Chapter 12 Diagnostic Enzymology of Domestic II. HISTORY OF CLINICAL ENZYMOLOGY III. FACTORS AFFECTING SERUM ENZYME ACTIVITY A. Organ Mass and Enzyme Tissue Concentration C. Mechanisms of Release of Cytoplasmic Enzymes or Other Protein Biomarkers from Cells to Blood D. Mechanisms of Release of Membrane-Bound Enzymes E. Blood Clearance Rates of Enzymes The detection of proteins in serum by their catalytic activity as a reporter of tissue damage is a cornerstone of medical laboratory analyses (Rej, 1998). Clinical enzymology is the discipline that studies and tests enzyme activity in serum, plasma, urine, or other body fluids for the purpose of helping to establish the diagnosis and prognosis of disease and to screen for abnormal organ function. Although not the subject of this chapter, it should be noted that some enzymes are also of major importance as analytical reagents. This chapter first explores the universal factors affecting changes in enzyme content of bodily fluids and then delves into specific details relevant to particular enzymes. Discussion of basic concepts in enzymology, such as enzyme structure, kinetics, or analysis, is limited to those that are of clinical relevance or that add insight into interpreting changes in body fluid enzyme activity. Additional information regarding enzyme structure and enzyme kinetics can be found in numerous biochemistry texts as well as clinical texts such as Tietz Fundamentals of Clinical Chemistry (Burtis and Ashwood, 2001) and the previous edition of this book. In addition, the methodology of the various enzyme assays can be found in the literature provided by the vendors of enzyme assay reagents. Although the presence of enzymes in cells and plasma was first recognized in the 1800s, the development of clinical enzymology began after the introduction of an assay for serum amylase by Wohlgemuth in 1908 and the report in 1916 that serum amylase activity in blood and urine was a reliable test for pancreatic disorders (Rosenfeld, 1999). This finding was followed in 1927 by the discovery of alkaline phosphatase (ALP) in bone and the description of serum alkaline phosphatase as a diagnostic test (Rosenfeld, 1999). The development and marketing by Sigma Chemical Company in St. Louis in the 1950s of simplified enzyme assays in kit form, such as aspartate and alanine aminotransferases, and an ALP assay that used p-nitrophenylphosphate as substrate (Technical Bulletin 104), were major factors in their routine clinical use and encouraged additional studies in diagnostic enzymology (Berger, 1993; Bessey et al., 1946; Reitman and Frankel, 1957). In addition to assay reagent development, an equally significant contribution to the development of clinical enzymology was the invention by Leonard Skeggs of a multichannel autoanalyzer that was marketed by Technicon in 1964 (Skeggs, 2000). The autoanalyzer increased the availability of reduced cost serum enzyme analyses, which ultimately led to their routine use in both human and veterinary diagnostic medicine. The advancement of clinical enzymology included the development and evaluation of enzyme assays for use in nonhuman animal species, some of which have been found useful, whereas others have been dropped for various reasons. In some cases, the decision to investigate an enzyme for diagnostic use may have related not only to its potential clinical relevance but also to the efficiency of offering the test. In spite of recognition of species differences, veterinary medicine has often followed human medicine in its choice of diagnostic tests. The bias toward enzyme assays used in human medicine is due in part to their availability on automated analyzers, making all tests low-cost, on-demand assays with a high degree of precision and accuracy. The automation of enzyme assays, and the popularity of the serum chemistry profile in veterinary medicine, has allowed retrospective studies to be conducted and has given the veterinarian an opportunity to critically evaluate the diagnostic function of the common assays in a large number of animals on a regular basis, as well as gain a “feel” for the results, thereby allowing for more subtle clinical interpretations. It is likely that diagnostic tests that are not automated are less understood and interpreted in a more rigid manner with less appreciation for nuances and significance of the test result. Interestingly, during the first approximately 30 years of serum enzyme testing in veterinary medicine, these tests were often viewed as “diagnostic” tests, whereas in the past approximately 20 years their variable and often somewhat limited degree of diagnostic specificity has been appreciated and they are now most often recognized as “screening” tests. Although serum enzyme activity is reported as part of numerous studies published in the literature, the number of studies directed primarily at answering specific questions regarding the enzymes appears to have decreased since the late 1990s from what might be considered the heyday of clinical enzymology in the 1960s to mid-1990s. As the field of clinical enzymology has developed, so has our understanding of the physiological factors responsible for the alterations in serum enzyme activity that occur with disease, although several unanswered questions remain. Organ specificity, subcellular location of the enzyme, the mechanism of enzyme release from cells, the clearance from blood, and the rate of induction of enzyme synthesis all affect to a lesser or greater extent the diagnostic accuracy of the various enzyme assays (Hoffmann and Solter, 1989; Solter, 2005). This section discusses the physiological, biochemical, and anatomical factors that affect changes to serum enzyme activity. The roles that enzyme tissue concentration and organ mass play on the magnitude of blood enzyme activity are relatively straightforward. Organs with a high concentration of an enzyme have the potential to cause a greater increase in serum enzyme activity with disease. For example, the intracellular to extracellular concentration gradient of hepatocellular alanine aminotransferase (ALT) is 100,000:1. Injury to hepatocytes, therefore, has the potential of causing markedly increased serum ALT activity. The higher the concentration gradient of the enzyme or protein marker between the cell and the interstitial space, the faster is the translocation of significant quantities of the enzyme to the interstitial space and ultimately the blood (Mair, 1999). Likewise, the liver has a large mass, thereby adding to the potential increase in serum ALT activity. The location of cellular enzymes relative to the blood, urine, or other fluids is an especially significant determinant of whether an increase in enzyme activity will occur with enzyme release and in which fluid it will be found. A well-known example is renal tubular gamma glutamyltransferase (GGT) located on the luminal surface of renal tubular epithelial cells. Injury to these cells results in release of the gamma GT into urine but not into blood. Similarly, alkaline phosphatase (ALP) located on the luminal surface of enterocytes is lost into the gut lumen rather than blood with enterocyte injury. Hepatocellular ALP, however, with activity over both bile canalicular and sinusoidal surfaces, can be increased in both bile and blood. Cytoplasmic enzymes are contained within cell membranes. Healthy plasma membranes are thought to be impermeable to macromolecules such as enzymes. Therefore, alteration in the cell membrane is necessary to allow cytoplasmic enzymes to gain access to the blood. In the event of cell necrosis, perforations and tears of the cell membrane allow the release of cytosolic contents in a relatively straightforward process. However, increases of serum enzymes do not always correlate to the degree of histological evidence of cell necrosis. Hence, it is has been long postulated that under certain circumstances, cytoplasmic enzymes may “leak” from diseased cells that remain viable, perhaps through membrane pores or tears. However, it is difficult to envision that a cell could develop a pore or tear large enough to allow “leakage” of macromolecules such as enzymes while maintaining the intracellular-to-extracellular electrolyte ratios necessary to remain viable. An alternative mechanism by which a cell could sustain damage from which it survives and yet allows the release of cytoplasmic enzymes is the formation of membrane blebs (Coltran et al., 1999; Gores et al., 1990; Lemasters et al., 1983; Mair, 1999). These blebs then are ruptured or are released as vesicles into the blood where they are eventually broken down, releasing their contents, including cytoplasmic enzymes. The body of knowledge supporting this concept has been growing since the 1980s (Gore et al., 1990; Kristensen, 1994; Mair, 1999; Solter, 2005). Cell membrane bleb formation has been recognized following hypoxic insults and likely reflects two sequential developments. Depletion in energy stores in the form of ATP is followed by several events including the influx of calcium into the cell (Coltran et al., 1999). This calcium influx results in activation of intracellular phospholipases, endonucleases, and proteases and ultimately in disruption in the phosphorylation state of cytoskeletal proteins and an alteration in lipid membrane content. A combination of the altered cytoskeletal proteins, lipid membrane content, and osmotic swelling of the cell leads to bleb formation, release of these blebs, and resealing of the cell membrane (Fig. 12-1). Hepatocyte bleb formation, projection of these blebs through the fenestrations of endothelial cells, and release of these blebs during hypoxia are clearly shown in scanning electron micrographs (Lemasters et al., 1983). Bleb formation has been described with many conditions including ischemia, shock, viral infections, toxemia, and cholestasis. The magnitude of serum enzyme increase with reversible cell injury and bleb formation is not clearly understood, but it is likely that the magnitude of release and resultant increase activity in serum are considerably less than what might be observed with cell necrosis. Hence, it is reasonable to assume that the greater the magnitude of serum enzyme increase, the greater likelihood of some irreversible cell death, whereas mild serum enzyme activity increases may be associated with reversible cell injury. Although cytoplasmic enzymes may be released from cells into blood because of bleb formation, enzymes associated with the mitochondria are not released by this mechanism (Kamiike et al., 1989). Appreciable loss of membrane integrity and presumably cell death are necessary for release of mitochondrial aspartic aminotransferase (mAST) from hepatocytes. Ischemic liver does not lose mAST until almost all cytoplasmic aspartic aminotransferase (cAST) is lost. Regardless of the mechanism of release of the enzymes, there is evidence that the enzymes are released into the interstitial space where the greater portion is carried by lymphatics to the thoracic duct and emptied into the blood (Bolter and Critz, 1976; Lindena et al., 1986). Lymph-to-serum ratios of most enzymes are greater than 1:1, providing support for the delivery of enzymes to blood via the lymphatics. However, the direct delivery of the enzyme from the injured cell to blood cannot be discounted and is supported by the electron micrographs of cell blebs extending through the fenestrations of the endothelium as discussed earlier. This delivery of the enzyme from the injured cell to blood, whether directly or indirectly via lymphatics, likely affects the time of maximum serum increase of the enzyme after injury and duration of the presence of the increase of the enzyme in blood as suggested by the longer half-life of CK when injected intramuscularly as opposed to intravenous injection (Aktas et al., 1995). FIGURE 12-1 Membrane bleb formation in reversible injury, allowing the release of cytoplasmic enzymes either directly into blood or into the interstitial space where they can be carried by lymphatics to blood. Those enzymes attached to the external surface of cell membranes such as alkaline phosphatase (ALP), gamma-glutamyl transferase (GGT), and 5′nucleotidase (5′N) are released from cells to blood by distinctly different mechanisms than enzymes derived from the cytoplasm. Of historical interest, increases of serum ALP activity were perhaps first thought to result from a failure of excretion of bone ALP by the liver. This concept was put to rest many years ago and followed by the concept that cholephilic enzymes were shed from the bile canalicular surface of hepatocytes or biliary epithelial cells into bile and then regurgitated into blood through tight junctions. Support for this pathway was provided by evidence of disruptive changes to tight junctions in cholestasis and by experimental observations of infused horseradish peroxidase moving through tight junctions (Boyer, 1993; Lowe et al., 1988). However, increases of serum ALP and GGT activity have been shown to occur in the absence of increased biliary pressure and any evidence of alterations in tight junctions, and it is unlikely that this paracellular pathway is a significant contributor to the appearance of cholephilic enzymes in serum in most cases (Debroe et al., 1985; Putzki, 1989; Toyota et al., 1983). An alternative pathway of movement of cholephilic enzymes to blood has been suggested as a retrograde vesicular transport system following the observation that retrograde infusion of ferritin and polymeric and secretory forms of IgA undergo reversed transcytosis from the biliary or apical surface of the hepatocytes to the basolateral or sinusoidal surface during cholestasis (Carpino et al., 1981; Jones et al., 1984). However, neither of these pathways allows explanation of the appearance of ALP and GGT activity in blood in the absence of cholestasis. Studies using a choledochocaval shunt model show that within 12 h of shunting of bile or taurocholic acid into blood, there is a marked induction of ALP synthesis, appearance of ALP on the basolateral membranes, and a parallel increase in serum ALP activity (Ogawa et al., 1990). This occurs in the absence of increased biliary pressure and any evidence of alterations in tight junctions (Toyota et al., 1983). These observations along with others led to a third and more likely mechanism of the appearance of cholephilic enzymes, especially ALP, in blood. The basolateral appearance of enzymes typically considered to be on the apical membrane or bile canalicular surface is not unexpected as following synthesis all apical membrane proteins are believed to first be transported to the basolateral surfaces before vesicular transport to their final site on the bile canalicular membrane (Bartles et al., 1987; Maurice et al., 1994; Schell et al., 1992). Therefore, these so-called biliary enzymes or proteins have a brief period of residence on the sinusoidal surface of the hepatocytes with the enzyme on the external surface in the space of Disse so that they can potentially be released into blood if and when a suitable release mechanism exists. In addition, the quantity of enzyme available on the basolateral membrane and accessible for release into blood is increased at any time there is increased synthesis of the enzyme as described previously with the choledochocaval shunt model, as occurs with cholestasis and as may occur during hormonal or drug-driven induction of enzyme synthesis (Ogawa et al., 1990; Putzki et al., 1989; Solter and Hoffmann, 1999; Solter et al., 1997). Although positioned on the basolateral membrane facing the space of Disse for a brief period allows the possibility of release into blood, this does not occur without appropriate conditions to cleave the hydrophobic anchor. Alkaline phosphatase and 5′nucleotidase are anchored to the membrane via a hydrophobic phosphatidylinositol glycan anchor, whereas GGT is anchored via a transmembrane peptide therefore requiring different mechanisms of release. These release mechanisms will be discussed specifically in the sections dealing with each of these enzymes. The amount of enzyme activity in blood is very dependent on the rate of clearance of the enzyme from the blood following its release from cells. The half-lives of various enzymes range from minutes to hours to days, and the mechanisms or factors that determine the half-life of the various enzymes vary. The actual mechanisms of removal of enzymes from blood are not well established but likely are varied. Some small-molecular-weight enzymes such as amylase and lipase are, in part, filtered through the glomerulus. Enzymes that are glycoproteins are likely endocytosed by the galactose receptors on hepatocytes either directly via exposed galactose molecules or after loss of terminal sialic acid molecules resulting in exposed galactose residues, or are endocytosed by mannose receptors on Kupffer cells. Other enzymes may be degraded by proteases or are labile and activity is lost while the protein continues to circulate. The rate of clearance of enzymes from blood can be affected by disease and may complicate the correct interpretation of diagnostic test results. For example, pancreatic amylase activity, which is normally cleared by the kidneys, will increase in patients with renal failure because of the decreased glomerular filtration rate. A false-positive test result for pancreatitis could result. Changes to serum enzyme activity may in some cases reflect changes in enzyme production by the cells, rather than cell injury. Although there is certainly evidence of varying concentrations of cytoplasmic enzymes in cells, these generally do not result in dramatic changes in the serum activity of these enzymes. Marked increases in serum enzymes as a result of induction are most often associated with enzymes that are membrane bound where they can readily be released from the membrane into the lymphatics or blood or secreted by the cell. This induction can be as a result of hormonal changes, pathophysiological events such as cholestasis, or can be drug induced. Alanine aminotransferase (EC 2.6.1.2) (ALT), formerly known as glutamic pyruvate transaminase, catalyzes the reversible transamination of L-alanine and 2-oxoglutarate to pyruvate and L-glutamate. ALT, along with other transaminases, plays a role in amino acid catabolism and interorgan nitrogen transport. Pyridoxal 5′-phosphate (PP) is the cofactor of ALT, thus forming the active holoenzyme. PP is generally present in serum in sufficient quantities to provide near maximum activity of the ALT with only a reported 11% and 7% inactive apoenzyme in dog and cat serum, respectively (Stokol and Erb, 1998). There was no difference found between the percentage of inactive apoenzyme in the serum of normal animals and those with hepatic disease. However, two dogs were identified with 14,225% and 336% greater serum ALT activity when PP was added (Mesher et al., 1998). Approximately half the ALT in serum from a group of exercising Thoroughbred horses was in the inactive apoenzyme form (Rej et al., 1990). Hence, because there are cases in which PP seems to be limiting the measured ALT activity, some, but not all, commercial assays for ALT now contain added PP reagent. ALT activity is found in several body organs, but the magnitude of activity varies dramatically with species. In dogs, the ALT activity per gram of liver is at least four times greater than in other organs, although considerable activity is found in both heart and skeletal muscle (Clampitt and Hart, 1978; Keller, 1981; Zinkl et al., 1971). Similar findings are true for cats, but in horses, cattle, and swine, the ALT activity per gram of tissue differs little in liver when compared to muscle. Hence, based on tissue concentrations of ALT, increased serum ALT activity is somewhat specific for hepatic injury in dogs and cats but offers no specificity for detection of liver injury in horses and cattle. ALT, found in the cytoplasm of hepatocytes, is also found in mitochondria but generally at considerably lower concentrations, depending on species and tissue. Although it has been suggested that the mitochondrial enzyme may be released into blood more slowly following hepatocellular injury, this activity is still poorly understood and has not been utilized as a diagnostic tool. The half-life of ALT in blood is not clearly defined, although the circulation time is obviously adequately long to evaluate organ injury and release of ALT into blood for hours to days after the event. In dogs, reports have suggested half-lives of 3, 20, 45, and 60 h (Fleisher and Wakim, 1963; Reichard, 1959; Zinkl et al., 1971). Semilogarithmic plots of the decline in serum ALT activity following peak activity induced by acute CCl4 exposure suggest a half-life of between 45 and 60 h in dogs, although this may be a slight overestimation, as injured tissue is still likely present and contributing to the blood pool (unpublished data). The half-life of ALT from feline liver extracts, administered intravenously to cats, was estimated as 3 to 4 h (Nilkumuhaug and Thornton, 1979). This is consistent with the half-life of 6 h for ALP activity in the blood of cats (Hoffman et al., 1977). Serum ALT has been recognized as a marker of hepatocellular injury since the 1950s (Chimsky et al., 1956; Cornelius, 1958). The use of ALT as a diagnostic tool was expedited by the development in the mid-1950s of a simple coupled assay for ALT activity in serum that eliminated the problem of product inhibition (Reitman and Frankel, 1957). Numerous studies using carbon tetrachloride have clearly shown the value of serum ALT as an indicator of hepatocellular necrosis, especially in dogs and cats, but to a much lesser extent in horses, cattle, swine, sheep, and goats (Cornelius et al., 1958; Everett et al., 1977; Noonan, 1981; Noonan and Meyer, 1979; Spano et al., 1983; Turgut et al., 1997; Zinkl et al., 1971). The length of time that serum ALT activity is increased ranges from 9 to 23 days in dogs, which suggests prolonged injury to the liver but also supports the longer half-life suggested earlier (Guelfi et al., 1982; Noonan, 1981; Turgut et al., 1997). Relatively mild increases in serum ALT activity occur in dogs and cats with biliary obstructive diseases that cause serum ALP activity to increase markedly (Everett et al., 1977; Spano et al., 1983). Hence, the ratio of serum ALT-to-ALP activity is far greater in cases of hepatic necrosis than with cholestasis, suggesting that very general interpretive conclusions can be made by comparing the magnitude of increase of serum activity of these two enzymes. Increased serum ALT activity occurs with a wide range of other disorders including hypoxia secondary to anemia, metabolic diseases such as lipidosis, nutritional disorders such as copper toxicosis, inflammatory or infectious diseases, neoplastic diseases, and traumatic liver injury. Increased serum ALT activity has also been associated with numerous drugs; in many cases, these are likely idiosyncratic reactions causing hepatocellular toxicity. Exposure to carbon tetrachloride, mushroom alkaloids, or acetaminophen is clearly a hepatotoxic event. Mild to moderate increases in serum ALT activity are also observed in dogs and cats with endocrine diseases such as diabetes mellitus, hyperthyroidism, hyperadrenocorticism, and hypothyroidism. For example, 163 (78%) dogs with diabetes mellitus have increased serum ALT activity (Hess et al., 2000). Cats with diabetic ketoacidosis commonly have increased serum ALT activity (Bruskiewicz et al., 1997). Increased serum ALT activity is common in dogs with hyperadrenocorticism or dogs treated with glucocorticoids (DeNova and Prasse, 1983; Dillon et al., 1980; Solter et al., 1994). It has been shown in rats that ALT synthesis may be induced by glucocorticoids in order to increase function of the gluconeogenic pathways. However, experimental treatment of healthy dogs with glucocorticoids did not result in an increase in the concentration of hepatic tissue ALT activity, suggesting that increased hepatic mass plays a larger role than increased hepatocellular enzyme induction for an observed increased serum ALT activity (Solter et al., 1994). Although early studies of increased serum ALT activity following experimentally induced hepatocellular injury and the studies demonstrating much higher ALT activity in liver than other organs led to the early conclusion that increases of ALT activity in serum are specific for hepatocellular injury, there is clear evidence that serum ALT activity can also be increased as a result of injury to myocytes as well. Dogs in a colony with canine X-linked muscular dystrophy and ongoing muscle necrosis had increased serum CK, AST, and up to a 25-fold increase in ALT activity but a normal SDH activity, suggesting that myonecrosis contributed to the increased serum ALT activity (Valentine et al., 1988). This is consistent with the presence of some ALT activity in cardiac and skeletal muscle of dogs. In a case report of a cat with myokymia and neuromyotonia, the CK activity was 28,380, whereas the ALT activity was only 195 U/l; in a study of rhabdomyolysis in three dystrophin-deficient cats, the CK activity ranged up to 2040 times the upper limit of the reference range, whereas the ALT activity only increased to 19 times the upper limit of the reference range, suggesting only a minimal increase of serum ALT activity should be expected with muscle injury in this species (Galano et al., 2005; Gaschen et al., 1998). Although at least one early study in dogs showed a correlation between the magnitude of serum ALT activity and histological evidence of necrosis, other studies have reported little correlation (VanVleet and Albert, 1968). Similarly, bile duct ligation of dogs led to a 25-fold increase in serum ALT activity with minimal evidence of hepatocellular necrosis. As discussed in the introduction, the recognition of the formation of membrane blebs on hepatocytes and the rupture of these blebs during various conditions such as endotoxic shock, carbon tetrachloride-induced injury, cholestasis, and experimentally induced hypoxia have led to the understanding that there can be an increase of serum enzymes derived from the cytoplasm of the cell in cases of reversible cellular injury. In summary, the observation of increased serum ALT activity indicates hepatocellular (or myocyte) injury, but it does not necessarily imply irreversible injury and does not suggest a specific cause. Aspartate aminotransferase (AST: EC 2.6.1.1) (formerly glutamic oxaloacetic transaminase; GOT) catalyzes the transamination of L-aspartate and 2-oxoglutatarate to oxaloacetate and glutamate. As with ALT, pyridoxal-5′-phosphate (PP) is required as a cofactor. Although serum ALT was poorly saturated with PP in a study following exercise in horses, 94% of the AST was saturated and present as the holoenzyme (Rej et al., 1990). Providing PP in the assay reagent may be less of a concern when determining serum AST activity than when determining serum ALT activity. AST activity is relatively high and in similar amounts in liver and in skeletal and cardiac muscle, but it varies between species (Boyd, 1983; Keller, 1981). It is routinely used in equine and food animal medicine as a screening test for injury to both organs. Serum AST activity is readily available on the biochemical profile, has a longer blood half-life than sorbitol dehydrogenase and creatine kinase, and is stable for days in serum at room temperature, refrigerated, or frozen. AST is found in erythrocytes, and the addition of erythrocyte lysate to serum increases the apparent AST activity (unpublished data). AST is located in the cytosol but is in higher concentrations in mitochondria. There is only 48.1% amino acid sequence homology between cytosolic AST (cAST) and mitochondrial AST (mAST) from horse heart (Doonan et al., 1986). Likewise, the nucleotide sequences of cDNA of bovine mAST and cAST are also distinctly different (Aurila et al., 1993; Palmisano et al., 1995). Although there have been some efforts to show enhanced ability to identify organ-specific injury by assays for mAST and cAST, this has been shown to be of no diagnostic value (Jones and Blackmore, 1982). It may be theoretically possible to estimate the magnitude of reversible versus irreversible cell injury by determining mAST and cAST separately; however, this has not been studied empirically. Although the half-life of AST has been reported to be as long as 7 to 8 days in horses and as short as 163 min in dogs, neither of these seems reasonable based on data obtained following carbon tetrachloride toxicity (Fleisher and Wakim, 1963; Zinkl et al., 1971). Decreasing serum AST activity in horses recovering from CCl4-induced hepatotoxicity, as well as studies of equine myoglobinuria, suggests a half-life of 3 to 4 days (Bernard and Divers, 1989; Cardinet et al., 1967; Noonan, 1981). In cattle with mild CCl4-induced hepatotoxicity, serum AST activity during recovery suggests a half-life of approximately 1 day (Yonezawa et al., 2005). Serum AST has a longer half-life than creatine kinase, and therefore it would be expected to have increased diagnostic sensitivity during recovery from myocyte or hepatocyte injury. Increased serum AST activity is observed with both reversible and irreversible injury to hepatocytes and can be seen following hepatocellular injury and cholestasis, similar to serum ALT activity in dogs and cats. Likewise, serum AST is increased following myocyte injury. In either case, the definitive disease process cannot be identified, only that cellular injury in muscle or liver has occurred. Because serum AST activity cannot differentiate between hepatocellular or myocyte injury, further testing is often required using organ-specific enzymes such as sorbitol dehydrogenase or creatine kinase. Markedly increased serum AST and sorbitol dehydrogenase activity suggest acute or active hepatocellular injury, and markedly increased serum AST with modest to moderate sorbitol dehydrogenase activity suggests chronic hepatic injury or recovery from acute liver injury. Similar conclusions can be drawn using serum AST and creatine kinase activity. As with other cytosolic enzymes, serum AST activity cannot distinguish between reversible and irreversible cell injury as the cAST can be released by mechanisms involving nonlethal cell membrane blebbing. However, because a major portion of AST is of mitochondrial origin, the magnitude of increase during reversible cell injury is expected to be less than in irreversible injury, but this has yet to be clearly shown. The diagnostic sensitivity of serum AST activity in horses has been reported as 72% for hepatic necrosis and 100% for hepatic lipidosis (West, 1989). The specificity of serum AST activity was variable, and it decreased with primary gastrointestinal and orthopedic conditions secondary to affects on liver and skeletal muscle, respectively. In cattle, the sensitivity is reported to be 94% for hepatic lipidosis, 100% for leptospirosis, but only 53% for hepatic abcessation, and 46% for fascioliasis (West, 1991). Specificity was again variable depending on the primary condition. In summary, serum AST determinations are still part of many biochemical profiles because of their relatively high sensitivity for detection of hepatocyte injury and myocyte injury and stability in serum. However, serum AST activity clearly lacks specificity when compared to tissue-specific enzymes, such as sorbitol dehydrogenase and glutamate dehydrogenase for the detection of hepatocyte injury and creatine kinase for the detection of myocyte injury. Sorbitol dehydrogenase (SDH; EC 1.1.1.14), also known as iditol dehydrogenase, catalyzes the following reaction: The active sites of SDH contain Zn2+. Hence, when EDTA blood collection tubes are used, SDH activity is inhibited. Serum or heparinized plasma can be used for analysis. Sample stability has also been of concern for the use of SDH in diagnostic medicine with bovine serum SDH activity stable for at least 5 h at room temperature, 24 h refrigerated, and 72 h frozen, whereas in equine serum SDH remains stable for 5 h at room temperature, 5 h refrigerated, and 48 h frozen (Horney et al., 1993). In another study, bovine SDH activity was stable for 1 month at –20°C (West, 1991). SDH is not membrane bound and is located in the cytoplasm of cells. The highest concentration of SDH activity is in liver followed by kidney, but it is also found in most other tissues at much lower amounts (Boyd, 1983; Keller, 1981; Nilkumang and Thornton, 1979). SDH activity is considered liver specific in all species, and there have been no reports of nephrotoxicity causing increased serum SDH activity. The T1/2 of SDH in blood is likely relatively short in all species. Its reported T1/2, based on intravenous administration of cat or dog liver extracts, is 3 to 4 h for cats and 5 h for dogs (Nilkumhang and Thornton, 1979; Zinkl et al., 1971). The T1/2 of SDH in swine is reported as 1.6 h. In dogs and horses treated with CCl4, a rapid decrease in SDH activity following peak activity, supports a T1/2 of less than 12 h. The short circulatory half-life may be due in part to the labile nature of the enzyme, similar to that observed in serum samples in vitro. This short T1/2 limits to some extent the usefulness of the test, as it is easy to miss peak activity following a hepatic insult, and serum SDH activity may be within reference intervals in chronic hepatic disease. Because of its short half-life and the labile nature of SDH activity in serum, SDH activity is less favored for detection of hepatic disease in dogs than serum ALT activity. However, there are two occasions when SDH analysis may be useful in dogs. First, in dogs with traumatic muscle injury, where there is increased serum ALT and CK activity, a determination of SDH activity will quickly rule out whether there is concurrent hepatic injury. A second use of SDH activity determination in dogs might be in conjunction with ALT activity to determine if there is persistent hepatocellular injury. If the ALT activity is markedly increased and SDH activity is not, recovery is likely, but if both are markedly elevated, an ongoing insult to the liver is likely present. This sort of interpretation, however, is highly subjective and would require repeated monitoring to be of value. Serum SDH activity is of greater value than serum AST activity in large animals because of its increased specificity for hepatocellular injury. Marked increases of serum SDH activity occur within hours of experimentally induced hepatic necrosis in horses and cattle (Noonan, 1981). Serum SDH activity has been reported as a value for the detection of hepatic lipidosis, hepatic necrosis, leptospirosis, fascioliasis, and hepatic abscessation in cattle, and detection of hepatic necrosis, lipidosis, and cirrhosis in horses (Cebra et al., 1997; Lechtenberg and Nagaraja, 1991; West, 1989, 1991). Whereas the specificity of serum SDH activity in both cattle and horses with nonhepatic disease is 100%, the sensitivity for detecting hepatic lipidosis, hepatic abscessation, and leptospirosis in cattle was less than 50%; for detecting hepatic cirrhosis and lipidosis in horses it was less than 50%; and for detecting hepatic necrosis in horses it was 76% (West, 1989, 1991). In essentially all conditions evaluated in these two species, serum AST and glutamate dehydrogenase (GDH) activity were more sensitive than SDH activity. However, the specificity of serum AST and GDH was generally less than the specificity of SDH activity. The lower sensitivity may be in part due to the short half-life of the enzyme in circulation; especially in chronic low-grade conditions where large numbers of cells are not injured at any one time, the SDH activity may not exceed the reference range. Glutamate dehydrogenase (GDH) (EC 1.4.1.3) is a mitochondrial enzyme that catalyzes the removal of hydrogen from L-glutamate to form the corresponding ketimine acid that then undergoes spontaneous hydrolysis to 2-osoglutarate. The liver has by far the highest concentration of GDH activity (Boyd, 1983; Keller, 1981). Lesser amounts are found in the kidney and small intestine, where the GDH activity is located in the proximal and distal tubular epithelial cells and in the mucosal epithelial cells, respectively. The GDH activity of nonhepatic tissues is relatively small compared to that found in liver, where GDH is concentrated in the central areas of the lobule. In all species, increases in serum GDH activity are considered liver specific. As a result, there has been little or no interest in investigating isoenzymes of GDH in serum for diagnostic purposes. GDH is a zinc-containing enzyme whose activity can be inhibited by EDTA. Bovine serum GDH activity reported as stable for greater than 1 month at –20°C and was considered more stable than SDH (West, 1991). The in vivo half-life in six cows was reported as 14 h (Collis et al., 1979). This value is consistent with data from cattle recovering from hepatic injury and suggests that the half-life of serum GDH is greater than SDH but slightly less than the half-life of AST (Braun et al., 1995). The half-life of circulating GDH in dogs is reported as 8 h, based on intravenous injection of liver extract (Zinkl et al., 1971). Serum GDH activity is used most commonly in food animals and horses. Because of its location within mitochondria, GDH should be released only with irreversible cell injury. Following carbon tetrachloride–induced hepatic necrosis in calves and sheep, GDH activity increases but peaks approximately 1 day later than serum AST activity (Boyd, 1962). This may be due to the intramitochondrial location of GDH. Nevertheless, serum GDH activity was shown to significantly increase in acute, subacute, and chronic grass sickness in horses (Marrs et al., 2001). However, serum GDH activity was found to be highly variable in ponies exposed to pyrrolizidine alkaloids, suggesting that GDH activity may only be diagnostically useful in the acute stages of liver injury (Craig et al., 1991). This study found that serum GDH activity was increased with zone 1 hepatocyte necrosis, but it returned to normal reference intervals once all cells in this region were destroyed. These findings are consistent with the reported hepatic location of GDH in humans (Burtis and Ashwood, 1994). Increases in GDH activity of approximately 12-fold and AST activity two-fold were observed 24 h following halothane anesthesia in horses, which also may reflect the centrolobular location of GDH activity (Durongphongtom et al., 2006). As suggested earlier, the sensitivity of GDH activity varies depending on the nature of the disease. For example, in a study of calves with hepatic disease, GDH activity increased in only 60% of the animals (Pearson et al., 1995). Similarly, in cattle, the sensitivity of GDH activity for the detection of hepatic lipidosis, hepatic abscessation, leptospirosis, and fascioliasis was only 28%, 53%, 71%, and 72%, respectively (West, 1991). However, with all categories of hepatic disease described, the sensitivity of GDH activity was higher than SDH activity. The specificity for GDH was slightly less than that of SDH. In a similarly designed study in horses, the sensitivity of GDH activity for detection of hepatic necrosis, hepatic lipidosis, and hepatic cirrhosis was 78%, 86%, and 44%, respectively (West, 1989). The sensitivity was higher than that of SDH and comparable to that observed with serum AST activity. The specificity of GDH in this study was nearly 100%, which was comparable to the specificity of SDH and superior to that of AST activity. In a more recent study of the sensitivity of increased liver enzymes for diagnosis of hepatic disease, GDH showed a sensitivity of 63% (Durham et al., 2003). The determination of GDH activity is best done in conjunction with the determination of other hepatic enzymes and other indicators of hepatic injury or disease. Serum GDH determinations for diagnosis of hepatic disease in domestic animals have received less attention in the United States than in some other countries. However, the increased stability of the enzyme, longer half-life, and apparent greater sensitivity discussed earlier suggest it may be a more useful test for horses and food-producing animals than the determination of SDH activity. Gamma glutamyltransferase (GGT) (EC 2.3.2.2) functions in the gamma glutamyl cycle where it catalyzes the transfer of gamma glutamyl groups from gamma glutamyl peptides such as the tripeptide glutathione to other peptides, amino acids, and water. In conjunction with a peptidase, GGT plays a major role in regulation of intracellular glutathione by hydrolysis of the tripeptide glutathione outside the cell into its three components, which can readily be taken up by the cells and be available for glutathione synthesis as needed within the cell. GGT also functions in the GSH transferase/GGT pathway that cleaves gamma glutamyl moieties from GSH conjugates, which aids in the detoxification of xenobiotics and carcinogens by rendering them more water soluble and readily excreted (Lieberman et al., 1995). This pathway also plays a role in metabolism of mediators such as leuko-trienes, hepoxillins, and prostaglandins. The tissue distribution of GGT has been studied in numerous domestic species with the highest concentration found in kidney, pancreas, intestines, and the mammary glands of dogs, cattle, goats, and sheep but at much lower concentration in mammary gland of horses. Less GGT activity is found in liver, spleen, intestine, lung, and seminal vesicles. The GGT activity per gram of liver tissue is consistently lower than in kidney but varies between species, with the highest liver GGT activity in cattle, horses, sheep, and goats. Serum GGT reference values are consequently higher in those species than in dogs and cats (Braun et al., 1983, 1987; Milne and Doxey, 1985; Rico et al., 1977a, 1977b; Shull and Hornbuckle, 1979). Efforts have been made to determine the presence of isoenzymes of GGT in laboratory animals and humans, but it is likely that only one form of GGT exists. Variations in sialic acid content have been reported and likely explain the ability to separate different fractions of GGT by various means, such as isoelectric focusing (Mortensen and Huseby, 1997). Only one band of GGT activity was found with cellulose acetate electrophoresis of serum from dogs (Milne and Doxey, 1985). Removal of GGT activity from blood likely involves endocytosis by the asialoglycoprotein or galactose receptor, as purified human liver GGT that has been fractionated by ion exchange chromatography and infused into rats has shown that the slowest rate of clearance is associated with the most sialated forms (Morensen and Huseby, 1997). Clearance can be blocked with asialofetuin, also suggesting that clearance of GGT is via the asialoglycoprotein or galactose receptor on hepatocytes. GGT is apparently eliminated from blood without prior desialylation via exposed galactose units binding with low affinity to the receptor. The rate of removal of each molecule may be related to the number of available galactose molecules not blocked by sialic acid. The half-life of GGT activity in blood is not known, as there have been no definitive studies done in domestic animals to our knowledge. However, in dogs, serum GGT and ALP activity increase and decrease in parallel during cholestasis, suggesting that in dogs the half-life of GGT may be similar to the approximate 3-day half-life of liver ALP. A half-life of 3 days is also suggested for GGT activity in horses (Barton and Morris, 1998). In any event, the half-life of liver GGT activity is of sufficient length that increases are maintained throughout the disease process and diagnostic sensitivity is not lost because of a rapid return of the GGT activity to reference ranges. Conditions such as hepatic necrosis and reversible hepatocellular injury induced by CCl4, dibromobenzene, chloroform toxicity, and trauma result in minimal or no changes in serum GGT activity in dogs (Barakat and Ford, 1988; Guelfi et al., 1982; Noonan and Meyer, 1979). However, some increase in serum GGT activity is observed with chloroform toxicity in horses, although no increase is observed in calves and sheep (Barakat and Ford, 1988). Following administration of CCl4 to ponies, a four-fold increase in serum GGT activity was observed, which persisted up to 10 days with no increase in serum ALP activity (Hoffmann et al., 1987). The minimal increase in GGT activity in serum following hepatocellular injury is likely due to the fact that GGT in liver is primarily associated with biliary epithelial cells and the absence of significant increase of bile acids to release the hydrophobic transmembrane attachment to the cell membrane. Increases in serum GGT are most often observed with cholestasis and conditions resulting in biliary hyperplasia in all species, as observed in experimentally induced cholestasis (DeNova and Prasse, 1983; Hoffmann et al., 1987; Shull and Hornbuckle, 1979; Spano et al., 1983). Serum GGT activity is an especially useful clinical indicator of cholestasis in horses and cattle because of relatively high liver GGT activity compared to dogs and cats. Serum GGT activity in horses and cattle has relatively higher sensitivity for the identification of cholestatic disorders than serum ALP activity. Only a two-fold increase of serum ALP activity was observed with cholestasis, whereas serum GGT activity rose nine-fold in horses (Hoffmann et al., 1987). Although the magnitude of increase is greatest in cholestasis, serum GGT activity can be used in large animals as a screening test for generalized hepatic disease as well. In a retrospective study of 50 cases of hepatic disease in horses, of the serum chemistry parameters evaluated only serum GGT activity increased in all cases (McGorum et al., 1999). Similarly, serum GGT activity showed 75% sensitivity and 90% specificity for detecting subclinical liver disease in horses exposed to pyrrolizidine alkaloids, whereas serum ALP activity showed only 58% sensitivity (Curran et al., 1996). Serum GGT activity was shown to be the most sensitive serum enzyme for detection of hepatic injury secondary to proximal enteritis in horses (Davis et al., 2003). Increases in serum GGT activity in numerous cases of plant-related hepatotoxicity in both cattle and horses have also been reported (Craig et al., 1991; Curran et al., 1996; Mendel et al., 1988). Serum GGT activity in cats and dogs is often interpreted in conjunction with serum ALP activity. The suggested advantage of serum GGT activity determination over serum ALP activity in dogs is increased specificity, as GGT activity is derived solely from liver whereas serum ALP activity is derived from bone and liver as well as the canine corticosteroid induced isoenzyme of ALP. A study of 270 dogs with suspected hepatic disease had the results of hepatic biopsies compared to serum hepatic enzyme results (Center et al., 1992). Hepatic disease was confirmed by histology in 207 of the 270 cases. The sensitivity of serum ALP and GGT activity to detect histologically confirmed hepatic disease was 85% and 46%, respectively. However, the specificity of serum ALP was only 51%, whereas the specificity of serum GGT was 87%. In a study of 69 cats with suspected hepatic disease, of which 54 had histological evidence of hepatic disease, the overall sensitivity of serum ALP and GGT activity was 48% and 83%, respectively (Center et al., 1986). Serum GGT activity was more sensitive than serum ALP activity for detection of extrahepatic cholestasis, cholangiohepatitis, and cirrhosis. In contrast, the percentage increase of serum ALP activity was greater than serum GGT activity in 11 of 15 cats with hepatic lipidosis. This is likely because hepatic lipidosis is a form of intracellular cholestasis, ALP is primarily associated with hepatocytes, and GGT is primarily associated with the biliary epithelial cells. Several laboratory animal models have been used to study the mechanism of increase of serum GGT activity. These include bile duct ligation, treatment of the animals with alpha naphthyl isothiocyanate (ANIT) to cause necrosis of biliary epithelial cells, and a choledochocaval shunt (CCS) model that shunts bile from the common bile duct directly into the anterior vena cava (Bulle et al., 1990; Hardison et al., 1983; Kryszewski et al., 1973; Leonard et al., 1984; Putzki et al., 1989). In the bile duct ligation model, there is an initial increase of serum GGT activity and a decrease in liver tissue GGT activity. The rise in serum GGT activity is associated with a parallel increase in serum bile acids, and it is almost certain that the bile acids alone or in conjunction with a hydrolytic enzyme facilitate solubilization or release of GGT from the membrane. With persistence of cholestasis, there is a proliferation of biliary epithelial cells and an increase in bile duct volume in the liver, which is paralleled by an increase in GGT activity in the liver and a second phase of increase in serum GGT activity. In the ANIT model, chronic treatment with ANIT results in repeated necrosis of the biliary epithelial cells and bile duct proliferation. As the bile duct mass increases, there is a persistent increase in serum GGT activity. These two models both support the concept that there is an initial release of GGT activity from injury to biliary epithelial cells and retention of bile, the magnitude of which is determined in part by whether the species has low or high tissue GGT activity. Persistently increased serum GGT activity may indicate biliary hyperplasia that provides an increased source of GGT for release. Although not confirmed experimentally, clinical observations suggest that domestic animals with markedly increased serum GGT activity often have biliary hyperplasia. The CCS model in rats is unique in that it provides persistently increased liver and blood bile acids and increased bile flow, but without increased biliary pressure (Hardison et al., 1983). In this model, within 24 h, serum GGT activity nearly equals that seen with experimental bile duct ligation, suggesting that bile acids or other bile constituents mediate the release of GGT into serum. Moreover, increased biliary pressure and regurgitation of GGT through tight junctions are not necessary for the observation of increased serum GGT activity (Putzki et al., 1989). The actual pathway traveled by GGT from the biliary epithelial cells to blood is unclear. It is also unclear if the amount of GGT on the sinusoidal surfaces of hepatocytes is adequate to account for the magnitude of increase of serum GGT activity observed in any of the three models described previously. A mechanism of release from hepatocytes analogous to that described for ALP, but with a different hydrolytic enzyme, might be considered. Increased serum GGT activity in calves led to the recognition that species that produce large amounts of GGT activity in mammary glands may excrete or release GGT into colostrum. Colostral GGT is taken up by passive transfer in the newborn and serves as an easy, inexpensive, and automated test for successful passive transfer (Braun et al., 1982; Perino et al., 1993; Wilson et al., 1999; Zanker et al., 2001). The difference between presuckling and postsuckling serum GGT activity can be more than a 100 fold in calves (Braun et al., 1982). This increase correlates well enough with increased serum immunoglobulin to allow serum GGT activity to substitute as a test of adequate immunoglobulin transfer (Perino et al., 1993). However, GGT activity decreases steadily for the first 18 to 20 days, so the ability of serum GGT activity to accurately conclude failure of passive transfer is reduced after approximately 8 days (Wilson et al., 1999). In a review of failure of passive transfer in calves, the authors concluded that the loss of correlation between serum GGT activity and immunoglobulin concentrations after the first few days of suckling negates the value of the test, and its use should be discouraged in cattle (Weaver et al., 2000). Serum GGT activity also indicates passive transfer of immunoglobulins in goats, but in foals there is no difference in pre- and postsuckling serum GGT activity (Braun et al., 1984; Patterson and Brown, 1986). In canine pups postsuckling serum GGT activity can reach up to 100 times the upper interval because of high colostral GGT activity (Center et al., 1991). Although renal tissue has the highest concentration of GGT activity per gram of tissue in all species studied, there is no evidence supporting the presence of renal tubular GGT in blood. This is likely the result of the location of GGT on the luminal surface of the tubular epithelial cells and possibly rapid clearance from blood by the galactose receptor on hepatocytes. However, the location of GGT on the tubular epithelial cells means that the enzyme is readily shed into urine and can indicate renal tubular cell injury. Because of the variability of urine volume, urine GGT activity must be normalized to urine creatinine concentration using a GGT activity:creatinine ratio. Numerous reports show that the urine GGT activity:creatinine ratio in dogs is a sensitive and early indicator of chemical-induced nephrotoxicity with several compounds including maleic acid, aminoglycosides, and cyclosporine (Clemo, 1998; Graur et al., 1995; Nahas et al., 1997; Rivers et al., 1996). Furthermore, both 24 h excretion of GGT and the GGT activity:creatinine ratio of single urine samples have proven useful in detecting tubular injury in dogs (Gosset et al., 1987; Rivers et al., 1996). Although there is no apparent circadian variation (Uechi et al., 1994), it has been suggested that the within-day variation of the GGT activity:creatinine ratio limits its usefulness (Gosset et al., 1987). As in dogs, the urine GGT activity:creatinine ratio in horses and cattle has proven of value in detecting nephrotoxicity (Meyer et al., 2005; Rossier et al., 1995; Ulutas and Sahal, 2005). The magnitude in all species is greatest in the acute phase of injury after which the GGT activity in urine drops rapidly during the chronic stage. These studies support the use of urine GGT activity as a screening test of potentially nephrotoxic drug exposure. Evaluation of more than one enzyme and at multiple time points is required to properly evaluate for nephrotoxicity. It should also be noted that the urinary GGT activity:creatinine ratio is extremely sensitive and increases can be observed with no clinical signs of nephrotoxicity or azotemia (van der Harst et al
Animals
I. INTRODUCTION
II. HISTORY OF CLINICAL ENZYMOLOGY
III. FACTORS AFFECTING SERUM ENZYME ACTIVITY
A. Organ Mass and Enzyme Tissue
Concentration
B. Cell Location
C. Mechanisms of Release of Cytoplasmic
Enzymes or Other Protein Biomarkers from
Cells to Blood
D. Mechanisms of Release of Membrane-
Bound Enzymes
E. Blood Clearance Rates of Enzymes
F. Enzyme Induction
IV. SPECIFIC ENZYMES
A. Alanine Aminotransferase
B. Aspartate Aminotransferase
C. Sorbitol Dehydrogenase
D. Glutamate Dehydrogenase
E. Gamma Glutamyltransferase
Stay updated, free articles. Join our Telegram channel
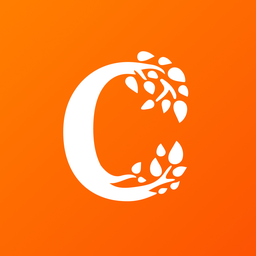
Full access? Get Clinical Tree
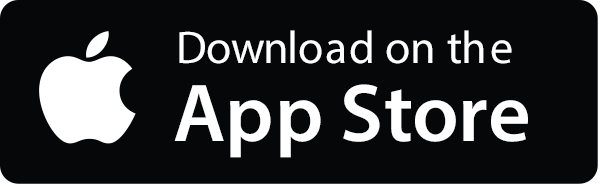
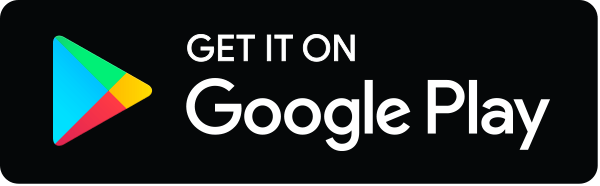