and control the synthesis and secretion of a number of bone-specific proteins in osteoblasts such as osteocalcin, osteopontin, collagen, and alkaline phosphatase. The actions of vitamin D metabolites are both direct (e.g., transcriptional regulation via VDR interactions) and indirect (modulation of secondary signaling pathways, e.g., protein kinase C regulated pathways). Although osteocalcin and osteopontin synthesis have been shown to be regulated at the transcriptional level of their respective genes, for the most part vitamin D metabolites attenuate the action of polypeptide hormones, such as PTH or calcitonin, which stimulates bone resorption and accretion, respectively. Of these two processes, maintaining bone resorption is the most important, because under normal conditions, the serum calcium and phosphate ion concentrations are at levels that favor bone apposition or accretion (Dusso et al., 2005). Naturally occurring deficiencies of vitamin D occur in lambs born to ewes not supplemented prepartum with D3 in northern latitudes during the winter months. Vitamin D deficiency also occurs in lambs reared indoors on grain diets (often barley), which do not supply an adequate amount of vitamin D2. Deficiency is frequently manifested as skeletal limb abnormalities. As an unusual and specific example, there are also published reports of vitamin D deficiency in llama offspring (crias) in Oregon during the winter months (Judson and Feakes, 1999; Murray et al., 2001; Van Saun et al., 1996). Vitamin D receptors (VDR) have been found in a large number of cell types, ranging from skeletal muscle to cells important to immune and phagocytic functions (e.g., macrophages). In pancreatic β-cells, 1,25-(OH)2-D3 has also been observed to be important to normal insulin secretion. Vitamin D increases insulin release from isolated perfused pancreatic cells. Moreover, vitamin D metabolites can suppress immunoglobulin production by activated B-lymphocytes. T cells are also affected by vitamin D metabolites; 1,25-(OH)2-D3 exhibits permissive or enhancing effects on T cell suppressor activity. A specific transport protein delivers 1,25-(OH)2-D3 and other active forms of vitamin D to targeted cells. The active form of vitamin D then interacts with receptor proteins, which in turn signals enhanced expression of selected proteins. The vitamin D-binding protein (DBP), also known as group-specific component or Gc-globulin, is a multifunctional plasma protein. DBP is expressed as a single polypeptide chain with a molecular mass of ∼56 kDa and circulates in plasma at 6 to 7 μM. Because of its extensive polymorphism, DBP initially was named the group-specific component of serum, later shortened to Gc-globulin. DBP is a member of the albumin, α-fetoprotein, and α-albumin/afamin gene family. In addition to functioning as a circulating vitamin D transport protein, it has been demonstrated to scavenge G-actin released at sites of necrotic cell death and prevents polymerization of actin in the circulation (Dusso et al., 2005). Most animals require about five micrograms cholecalciferol per 1000 kcal of diet. When intake exceeds five to ten times this amount, there is a risk of toxicity, characterized by hypercalcemia and soft tissue calcification, in particularly the blood vessels of the lung, kidney, and heart. Acute doses of vitamin D (>100 times the requirement) can eventually result in a negative calcium balance, because bone resorption is accelerated. As noted, some plants (e.g., Solanum malacoxylon, Cestrum diurnun, and Trisetum flavescens) contain compounds with vitamin D activity (mostly glycosylated forms of ergocalciferols) and vitamin D intoxication can follow their ingestion. Rodenticides containing cholecalciferol as the active ingredient have resulted in toxicity in companion animals that ingest the bait directly, or carcasses of rodents that have ingested the bait. Naturally occurring toxicity has occurred in cats in Japan given a commercial diet containing large amounts of tuna viscera. Tuna viscera contains extremely high amounts of vitamin D, most of it in the liver. Reliable assays for the measurement of vitamin D, calcidiol, and calcitriol in plasma are available. Calcitriol occurs in picomolar concentrations (normal values 40 to 150 pmol/l or 16 to 60 pg/ml) and has a half-life of about 4 to 6 h in a large (50 to 100 kg) animal. Concentrations of vitamin D in plasma after oral administration are in the nanomolar range (“normal” values range from 0 to 310 nmol/l or 0 to 120 ng/ml). Vitamin D has a half-life of 24 h, so the plasma concentration reflects immediate intake, rather than overall status. In contrast, 25-OH vitamin D has a half-life of about 3 weeks, provides the useful index of vitamin D status, and is the measurement of choice. Plasma concentrations of 25-OH vitamin D of 20 to 150 nmol/l or 8 to 60 ng/ml cover the normal range for most animals. Much higher levels than these have been observed in cats given diets containing high levels of cholecalciferol without apparent deleterious effects (Committee on Animal Nutrition, 2001a, 2001b; Subcommittee on Laboratory Animal Nutrition, Board on Agriculture, National Research Council, 1995). In the early 1920s, Herbert Evans and Kathryn Bishop observed that rats failed to reproduce when fed diets containing rancid lard, unless they were supplemented with lettuce or whole wheat. Later it was found that germ oils, particularly wheat germ oil, contained an active principle that seemed responsible for improving reproductive performance. These early studies provided yet another function for a fat-soluble substance. By the early 1930s, it was recognized that this substance was a factor that differed from vitamin A or vitamin D. The compound was designated as vitamin E by Barnett Sure and later as α-tocopherol from the Greek word “tokos” meaning childbirth or reproduction. By 1940, a number of compounds in the tocopherol family were identified and purified. With elucidation of tocopherol structures and eventual chemical synthesis (Fig. 23-10), studies quickly followed that demonstrated embryonic failure resulted from vitamin E deficiency. Pappenheimer, Olcott, Martill, and others observed that muscle degeneration was also a common deficiency symptom and that vitamin E seemed to function as an antioxidant. Next, other signs and symptoms were identified, including oxidative diathesis and encephalomalacia in chickens. In addition to these signs, liver necrosis and hemolytic anemias were observed in vitamin E-deficient animals (Traber, 2007). The principal sources of tocopherols are plant oils. Tocopherols are unique because they act primarily at a chemical level as antioxidants, although other possible roles in cell signaling have been described. Primarily, vitamin E protects unsaturated fatty acids found in the phospholipids of cell membranes. The quinone moiety of tocopherols is capable of quenching free radicals, such as the free radical of hydrogen (H•), superoxide radicals (O2•–), hydroxyl radicals (OH•), and other lipid-derived radical species (LOO•). Vitamin E in the course of its action is sacrificed in acting as a free-radical scavenger. Vitamin E is very reactive and is in effect sacrificed thus inhibiting the formation of lipid-derived oxidation products (Traber, 2007).
I. INTRODUCTION AND BRIEF HISTORY
II. DEFINITION, GENERAL PROPERTIES, AND OVERVIEW OF FUNCTIONS
III. FAT-SOLUBLE VITAMINS
A. Vitamin A
1. Introduction
2. Proforms of Vitamin A: The Carotenoids
3. Metabolism
4. Functions
5. Growth and Cell Differentiation
6. Requirements
7. Evaluation of Vitamin A Status of Animals
8. Pharmacology and Toxicity
9. Other Carotenoids
B. Vitamin D
1. Introduction
2. Sources, Functions, and Metabolism of Vitamin D
3. Other Functions of Vitamin D
4. Requirements and Toxicity
5. Assessment of Vitamin D Status
C. Vitamin E
1. Introduction
2. Chemistry, Metabolism, and Sources
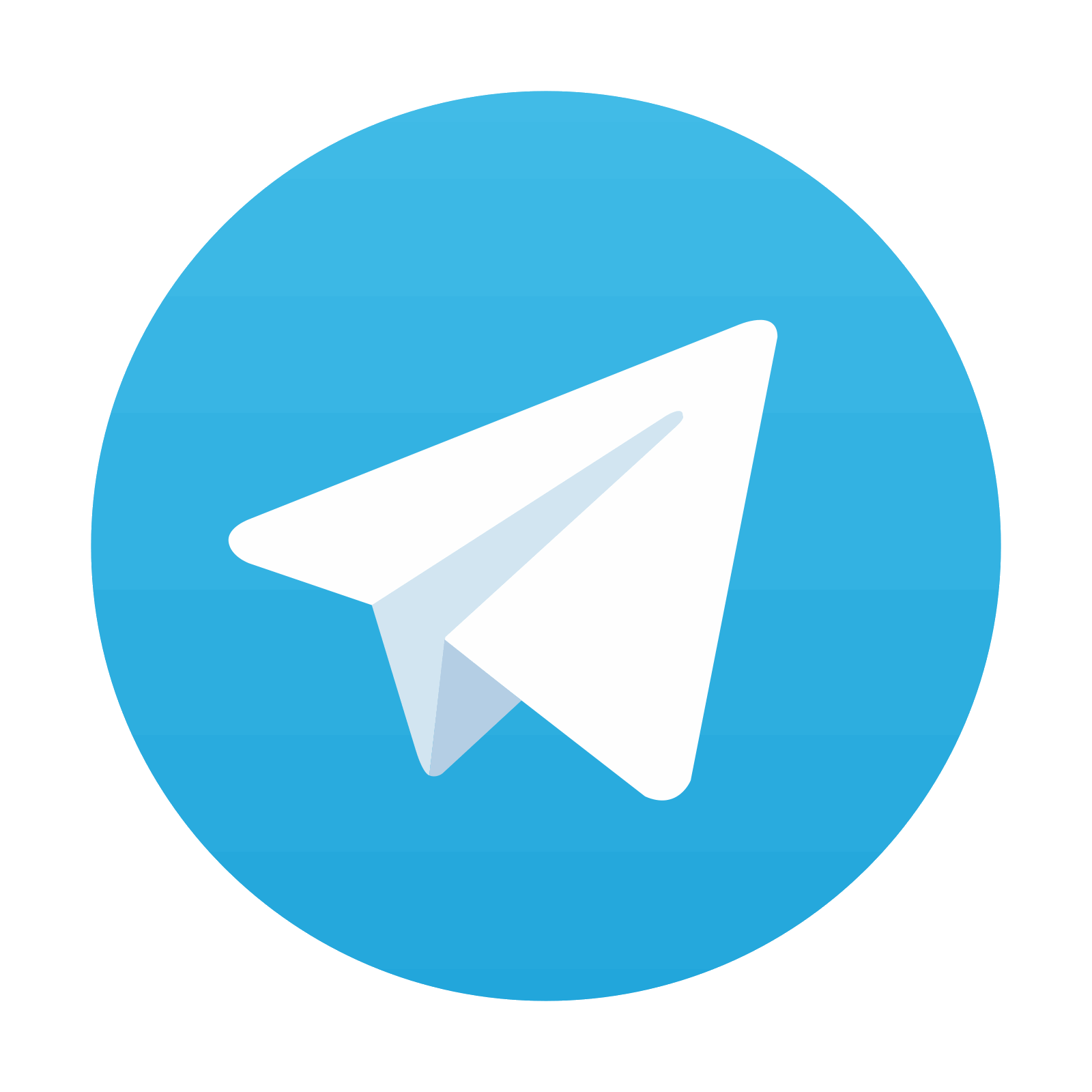
Stay updated, free articles. Join our Telegram channel
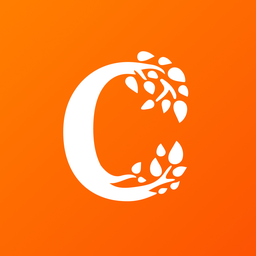
Full access? Get Clinical Tree
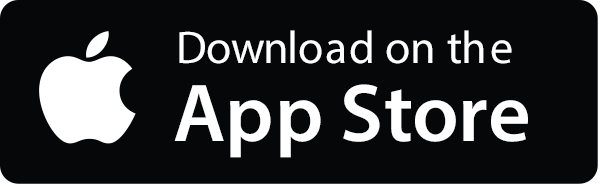
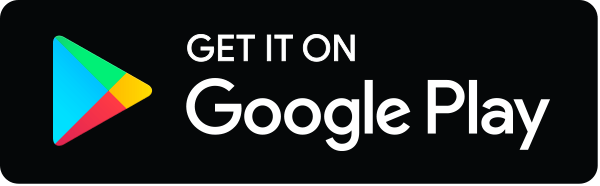