Chapter 10 Hemostasis A. Role of Vascular Endothelium C. Coagulation Proteins, Complexes, and Thrombin Activation III. LABORATORY ASSESSMENT OF HEMOSTASIS A. Quality Control and Reagent Variation B. Routine Testing of Hemostasis V. BEYOND HEMOSTASIS: INTERACTIONS WITH INFLAMMATION The maintenance of blood fluidity occurs through the process of finely balancing hemorrhage and clotting. Hemostasis, which is the process of arresting the escape of blood from the vascular system, is integral to survival in animals, is regulated by a series of orchestrated events, and is dependent on the vessels through which blood flows, as well as numerous proteins (coagulation factors, inhibitors, and fibrinolytic proteins) and cells (platelets, endothelial cells, and monocytes predominantly). Other systems in the body are also closely related to blood clotting and influenced by the activation of hemostasis such as the innate immune system (complement and phagocytes) and the inflammatory response (the kinin system and the cells important for hemostasis). The stepwise process that takes place to minimize blood loss and repair the injury includes (1) initial vasospasm, (2) platelet activation and plug formation, (3) assembly and activation of the coagulation cascade factors, (4) fibrin clot formation at the site of injury, and (5) dissolution of the clot and vascular repair. Each of these processes is described in more detail in the discussion that follows. Numerous reviews are available that describe the hemostatic process in detail (Crawley et al., 2007; Hoffman and Monroe, 2007; Hopper and Bateman, 2005; Sere and Hackeng, 2003). The goals of this chapter are to review the comparative biochemistry of hemostasis and to describe how the dramatic variations in blood clotting in different species impact laboratory testing, response to therapeutic agents, downstream effects on other body systems, and the array of diseases that can occur. Endothelial cells that line the lumen of blood vessels are the principal components of the vessel wall that contribute to maintenance of the fluid state of blood during health and the formation of clots during vascular compromise. Besides serving as a direct barrier between blood and tissue, these cells are actively involved in the regulation of hemostasis, inflammation, host defense, and numerous metabolic reactions. The phenotype of endothelial cells varies throughout the body, so the importance of these functions in different parts of the vascular system also varies. The endothelium exhibits plasticity with respect to hemostasis in that it is the balance of pro- and antithrombotic mediators that determine whether normal blood flow, hemorrhage, or clot formation occurs (Table 10-1). Unregulated or widespread activation of endothelial cells can have drastic consequences, as observed with disseminated intravascular coagulation (DIC). TABLE 10-1 Antithrombotic and Prothrombotic Properties of Endothelium During homeostasis, endothelial cells promote an anticoagulant and anti-inflammatory state. This is facilitated by several factors including the production of prostacyclin (PGI2), adenosine, and nitric oxide, which act together to inhibit the association of platelets with the endothelium and with other platelets (Becker et al., 2000). The expression of thrombomodulin on the lumenal surface binds any thrombin that may be formed and activates protein C to down-regulate the effects of any factor V (FV) and factor VIII (FVIII) that may be activated (see Section II.C.5.c). Tissue factor pathway inhibitor (TFPI) is synthesized by endothelial cells and is important for preventing co-localization of tissue factor with activated FVII (FVIIa), which would stimulate downstream production of thrombin and subsequently fibrin (see Section II.C.5.a). Tissue plasminogen activator (tPA) from endothelial cells activates plasmin, which results in lysis of any fibrin that is formed (see Section II.D.3). Proteoglycans such as heparin, heparan sulfate, and dermatan sulfate inhibit clotting factors and platelet aggregation. Finally, there is a relative lack of expression of adhesion molecules (e.g., P selectin) that would facilitate tethering of platelets to the endothelial surface (Becker et al., 2000). The initial reaction to vessel injury is vasoconstriction. This is a transient effect that minimizes blood flow to the affected area and is mediated by an autonomic neurogenic reflex and vasoactive mediators including endothelin. Within minutes of vascular injury or activation, the anticoagulant effect can change to a procoagulant milieu with resultant clot formation. Several factors contribute to this alteration. The expression of TF on the endothelial cell luminal surface is usually limited, but if expression is enhanced, activation of the clotting mechanism can occur. At the same time, expression of thrombomodulin and heparan sulfate can be lost, removing an important inhibitor to fibrin clot formation. Release of von Willebrand factor (vWF) from endothelial Wiebel-Palade bodies facilitates the binding of platelets to subendothelial collagen. This contributes to platelet activation and release of granule contents, facilitating platelet aggregation and plug formation (see Section II.B.3.a). Plasminogen activator inhibitor-1 is released, negating the activation of plasmin and thereby minimizing fibrinolysis (see Section II.D.4.a). Thromboxane A2 and platelet activating factor (PAF) are released, which encourage further platelet aggregation and activation. Endothelial cells also contain FV, which, when available, greatly amplifies thrombin formation. Additionally, there is locally enhanced expression of adhesion molecules such as P-selectin, which promote tethering of platelets to the endothelial surface. If the endothelial cell becomes apoptotic, the exposure of phosphatidylserine (PS) on the outer cell membrane leaflet can support the direct formation of thrombin because it can act as the phospholipid source for the prothrombinase complex (Becker et al., 2000). Besides traumatic injury, endothelial dysfunction can occur in various disease states, which may have clinical consequences on a proper functioning hemostatic system. Examples include systemic inflammatory mediators such as tumor necrosis factor (TNF) and interleukin-1 (IL-1), various systemic viral infections, Gram-negative bacteria, rickettsial agents, and in people, cholesterol and oxidative lipoproteins as observed in atherogenesis (see Cullen et al., 2005 for a thorough review on atherosclerosis). The outcome of endothelial dysfunction observed in these disease states includes local or disseminated formation of thrombi, vascular permeability causing accumulation of extravascular fluid, and petechiae or hemorrhage. Platelet plug formation involves a complex, interrelated sequence of events that overcome local resistance to platelet activation long enough to permit the cessation of bleeding. It is not possible to describe the biochemical events involved in platelet function, activation, and aggregation in a strictly temporal sequence because of the numerous positive feedback reactions, multiple agonists, and complementary intracellular pathways that function in a cooperative, coordinated fashion (Fig. 10-1). For simplicity, the process that results from activation, and leads to platelet plug formation, can be divided into three major events. The initiating response is the tethering of platelets to ligands exposed in the perturbed extracellular matrix (ECM) and subsequent formation of a platelet monolayer around the site of damage. The platelet plug grows when additional activated platelets accumulate on top of this monolayer in a complex series of reactions involving “outside-in” and “inside out” signaling (see Section II.B.2). These events involve platelet shape change, the release of granule contents, and the formation of platelet-platelet aggregates that initiate contact-dependent signaling and the recruitment of additional intracellular signals. The various reactions are controlled by an integrated network of biochemical pathways (Levy-Toledano, 1999). Results of in vitro studies, involving the exposure of platelets from various mammalian species to a single agonist, indicate that there are species differences in the relative importance of various membrane receptors and signaling pathways (Gentry, 1992, 2000b). However, it is difficult to extrapolate the results of in vitro studies to the physiological setting. Under in vivo conditions, circulating platelets are exposed to multiple agonists and, once platelets are activated, the phenomenon of “crosstalk” between the various extracellular and intracellular signaling pathways occurs (Fig. 10-1). Although most of the current knowledge regarding platelet biochemistry has come from human and murine studies, it is likely that there are more similarities than differences in biochemical mechanisms among species. In addition to a full complement of biochemical pathways, an adequate number of circulating platelets with structural integrity are required for platelets to fulfill their central role in hemostasis. FIGURE 10-1 Simplified sequence of platelet activation and aggregation. Abbreviations: AA, arachidonic acid; ADP, adenosine diphosphate; PAF, platelet activating factor; TF, tissue factor; TXA2, thromboxane A2. Platelet formation is the culminating event of megakaryopoiesis. In bone marrow, long, cylindrical processes of megakaryocyte cytoplasm are pinched off, to form proplatelets, and released into the circulation (Nagata et al., 2003). These fusiform, or elongated, platelets undergo morphogenesis into mature platelets through additional fragmentation processes involving the fusion of cytoplasmic vesicles with the plasma membrane (Benke, 1993). Fusiform platelets constitute about 15% of the platelet population in the rat and guinea pig, and elongated platelets, up to 20μ long, can occur in horses (Tablin, 2000). The mature mammalian platelet is small, 5 to 7μ long and frequently less that 3μ wide (Tablin, 2000). Early clinical studies indicated that among the physiological roles of interleukins, including IL-1, IL-3, IL-6, and IL-11, was their ability to stimulate platelet production. More recent studies have confirmed that thrombopoietin (TPO) is the primary biochemical regulator of steady state megakaryopoiesis and platelet production (Kuter and Begley, 2002). TPO, also known as c-Mp1 ligand, is a lineage-specific cytokine that is constitutively synthesized in the liver. It is removed from the circulation by binding to the c-Mp1 receptor on platelets and bone marrow megakaryocytes (Kaushansky, 2002). The TPO molecule consists of 331 amino acids organized in two domains, a receptor-binding domain that shows some homology to erythropoietin and a highly glycosylated carboxy-terminal portion that stabilizes the TPO protein. Although TPO increases the size, ploidy, and number of megakaryocytes, and also stimulates the expression of platelet-specific markers, it does not appear to increase the rate of platelet shedding from late-stage megakaryocytes (Choi et al., 1996). Circulating TPO levels appear to be directly influenced by platelet mass because TPO levels rise and remain elevated during persistent thrombocytopenia. It is likely that TPO-independent mechanisms are also involved in platelet production because TPO–/– mice are healthy and show no signs of spontaneous hemorrhage, despite being thrombocytopenic (Alexander et al., 1996). The normal circulating life span of mammalian platelets is in the range of 3 to 10 days. The mechanisms involved in the clearance of senescent platelets from the circulation are not yet known, but evidence is emerging that platelet aging is associated with the universal cellular phenomenon of loss of membrane asymmetry. For example, the proportion of canine platelets with PS exposed on the plasma membrane increases with age and is accompanied by mitochondrial changes consistent with apoptosis (Pereira et al., 2002). Mammalian platelets circulate as discoid, anucleate sub-cellular particles that contain several types of organelles including α-granules, dense granules, mitochondria, and lysosomes (Triplett, 2000). The majority of mammalian platelets exhibit an extensive canalicular system that is continuous with the plasma membrane, also known as the open-canalicular system (OCS). The OCS not only plays an essential role in platelet adhesion and aggregation but it also facilitates the two-way exchange of biological compounds between the platelet interior and the surrounding plasma (Escolar and White, 1991). In platelets of domestic cattle, horses, and Asian elephants, there is no evidence for the presence of an OCS. Despite this major ultrastructural difference, the biochemical mechanisms involved in platelet aggregation in these species are generally similar to those of other mammalian species (Bondy and Gentry, 1988; Cheryk et al., 1997; Gentry et al., 1989; Segura et al., 2006). The biochemical mechanisms required for normal platelet function are tightly regulated. Multiple positive feedback reactions facilitate rapid adhesion and aggregation at sites of vascular injury, whereas negative feedback responses modulate the continuation of platelet activation and thus regulate the extent of thrombus formation once wound healing has been initiated. Several types of membrane proteins and phospholipids are essential for platelet adhesion and aggregation (Tables 10-2 and 10-3). Integrins are heterodimeric (αβ) type 1 transmembrane receptors in which each subunit typically contains a large extracellular domain and a short cytoplasmic tail composed of 20 to 60 amino acids. Integrins, like the membrane G-protein coupled receptors (GPCR), can be viewed as “two-faced” receptors. In the case of integrins, the face oriented to the platelet exterior interacts with ligands on the ECM, and the face internally oriented interacts with platelet cytoplasmic proteins. Ligand binding to either face can trigger information transfer across the plasma membrane to initiate biochemical responses at the other face. This type of cross-membrane signaling is referred to as “outside-in” and “inside-out” signaling depending on the direction of information transfer (Barkalow et al., 2003; Shattil and Newman, 2004). Current knowledge of integrin biochemistry has come primarily from the investigation of human and murine platelets and the impaired aggregation responses in “knockout” mice. The major integrins involved in platelet adhesion and the stabilization of the primary hemostatic plug are the glycoproteins GPIIb-IIIa (also termed αIIbβ3), α2β1, the glycoprotein complex GP1b-V-IX, and GPVI, which is a member of the Ig receptor superfamily (Boudreaux, 1996; Gibbins, 2004; Shattil and Newman, 2004). Other examples of integrins involved in cross-membrane signaling are the ephrins that activate specific Eph kinases to ephrins, enhancing platelet adhesion through phosphatidylinositol 3 (PI3) kinase and protein kinase C (PKC) mediated mechanisms (see Table 10-3) (Prevost et al., 2004). CD40, a phosphorylated transmembrane glycoprotein, a member of the TNF receptor superfamily, is constitutively expressed in platelets, but it is only functional following platelet activation by other agonists. It has been shown that CD40 participates in the release of P selectin (CD62P) and other α-granule constituents, following agonist stimulation of human platelets (Inwald et al., 2003). TABLE 10-3 Intracellular Activation Signaling Pathways Abbreviations: AA, arachidonic acid; DAG, diacyl glycerol; IP3, inositol 3-phosphate; MLCK, myosin light chain kinase; PAF, platelet activating factor; PI, phosphoinositol; PKC, protein kinase C; PLA2, phospholipase A2; PLC, phospholipase C. Several members of the GPCR superfamily have been identified as integral components of platelet membranes (Coughlin, 2005b). Platelet agonists bind to the surface-accessible N-terminal domain of these proteins causing information to be transmitted through a seven-transmembrane domain. This induces a conformational change in the intracellular C-terminal domain which, in turn, activates GPCR proteins associated with the intracellular surface of the receptor (Hamm, 2001). GPCR proteins are heterotrimeric, being composed of single α, β, and γ subunits, and are classified into families according to the structure of their α subunit. Members of four families have been identified in human platelets. Activation of Gs and Gi proteins induces an increase and decrease in intracellular cAMP levels, respectively, activation of Gq stimulates the β isoforms of phospholipase C (PLC, see Section II.B.3.c), and activation of the G12 contributes to the regulation of the platelet actin cytoskeleton through protein phosphorylation (Brass, 2003). Whether a similar array of G protein receptors is expressed on other types of mammalian platelets has yet to be determined. However, based on studies with murine platelets, it is evident that the abundance of G protein types is necessary to support the similarity of platelet responses to multiple dissimilar agonists (Yang et al., 2002). The most important group of platelet membrane lipids involved in hemostasis are the phospholipids (phosphoglycerides), which constitute 63% and 57% of the total lipid content of pig and human platelets, respectively (Gentry and Nyarko, 2000). The major phospholipids involved in platelet function are phosphatidylserine (PS), phosphatidyl ethanolamine (PE), phosphatidylcholine (PC), sphingosine (SP), and phosphatidyl inositol (PI). PI is the parent molecule for the downstream, or secondary messenger, signal transducers inositol triphosphate (IP3) and 1,2 diacylglycerol (DAG) (Table 10-3), whereas PC is hydrolyzed to arachidonic acid (AA) in activated platelets (Nozawa et al., 1991). In all species, AA serves as the precursor of thromboxane A2 (TXA2) and prostaglandins, such as prostacyclin (PGI2), and serves as an autocrine agonist in some species (Gentry and Nyarko, 2000). In unstimulated platelets the negatively charged PS and PE are found predominantly on the intracellular side of the plasma membrane, whereas the neutral phospholipids, PC and SP, are localized to the outer leaflet. In response to the elevated intracellular calcium levels that occur following platelet activation, PS and PE are translocated to the outer leaflet of the membrane. Here they congregate to form the lipid platform that is essential for the localized platelet membrane binding and proteolytic activity of two key enzyme complexes in thrombin formation, namely the factor VIIIa-IXa-Ca-PS (tenase) complex and the factor Va-IXa-Ca-PS (prothrombinase) complex (see Section II.C.3). Platelets contain three types of organelles, also known as granules, in which they transport various specific hemostatic and wound healing compounds around the body (Tablin, 2000). Dense granules contain small nonprotein molecules such as ADP, ATP, serotonin, Ca2+, and Mg2+. The release of ADP and serotonin, from the first layer of activated platelets that adhere to a site of vascular damage, is important in the recruitment of additional platelets and the development of the platelet plug (Fig. 10-1) α-granules, the largest granule population, contain a variety of proteins such as albumin, fibronectin, thrombospondin, and the hemostatic proteins, fibrinogen, and FV (Polasek, 2004). They also contain platelet-specific proteins, including β-thromboglobulin and platelet factor 4, platelet derived growth factor (PDGF), epidermal growth factor (EGF), transforming growth factor β (TGFβ), and endothelial cell growth factor (ECGF) (Rendu and Brohard-Bohn, 2001). The proteins stored in the α-granules are either synthesized by megakaryocytes or are endocytosed by platelets as they circulate around the body (Reed, 2004). Like other endocytotic cell types, platelets contain lysosomes that release hydrolases. It has been suggested that these hydrolases assist in the elimination of circulating platelet aggregates to prevent inappropriate thrombus formation (Rendu and Brohard-Bohn, 2001). Mitochondria and cytoplasmic glycogen stores provide the energy to support the granule exocytotic secretory process in activated platelets (Flaumenhaft, 2003). This exocytosis process involves contraction of the actin-myosin cytoskeleton and the apposition and fusion of each granule membrane with the platelet plasma membrane (Escolar and White, 1991; Reed, 2004; Rendu and Brohard-Bohn, 2001). In addition to the extrusion of granule contents, the alteration in platelet morphology results in the exposure of both GPIIb-IIIa integrin complexes and membrane markers, such as CD62 and CD63, on the surface of the activated platelets (Polasek, 2004). CD62, also referred to as P-selectin, has been used as a marker of activated canine platelets (Moritz et al., 2003a, 2003b). When the ECM is damaged, various macromolecules interact with platelets as they roll along the exposed subendothelial surface collagen. Of these, collagen-bound vWF is considered to be the most important for the initial tethering and adhesion of platelets to areas of vascular damage (Nieswandt and Watson, 2003). This occurs through the interaction of collagen-bound vWF with the GPIb-IX-V integrin complex. However, at the medium and high shear rates found in arteries and arterioles, this reaction is reversible and must be followed by the more stable binding of collagen to its specific platelet receptors α2β1 and GPVI (Table 10-2). The initial interaction between platelets and the ECM through vWF-GPIb-IX-V binding is important because it induces activation of intracellular tyrosine kinases, causing increased affinity of α2β1 for collagen binding as well as an increase in the affinity of GPIIb-IIIa for a variety of ligands including fibrinogen, vWF, fibronectin, vitronectin, and thrombospondin (Calvete, 2004). The two collagen receptors, α2β1 and GPVI, are thought to have complementary functions in platelet adhesion (Andrews and Berndt, 2004). In human platelets, α2β1 binds collagen in an Mg2+-dependent manner, tethering platelets to the ECM before platelet activation. At the same time, collagen-α2β1 binding facilitates the interaction between collagen and GPVI in a nontyrosine kinase-dependent reaction. GPVI is currently considered to be the major collagen receptor because it initiates shape change, activation, and secretion reactions following platelet adhesion (Nieswandt and Watson, 2003). In mouse platelets, it appears that GPVI provides the primary collagen signal that activates and recruits α2β1 integrins to the surface of the platelet so that collagen signaling is amplified (Chen and Kahn, 2003). One of the important intracellular pathways activated as a result of collagen binding to GPVI is the PLC pathway, specifically the activation of the PLCγ2 isomer (Savage et al., 2001). As platelets adhere to collagen and receptor activation occurs, they lose their resting discoid shape and extend pseudopods as they spread over the endothelial surface (Escolar and White, 1991; Gentry, 2000b). This increases the surface area of the platelets, which, coupled with the clustering of integrin molecules on platelet membranes and the increase in integrin affinity, results in the formation of irreversible platelet-endothelial binding via vWF and the GPIb-IX-V receptors and platelet-platelet interactions via vWF and fibrinogen binding to GPIIb-IIIa receptors (Boudreaux, 1996; Savage et al., 2001). The GPIb-IX-V receptor is a sialoglycoprotein-rich complex that contributes to the net negative charge on the platelet surface. GPIb consists of two disulfide-linked subunits, GPIbα and GPIbβ, that are associated with GPIX in a 1:1 complex (Lopez and Dong, 1997). In mouse platelets, the activation function of vWF binding to GPIb-IX-V is, like collagen binding to the GPVI receptor, dependent on tyrosine phosphorylation (Shattil and Newman, 2004). This phosphorylation activity is dependent on the FcR, a molecule that associates noncovalently with GPVI and GPIb-IX-V. FcRγ is a component of the multisubunit high-affinity receptor for immunoglobulin (IgE), and its tyrosine-phosphorylated active motif helps to recruit tyrosine kinases to the glycoprotein receptors. The binding site for vWF on the receptor complex is localized to a site on the GPIbβ chain, which can also serve as a thrombin receptor. The outside-in signaling events that ensue from vWF-GPIbβ binding include activation of protein kinase C (PKC), protein kinase G (PKG) and phosphoinositol 3 kinase (PI3K), elevation of intracellular Ca2+, and rearrangement of the cytoskeleton (Gibbins, 2004). These reactions complete the irreversible adhesion of the activated platelet monolayer to the damaged endothelium. As part of the defense mechanism against inappropriate platelet adhesion and aggregation, GPIb-IX-V receptors only bind vWF immobilized on collagen and not soluble vWF, except under conditions of high shear flow rates (Lopez and Dong, 1997). The continued growth of the platelet plug requires platelet-platelet adhesion that is accomplished through a process described as “contact-dependent” signaling and involves the expression and activation of GPIIb-IIIa receptors (Shattil and Newman, 2004). GPIIb-IIIa is the most abundant integrin on the platelet surface and is not only necessary for platelet-platelet aggregation but also for platelet secretion, the development of procoagulant activity, and clot retraction. This integrin is typical of type 1 membrane receptors in that it contains a relatively large globular external domain, formed by the association of the N-terminal ends of both the α and β subunits, a single pass transmembrane domain and short C-terminal cytoplasmic tails composed of 20 to 60 amino acids (Hynes, 2002). The ligand binding sites contained in the external domain are converted to a high-affinity state through several inside-out signaling mechanisms. Among these signals are the interaction of the α and β subunit cytoplasmic tails with cytoskeleton proteins, actin and talin, inducing the formation of larger actin-based signaling complexes that are essential for granule migration and the fusion of granule and plasma membranes that precede the secretion of granule contents (Williams et al., 1995). Fibrinogen is the primary hemostatic ligand for GPIIb-IIIa, and fibrinogen bridges between platelets are the backbone of stable platelet aggregates. Other plasma proteins, including vWF, fibronectin, vitronectin, and thrombospondin, that have an RGD sequence similar to that present in fibrinogen can also bind to the α subunit of the GPIIb-IIIa. Fibrinogen has two RGD sequences per monomer, but it can also bind to GPIIb-IIIa through a recognition site involving residues 400 to 411 on its γ chain (Calvete, 2004; Williams et al., 1995). Occupancy of GPIIb-IIIa binding sites with fibrinogen up-regulates this integrin by inducing microclustering, as well as initiating downstream signaling through activation of Src and Syk protein kinases (Tables 10-2 and 10-3) (Shattil and Newman, 2004). It is now recognized that GPIIb-IIIa plays a continuing role in platelet function after a thrombus has formed through its role in clot retraction (Osdoit and Rosa, 2001). It has been postulated that one of the reasons platelet-rich clots are more resistant to thrombolysis than platelet-poor clots is related to the lower affinity of tissue plasminogen activator (tPA) for the retracted fibrin fibers of a platelet-rich clot compared to the less retracted fibrin fibers of a platelet-poor clot (Collet et al., 2002) (see Section II.D.2.b). Although the biochemical mechanisms involved in clot retraction are poorly understood, studies with mouse platelets have shown that after the initial wave of tyrosine phosphorylation initiated by activation of the β3 cytoplasmic tail of the GPIIb-IIIa receptor, a sustained GPIIb-IIIa-dependent tyrosine dephosphorylation of several polypeptides occurs (Osdoit and Rosa, 2001). This dephosphorylation response causes actin-dependent changes in the cytoskeleton that result in shrinkage in the size of the platelet-fibrin mass. In addition to immobilized collagen and vWF on the surface of damaged ECM, there are several soluble mediators that act as potent platelet agonists in the aggregation of mammalian platelets (Gentry, 2000b). The local accumulation of thrombin, generated from TF expression on the surface of damaged endothelial cells (see Section II.C.2), and ADP, released from α-granule stores in activated platelets, is essential for the growth of the primary hemostatic plug on top of the initial monolayer of collagen-bound platelets. Thrombin and ADP, and in some species TXA2, induce similar platelet-platelet aggregation formation through activation of the αIIbβ3 receptors (Brass, 2003). The major pathways of thrombin-induced activation result from hydrolysis of specific thrombin substrates, protease-activated receptors-1 and -4 (PAR-1, PAR-4), that are members of the G-protein coupled seven transmembrane domain receptor family (Major et al., 2003). To activate these receptors, thrombin cleaves the N-terminus, exposing a new N-terminus that serves as a “tethered-ligand” and binds to the extracellular-2 domain of the cleaved receptor (Dugina et al., 2002). A highly effective local concentration of this ligand is present, as it is not free to diffuse away from the platelet surface (Brass, 2003). Activation of PAR-1 and PAR-4 causes a downstream activation of Gq, G12, and Gi that, in turn, leads to the activation of the β isomer of PLC, PI3-kinase, and the inhibition of adenylyl cyclase, respectively (Table 10-2) (Grand et al., 1996). Studies on PAR receptors to date have focused on human and murine platelets, and results have revealed distinct differences in PAR receptor expression. In human platelets, activation of PAR-4 requires 10- to 100-fold higher concentrations of thrombin than PAR-1. Hence, PAR-1 is considered to be the more important thrombin receptor (Kahn et al., 1998). This difference in receptor sensitivity may be related to the hirudin-like sequences in PAR-1, but not PAR-4, that facilitate receptor cleavage by thrombin. In contrast, PAR-4 is the primary thrombin receptor in mouse platelets (Ishihara et al., 1997). In this species the cleavage of PAR-4 is facilitated by PAR-3 receptors. PAR-3 is the only member of the PAR family that does not possess an activating peptide, and, hence, it cannot directly activate platelets. PAR-2, the fourth member of the PAR family to be identified, is not activated by thrombin but by other serine proteases such as trypsin, TF, and FXa (Camerer et al., 2000). The PAR-2 receptor has not been found on platelets but is present in a number of other cell types, including endothelial cells. The potency of thrombin as an agonist may also be related to its ability to interact not only with PARs but also non-PARs, particularly the GPIbα component of the GPIb-IX-V complex (Soslau et al., 2001). It has been suggested that, in human platelets, binding of thrombin to GPIbα may facilitate PAR-1 cleavage in a manner analogous to the role of PAR-3 in mouse platelets (DeCandia et al., 2001). ADP was the first low-molecular-weight platelet-aggregating agent to be discovered and, like thrombin, is recognized as a universal agonist. It is stored in dense granules, in near molar amounts, and can be released not only from this source but also from damaged endothelial cells and red blood cells at sites of vascular damage (Gachet, 2001). ADP induces a broad range of biochemical changes in platelets. By itself, it is a relatively weak agonist, but it exhibits synergy with the stronger agonists, such as collagen and thrombin, which induce its secretion from dense granules (Mills, 1996). Further, low concentrations of ADP potentiate and amplify the effects of other weak agonists such as serotonin, epinephrine, and TXA2 (Dangelmaier et al., 2001). So far, three platelet ADP receptors have been identified: P2Y1, P2Y12, and P2X1 (Table 10-2) (Gachet, 2001). The central role of ADP in platelet function may be due, at least in part, to its ability to directly cause an elevation in intracellular calcium levels while simultaneously stimulating two biochemical pathways, one of which is mediated through Gq/PLC-mediated reactions (Table 10-3) and the other through Gi2-mediated inhibition of adenylyl cyclase. Co-activation of both Gq and Gi2 pathways, through P2Y1 and P2Y12, respectively, are essential for normal ADP-induced aggregation (Gachet, 2001). P2Y1 and P2Y12 are GPCRs that exhibit the usual seven hydrophobic domains and are sometimes referred to as “metabotropic.” In addition to activating the PLCβ isomer of PLC, the P2Y1 receptor induces phosphorylation of myosin light chain kinase (MLCK), which permits reversible incorporation of proteins, such as actin and talin, into the cytoskeleton to promote shape change (Savage et al., 2001). This cytoskeletal reorganization is accompanied by an increase in the levels of the regulatory subunit of P13 kinase analogous to that observed following activation of platelets by thrombin (Rittenhouse, 1996). When ADP interacts with the P2Y12 receptor it causes the heterodimeric Gi protein to disassociate into its αi2 and βγ subunits, which in turn causes the inhibition of adenylyl cyclase and a reduction in intracellular cAMP levels. These biochemical reactions slow the rate of calcium removal from the cytosol, which helps sustain the aggregation response. ADP also facilitates the development of stable platelet-platelet aggregates because activation of both the P2Y1 and P2Y12 receptors enhances the number and clustering of binding sites for fibrinogen on αIIbβ3 receptors (Gachet, 2001). The P2X1 receptor resembles a ligand-gated ion channel protein, exhibits two hydrophobic domains, and is referred to as “ionotropic” (Gachet, 2001; Mills, 1996). ADP interacts with its receptors as the anion ADP3–, indicating that positively charged groups on the receptors are involved in ligand binding. The major advances in knowledge of ADP receptors have come from studies with human and mouse platelets. It appears that at least the P2Y1 and P2Y12 receptors may be ubiquitous, although the relative number and sensitivity of receptors may differ (Coomber et al., 2006). For example, compared to human platelets, the P2Y12 receptor may have a more important role in equine platelets than the P2Y1 receptor (Mateos-Trigos et al., 2002). Platelet-activating factor (PAF) is also a potent soluble inducer of platelet aggregation but, unlike the universal agonists thrombin and ADP, not all mammalian platelets exhibit specific PAF receptors. Like human platelets, cow, sheep, horse, pig, dog, cat, elephant, guinea pig, and rabbit platelets respond to PAF at concentrations between 10–10 and 10–7 M (Kulikov and Muzya, 1998). Platelets from mice, rabbits, and hamsters are unresponsive to PAF. PAF is a proinflammatory lipid mediator with a unique 1-O-alkyl-glycerophospholipid backbone that exhibits multiple biological properties, including activation of platelets, neutrophils, monocytes, and macrophages (Chao and Olson, 1993; Prescott et al., 2000). In PAF activated endothelial cells and platelets, cytoplasmic PLA2 simultaneously releases AA and lyso-PAF that is converted to additional PAF molecules by acetyl CoA lyso-PAF transferase (Chao and Olson, 1993; Honda et al., 2002). In endothelial cells, PAF is translocated from its site of synthesis in the endoplasmic reticulum to the plasma membrane where the polar head of the molecule is inserted into the outer leaflet and serves as a juxtacrine ligand (Honda et al., 2002). In contrast, PAF formed in platelets, from lyso-PAF, is released into the circulation. PAF is degraded in the plasma by removal of an acetyl group at the sn-2 position, regenerating lyso-PAF and acetate. This reaction is catalyzed by a PAF acetylhydrolase that circulates in association with low-density and high-density lipoproteins (Prescott et al., 2000). The PAF receptor (PAFR) possesses the typical structure of the GPCRs. Therefore, PAF can induce intracellular protein phosphorylation in activated platelets indirectly through DAG stimulated protein kinase C activation, as well as through activation of tyrosine kinases (Table 10-2). It is well established that platelets from various species exhibit different sensitivities when exposed in vitro to a single dose of a weak agonist such as serotonin, epinephrine, or arachidonic acid (Dodds, 1978; Meyers and Wardrop, 1991, Pelagalli et al., 2002). Serotonin causes only a weak aggregation response in human, rabbit, cat, cow, sheep, and horse platelets, whereas canine platelets are unresponsive (Bailey et al., 2000; Mischke and Schulze, 2004; Ogawa et al., 1998). As in mammalian platelets, serotonin is present in thrombocytes of birds and reptiles (Maurer-Spurej, 2005). In these species serotonin acts as a vasoconstrictor and plays a role in endothermic body temperature regulation through its ability to regulate skin blood flow. It has been postulated that serotonin released from activated platelets may also have a role in mammalian thermoregulation (Maurer-Spurej, 2005). Canine and equine platelets are relatively unresponsive to epinephrine alone (Mischke and Shulze, 2004; Segura et al., 2005). The ability of epinephrine to act synergistically with collagen is used to advantage for the assessment of platelet function in whole blood with the PFA analyzer (see Section III.B.2.c). However, the response of both canine and equine platelets is lower when collagen-epinephrine is used as the agonist compared to collagen-ADP (Mischke and Shulze, 2004; Segura et al., 2005). The ligand binding of integrins and agonist binding to G-protein coupled receptors initiate intracellular signaling through multiple, interrelated pathways. The major signal transduction systems are summarized in Table 10-3. These biochemical pathways appear to be universal in platelets from all species, although they have been primarily investigated in human platelets. The limited information available for nonhuman platelets indicates that variations occur in the relative predominance of the individual enzymatic pathways (Gentry, 1992, 2000b). Protein phosphorylation by tyrosine and serine/threonine kinases is known to be critical for the modulation of the biological functions of platelets. Enzyme systems that have been identified include the nonreceptor tyrosine kinases, Src and Syk, that activate the cytoplasmic tail of the GPIIb-IIIa integrin β3 chain inducing an increase in the affinity of the αIIb chain for fibrinogen (Marshall et al., 2004). The fibrinogen binding reaction is also enhanced by protein kinase C (PKC), following its activation through a diacylglycerol (DAG)-dependent mechanism (Buensuceso et al., 2005). α2β1 activation of collagen-adherent platelets also stimulates phosphorylation of proteins such as Src, Syk, and the PLCγ2 isomer of PLC, all of which are components in the GPVI-FcRγ-chain cascade (Inoue et al., 2003). Activation of platelets by collagen-GPVI interaction induces PKC-Ca2+-mediated activation of the MLCK that, in turn, induces the cytoskeletal changes necessary for granule secretion. The phosphorylation of the myosin light chain can also occur through a Ca2+/calmodulin mechanism that is mediated by an extracellular signal regulated kinase (Erk2) (Toth-Zsamboki et al., 2003). The elevation of free cytosolic calcium, following receptor-mediated influx of extracellular calcium and secondary release of calcium from the dense tubular system, is one of the critical biochemical events in platelet activation in all species (Gentry, 2000b; Heemskerk and Sage, 1994). In addition to mediating the activation of PKC, calcium is directly involved in the regulation of phospholipid metabolism through activation of the calcium-dependent enzymes phospholipase C (PLC) and phospholipase A2 (PLA2). A further response to increased intracellular calcium is the lowering of intracellular cyclic adenosine monophosphate (cAMP) levels through activation of cAMP phosphodiesterase. The reduced cAMP levels exert a positive feedback response to further increase intracellular calcium levels, which, in turn, further enhance platelet reactivity and aggregation (Table 10-3). PLC is one of the secondary messenger systems that is universally activated by platelet agonists. It catalyses the cleavage of membrane bound phosphatidyl 4,5 bisphosphate (PIP2), one of the phosphorylated derivatives of PI, to lipophilic membrane bound DAG and hydrophilic IP3 that is released into the cytoplasm (Kroll and Schafer, 1989; Nozawa et al., 1991). DAG and IP3 act synergistically as signal transducers to promote all aspects of the basic platelet response, including shape change, increase in integrin and receptor affinity, and secretion of granule contents (Table 10-3). As noted previously, DAG-activated kinases, such as PKC, cause protein phosphorylation to mediate cellular changes such as increased affinity of GPIIb-IIIa integrins and a rise in intracellular calcium. IP3 is also instrumental in elevating calcium levels through the activation of a calcium transporting adenosine triphosphate (ATPase) system that mobilizes calcium from the dense tubular system. The continued functioning of the IP3-DAG system depends on the continued agonist-receptor-mediated activation of PLC, as both IP3 and DAG are rapidly converted to inositol and phosphatidic acid (PA), respectively. Both inositol and PA can be utilized for the resynthesis of PI and therefore are recycled within the platelet (Nozawa et al., 1991). In platelets from most species of domestic animals, collagen and thrombin are more potent activators of the phospholipase A2 (PLA2)-mediated secondary messenger system than are ADP or PAF (Gentry and Nyarko, 2000). PLA2 is an acyl hydrolase that cleaves the sn-2 acyl bond of the platelet membrane phospholipids, PC and PE, to release AA and lysophospholipids (Puri, 1998). AA is the major free fatty acid present in both platelet membranes and granules. Following its release into the cytoplasm, it is rapidly metabolized by the cyclooxygenase and lipoxygenase enzyme systems into labile products that function as both intracellular and extracellular messengers (Gentry and Nyarko, 2000). Unlike many nucleated cells, including endothelial cells that contain two forms of cyclooxygenase (COX), only the constitutively expressed form, COX-1, is found in platelets. In platelets, COX-1 first oxidizes and cyclizes AA to form prostaglandin PGG2, which it subsequently hydrolyzes to prostaglandin PGH2. These cyclic endoperoxides are rapidly metabolized to TXA2 by thromboxane synthetase; prostacyclin (PGI2) by PGI synthetase; or are converted to the eicosanoids, PGD2, PGE2, or PGF2 by isomerase enzymes that have yet to be fully characterized in platelets. In most mammalian platelets, TXA2 is the major metabolite, and in human platelets, it exerts a positive feedback effect on platelet aggregation through its receptor. Variable responses to TXA2 have been found in canine platelets, and bovine platelets do not respond to this agonist (Gentry, 1992, 2000b). It is possible that the variable response of mammalian platelets to the agonist effect of TXA2 is related to different receptor populations in different species. Because of the labile nature of TXA2, its stable metabolite, TXB2, is used to evaluate the extent of AA metabolism in activated platelets. Comparable to the difference in aggregation response to TXA2, the level of TXB2 released from thrombin-stimulated human, horse, and cat platelets is 10-fold greater than the amount released after similar activation of cow, pig, sheep, and mink platelets (Gentry and Nyarko, 2000). To balance the ability of platelets to be rapidly activated when needed, a number of regulatory mechanisms exist that prevent unwarranted platelet activation and limit the extent of aggregation. Most of the important physiological platelet inhibitors are endothelial-derived factors and their mediators (Table 10-4). The PGI2/cAMP and nitric oxide (NO)/cGMP inhibitory pathways have multiple synergistic interactions with respect to cyclic nucleotide generation/degeneration and protein phosphorylation in platelets (Schwarz et al., 2001). TABLE 10-4 Inhibitory Intracellular Signaling Mechanisms Abbreviations: DAG, diacyl glycerol; GKI, GMP-dependent protein kinase; IP3, inositol 3-phosphate; NO, nitric oxide; PECAM, platelet endothelial cell adhesion molecule; PGI 2, prostacyclin; PI, phosphoinositol. Eicosanoids, such as PGI2 and TXA2, are autocoids that only influence the activity of cells in the proximity of the producing cell. Both compounds are metabolites of AA, and whereas TXA2 is the major product in platelets, PGI2 is the major end product in endothelial cells after stimulation by agonists such as thrombin, ADP, and inflammatory mediators. PGI2 acts as a potent platelet inhibitor, whereas enhancement of aggregation occurs in response to TXA2 (deGroot and Sixma, 1996). To a great extent, platelet function is regulated by the intracellular concentration of cAMP. In platelets, as in other cells, cAMP formation is regulated bimodally by GPCRs that stimulate or inhibit adenylyl cyclase (Tables 10-2 and 10-3). PGI2 suppresses intracellular signaling in activated platelets by stimulating adenylyl cyclase formation via Gs-coupled IP receptors (Moncada and Vane, 1976). This results in the activation of a cAMP-dependent protein kinase (PKA) that stimulates the phosphorylation of a low-molecular-weight GTP-binding protein, rap 1B, which subsequently dissociates from the platelet membrane and inhibits PLC membrane binding. The net effect of this response is to suppress PI hydrolysis and reduce intracellular levels of IP3 and DAG (Table 10-4). In addition to these effects on phospholipid metabolism, elevated cAMP levels reduce platelet reactivity through a number of mechanisms. These include a reduction in GPIIb-IIIa expression and a reduction in the magnitude and duration of elevated cytosolic-free calcium after platelet activation (Gentry, 2000b). Human platelets generate cGMP through the soluble NO-activated guanylyl cyclase enzyme and degrade it through the phosphodiesterase (PDE 2 and 5) (Feil et al., 2003). The inhibitor effects of cGMP on platelet adhesion/activation are mediated through a cGMP-dependent protein kinase, cGKI (Table 10-4). The isomer present in platelets is cGKIβ, a homodimer consisting of two 75-kDa subunits. Each subunit is composed of an N-terminal domain that suppresses kinase activity in the absence of cGMP, a regulatory domain that contains two nonidentical cGMP-binding sites, and a kinase domain that catalyzes the transfer of the γ phosphate of ATP to the hyroxyl group of a serine/threonine side chain of the target protein (Feil et al., 2003). One of the ways in which cGKI contributes to a negative feedback response is through the inhibition of the Gq/Gi-coupled receptor responses, particularly in response to activation of the ADP-P2Y12 receptor (Atkas et al., 2002). Another negative feedback response is initiated by cGKI-mediated phosphorylation of platelet proteins, such as vasodilator-stimulated phosphoprotein (VASP), and is correlated with inhibition of the GPIIb-IIIa-fibrinogen receptor (Feil et al., 2003). Studies with cGKI-deficient mice have shown that platelet cGKI, but not endothelial or smooth muscle cGKI, plays an important role in the prevention of platelet adhesion and aggregation in the microcirculation after ischemia (Massberg et al., 1999). Platelet endothelial cell adhesion molecule (PECAM-1), also known as CD31, is a 130-kDa type 1 transmembrane glycoprotein whose function is regulated by phosphorylation of serine residues in the cytoplasmic domain (Newman and Newman, 2003). Based on cDNA sequencing, there appears to be considerable homology in PECAM-1 across species. Phosphorylated PECAM-1 serine residues have been detected in resting platelets, and their level of phosphorylation increases two- to three-fold in collagen and thrombin-activated platelets. PKC is thought to play a primary role in this phosphorylation process, although Src kinases may also be involved (Edmead et al., 1999). The presence of two distinct immunoreceptor tyrosine-based inhibitory motifs (ITIMs) in the cytoplasmic domain characterize PECAM-1 as belonging to the Ig-ITIM family of inhibitory receptors (Newman, 1999). ITIM-containing inhibitory receptors are thought to function primarily by counteracting signal transduction pathways initiated by receptors via their immunoreceptor tyrosine-based activation motifs, such as the FcRγ chain that is associated with the GPVI collagen receptor (Ravetch and Lanier, 2000). PECAM-1 is capable of inhibiting calcium mobilization, granule secretion, and aggregation following collagen activation of the GPVI/FcRγ chain complexes (Table 10-4) (Newman and Newman, 2003). It is important to note, however, that PECAM-1 inhibitory signaling can be overcome by strong stimulation of GPIV/FcRγ chain receptors, and that PECAM-1 does not inhibit GCPR signaling (Patil et al., 2001). Consequently, when endothelial damage is severe enough to expose high concentrations of collagen and to locally generate thrombin, PECAM-1 will not be an effective inhibitor of thrombus formation. FIGURE 10-2 Major components of pro- and anticoagulant systems. Black lines represent activation reactions, and red lines represent inhibitory reactions. Abbreviations: APC, activated protein C; AT, antithrombin; PC, protein C; PS, phosphatidylserine; TF, tissue factor; TFPI, tissue factor pathway inhibitor; TM, thrombomodulin; vWF, von Willebrand factor. Bleeding from a vessel may be transiently arrested by vasospasm and platelet plug formation, but the formation of a thrombus eventually occurs and provides a permanent clot while repair occurs. The soluble plasma coagulation proteins are required for formation of the thrombus. Most of these proteins are made in the liver, but other cells, including endothelial cells and platelets, are important sources of additional coagulation factors. Most coagulation proteins circulate in plasma as inactive zymogens, but when provided with the appropriate catalyst, they become activated in a cascade-like manner to ultimately produce thrombin and then fibrin (Fig. 10-2). Along with a phospholipid surface provided by activated platelets and calcium as a cofactor, this series of enzymatic reactions result in cross-linked fibrin deposition within the platelet plug. Most coagulation proteins have a Roman numeral designation, which is followed with an “a” when referring to the activated form (e.g., FVII is the inactive zymogen, whereas FVIIa is the activated protein). All coagulation proteins additionally may be referred to by a common or alternate name (Table 10-5). Specific details of the characteristics of individual factors can be found elsewhere (Dodds, 1997), but details on groups of proteins and how they contribute to blood clot formation are provided in Section II.C.2. TABLE 10-5 Coagulation Factors Currently, a single pathway, the tissue factor (TF) pathway, formerly known as the extrinsic pathway, is considered to be predominant in the initiation of thrombin formation (see Fig. 10-2). TF is a lipid-dependent transmembrane glycoprotein that is present in unactivated endothelial cells and monocytes, as well as free in plasma (Monroe and Key, 2007). TF is recognized to contribute to many biological processes in addition to coagulation, which include thrombus propagation, migration and proliferation of vascular smooth muscle cells, development of embryonic blood vessels, tumor neovascularization and metastasis, and induction of the proinflammatory response (Monroe and Key, 2007). Following cellular activation by vascular trauma or an inflammatory stimulus, TF becomes exposed on the plasma membrane where it interacts with circulating FVII, or its activated form, FVIIa, to form the enzymatically reactive TF-FVIIa complex (Gentry, 2004). About 99% of FVII circulates in the inactive zymogen form (Monroe and Key, 2007). Cell-associated TF binds to either FVII or FVIIa in order to either promote FVII activation or enhance catalysis, respectively. TF and FVII/FVIIa bind together over a large area at multiple sites in a Ca+-dependent manner to form the binary complex. The stabilization of the structure of FVIIa is crucial for its procoagulant activity. Unique from tenase and prothrombinase complex formation, TF-FVIIa complex formation is less dependent on a phospholipid surface for expression of its procoagulant activity (Monroe and Key, 2007). This complex functions primarily to convert FX to its activated form, FXa, but it also activates circulating FIX to FIXa. It is this FXa formation that results in initial production of thrombin (see Section II.C.3). Factor IX in plasma is proteolytically activated to the serine protease factor IXa by the TF-VIIa complex (see Section II.C.2.a) (see Fig. 10-2). FVIII normally circulates in a complex with von Willebrand factor (vWF), which effectively extends the plasma half-life of FVIII because it is protected from proteolytic degradation in the complex form (Gentry, 2004). Initial thrombin formation not only results in dissociation of FVIII from vWF, but it also converts it to a more potent cofactor, FVIIIa. Factor IXa and factor VIIIa then assemble on phosphatidyl-L-serine-containing phospholipid membranes in the presence of Ca2+ to form an enzymatic complex known as the tenase complex (Blostein et al., 2003). The tenase complex converts factor X to factor Xa, the enzyme that converts prothrombin to thrombin leading to the conversion of fibrinogen to fibrin and the formation of a fibrin clot. This tenase complex cleaves FX at the same reactive site as that cleaved by the TF-FVIIa complex and hence produces the same FXa product. Factor X activation by the tenase complex is the rate-limiting step for thrombin generation during tissue factor-dependent coagulation (Blostein et al., 2003). The generation of thrombin requires the formation of the prothrombinase complex, which consists of FXa, phospholipid, calcium, and a protein cofactor, FV (Gentry, 2004) (see Fig. 10-2). The complex catalyzes two cleavages in prothrombin, at Arg320 (to produce meizothrombin) and at Arg271, leading to the formation of thrombin (Autin et al., 2006). The first few molecules of thrombin generated by this prothrombinase complex initiate several positive-feedback reactions that sustain its own formation and facilitate the rapid growth of the blood clot or thrombus around the area of vascular damage. For example, thrombin can convert FXI to its proteolytically active form, FXIa, which, in turn, converts FIX to FIXa. The thrombin-induced conversion of FV to FVa, along with the increased availability of FXa, greatly enhances the rate and extent of thrombin formation by the prothrombinase complex. This is a crucial reaction for normal blood coagulation. FXa alone can slowly activate PT, but the rate of thrombin formation is enhanced several orders of magnitude by the presence of FVa in the PTase complex (Autin et al., 2006). Another positive feedback response is the increased availability of phospholipids on the surface on thrombin-activated platelets that accumulate at sites of vascular damage (Gentry, 2004). Minimal activation of coagulation proteins is needed to initiate further propagation and amplification of the cascade of reactions that ultimately leads to the formation of thrombin, which then converts fibrinogen to fibrin and forms the stable clot at a site of vascular injury (see Fig. 10-2). Each complex that participates in thrombin generation is simply composed of a serine protease interacting with a cofactor or receptor on an activated cell membrane surface (Mann, 2003). Coagulation is dependent on vitamin K because adequate concentration of this vitamin is required for activation of procoagulants, FVII, FIX, FX, prothrombin, and for anticoagulants, protein C, and protein S. The clinical consequences of antagonism or deficiency of vitamin K demonstrate how important it is to proper hemostasis (see Sections IV.B.5). The release of tissue thromboplastin or TF from damaged cells or its enhanced expression on cell membranes initiates in vivo coagulation. This occurs when TF binds with and activates FVII to FVIIa (see Section II.C.2.a). The TF-FVIIa complex then has the ability to activate subsequent zymogens FIX and FX in the presence of calcium ions and a negatively charged phospholipid surface provided by activated platelets (see Section II.B.2), resulting in the formation of their respective activated forms, FIXa and FXa. The latter is the more efficient substrate in the early phase of thrombin generation. Once some Xa has been formed, it actually facilitates further activation of FIX (Lawson and Mann, 1991). The TF-FVIIa activation process has historically been referred to as the extrinsic coagulation pathway and is currently often referred to as the tissue factor pathway. There appears to be a small proportion (1% to 2%) of constitutively activated FVII in plasma (Mann et al., 2003). However, it does not exhibit proteolytic activity unless bound to TF. Once the TF-FVIIa complex forms, the rate of FXa formation increased four-fold (Mann et al., 2003). During the initiation stage, any membrane-bound Xa produced is able to begin converting a small amount of prothrombin to thrombin. This small amount of thrombin is crucial for further propagation of the coagulation cascade, as it activates platelets and converts FV to FVa and FVIII to FVIIIa (Brummel et al., 2002). The ability of the TF-FVIIa complex to continue the generation of FIXa is compromised by the presence in the circulation of tissue factor pathway inhibitor (TFPI) (see Section II.C.5.a). Alternative activation pathways exist for the activation of FIX so that the tenase complex can be formed at a sufficient rate and adequate level to sustain thrombin formation until a clot has formed and wound healing is initiated. In a direct positive feedback reaction, thrombin can convert FIX to the same enzymatically active form of FIXa as produced by the TF-FVIIa complex (Minnema et al., 1999). In an indirect feedback pathway, thrombin can activate FXI to a proteolytically active enzyme (FXIa), which can, in turn, convert FIX to FIXa (Gailani, 2000; Gailani and Broze, 1991). FXI is one of the proteins involved in the coagulation pathway historically known as the intrinsic pathway that is now referred to as the contact activation system. The other components of this system are FXII, prekallikrein (PK), and high-molecular-weight kininogen (HK) (Table 10-5). This pathway is initiated when the zymogen FXII comes into contact with a negatively charged surface (e.g., damaged endothelium or an endotoxin contact) and undergoes a conformational change to become an active serine protease (FXIIa). FXIIa then propagates coagulation by activating the next protein in this system, FXI, in a reaction that is accelerated by the presence of PK and HK (Wachtfogel et al., 1993). In plasma, HK circulates as biomolecular complexes with FXI and PK, respectively. The initial molecules of FXIIa generated on damaged endothelium convert HK to HKa. HKa has a high surface binding affinity and thus brings large amounts of FXI and PK to the charged surface in close proximity to the already adherent FXIIa. The spatial configuration of these complexes permits FXIIa to rapidly convert FXI to FXIa and convert PK to kallikrein. It is now generally accepted that activation of the contact activation system is not the predominant in vivo mechanism of thrombin generation (Gentry, 2004; Rojkjaer and Schmaier, 1999). However, it may serve as a link between fibrin formation, fibrinolysis, and inflammation because kallikrein can function as a weak activator of the fibrinolytic system (see Section II.E) and as stimulator of both chemotaxis and degranulation in neutrophils attracted to a site of endothelial damage (Jiminez and Fernandez, 2000). After the coagulation cascade is initiated, further amplification ensures that sufficient thrombin is produced to cleave fibrinogen and form insoluble fibrin. Once FIXa is formed, it complexes with calcium ions (Ca) and platelet PSs and FVIIIa to form the FIXa-FVIIIa-Ca-PS (tenase or Xase) complex, a potent stimulator of further FX activation. Large amounts of FXa are created by the tenase complex, which is approximately 50-fold more efficient than the TF-FVIIa complex at performing the same process (Ahmed et al., 1992). Once formed, FXa converts prothrombin (factor II) to thrombin (FIIa). This reaction is additionally dependent on the formation of the prothrombinase complex, composed of FVa, FXa, platelet phospholipid surface, and calcium ions (FXa-FVa-Ca-PS) and is 300,000-fold more potent than Xa at catalyzing prothrombin conversion (Mann et al., 2003). Thrombin (FIIa) converts fibrinogen to fibrin and additionally activates factor XIII, which is important for cross-linking and stabilizing the fibrin clot (see Section II.C.4). Ultimately, repair of a vascular lesion requires development of a more permanent clot than a platelet plug can provide. A stable fibrin clot is the end point of the conversion of fibrinogen to fibrin and involves three phases: proteolysis, polymerization, and stabilization. Proteolysis occurs when thrombin cleaves fibrinogen to form fibrin monomers and the secondary by-product fragments fibrinopeptides A and B (FPA and FPB). These fibrinopeptide molecules are cleaved from the N-terminal regions of the Aα and Bβ chains (Sidelmann et al., 2000). It is proposed that removal of FPA permits end-to-end polymerization, whereas removal of FPB promotes side-to-side polymerization (Mosesson, 1992). The cleaved fibrinogen molecules can then form complexes with other fibrinogen and fibrin molecules to form what is referred to as soluble fibrin or fibrin monomers (Sidelmann et al., 2000). Fibrin monomers then polymerize at their ends via hydrogen bonding, and the polymers are then stabilized covalently via peptide bond formation under the influence of the transglutaminase FXIIIa (which is activated by thrombin) to form cross-linked fibrin strands. Thrombin cleaves a peptide from each of two alpha chains on FXIII, which results in the formation of an inactive intermediate. In the presence of Ca, this intermediate dissociates and produces the activated protein. FXIIIa then induces adjacent γ-chains to quickly cross-link to form γ-dimers, whereas α-chain cross-linking occurs more slowly. Even native fibrinogen can be cross-linked by FXIIIa, following the same pattern of initial γ-chain dimerization followed by Aα-chains to form high-molecular-weight polymers. The cross-linking that occurs between fibrin polymers makes the fibrin “gel” more resistant to lysis by fibrinolytic proteins such as plasmin. One way this occurs is by FXIIIa-mediated incorporation of plasmin inhibitor into the developing fibrin meshwork (see Section II.D.3). Plasmin inhibitor becomes bound to α-chains of both fibrinogen and fibrin and prevents the absorption of plasminogen to fibrin. FXIIIa can also cross-link other plasma proteins to fibrin strands (see Section II.C.4) (Sidelmann et al., 2000). Once the lesion has been repaired, which may also be partly mediated by FXIIIa (Barry and Mosher, 1988), a process called fibrinolysis dissolves the fibrin clot (see Section II.D). Several biochemical mechanisms exist that function to keep the formation of a blood clot localized to a site of vascular injury, to prevent the excessive growth of the clot, and to prevent thrombin generation and fibrin formation from becoming generalized to nondamaged areas of the vascular system. The target enzymes and cofactors for these and other circulating inhibitors are summarized in Table 10-6. TFPI is the principal endogenous inhibitor of the tissue factor pathway. TFPI is a Kunitz-type serine protease inhibitor that has a double inhibitory effect: it inhibits both FXa and the TF/FVIIa complex (Golino et al., 2002; Lindahl, 1997). Between 75% and 90% of the total body TFPI is located in endothelial cells; the remaining 10% to 25% circulates bound to plasma lipoproteins with only trace amounts being detectable free in plasma or in platelet cytosol. Because of its association with lipoproteins, TFPI was formerly known as lipoprotein-associated coagulation inhibitor (LACI). TFPI is a single-chain 32-kDa glycoprotein that has a highly negatively charged N-terminus connected to three tandem domains that show homology to Kunitz-type protease inhibitors and a highly positively charge C-terminal region (Broze, 1992). Multiple forms of TFPI have been identified in human plasma with 36- and 43-kDa forms predominating. This size variation appears to be due to the formation of disulfide bonds and glycosolation of the TFPI molecule (Broze, 1992; Lindahl, 1997). A 34-kDa form of TFPI circulates in association with plasma LDL, and a 40-kDa form associates with HDL. TFPI is not uniformly dispersed in the vasculature. It is constitutively expressed by endothelial cells of the microvasculature but not by endothelial cells of larger vessels (Doshi and Mamur, 2002). A localized increase of TFPI can occur at the site of a clot because thrombin is able to induce the release of TFPI from activated platelets (Novotny et al., 1988). TFPI is stored on the extracellular matrix of endothelial cells bound to GAGs such as heparan sulfate (Abildgaard, 1992). Consequently, infusion of heparin can displace this surface-bound TFPI to induce a two- to four-fold increase in circulating TFPI levels (Golino et al., 2002; Novotny et al., 1991). The liver effectively clears circulating TFPI by receptor-mediated endocytosis resulting in a half-life for TFPI of less than 70 min (Lindahl, 1997). The inhibitory activity of TFPI depends on the activation of the TF pathway and the formation of FXa. TFPI binds to FXa at, or near, its serine active site in a reversible calcium-independent reaction to form a 1:1 complex (Broze, 1992). This complex is formed most efficiently when FXa is incorporated into the prothrombinase complex (see Section II.C.3). The ability of TFPI to inhibit the activity of the TF/FVIIa complex depends on the TFPI/FXa complex forming an irreversible, calcium-dependent quaternary complex consisting of TFPI-FXa-TF-FVIIa (Lindahl, 1997). Hence, TFPI is not only able to inhibit FXa activity, but it is also able to suppress the generation of additional FXa by blocking the TF/FVIIa-catalyzed conversion of FX to FXa. TFPI is able to exert this bimodal inhibitory effect because the inhibition of FXa depends on the first Kunitz-type domain and an intact C-terminal region, whereas the second Kunitz-type domain of TFPI molecule is responsible for the binding of the TFPI/FXa complex to the TF/FVIIa complex (Lindahl, 1997). Because TFPI does not interfere with FXa activation by the tenase complex (FIXa-FVIIIa-Ca-PL, see Section II.C.3), it does not completely block thrombin formation. The importance of TFPI in regulating thrombin generation and fibrin formation has been demonstrated in animal models depleted of TFPI. These animals are sensitized to TF and readily develop DIC (see Section IV.B.4) in response to endotoxin, which causes the release of TF from endothelial cells (Huang et al., 1997; Sandset et al., 1991). Hence, one of the factors involved in DIC is an imbalance between the levels of TF and TFPI. Antithrombin (AT, also known as antithrombin III, ATIII) is the principal member of the superfamily of serine protease inhibitors, or serpins, that inhibit the action of several serine proteases of the hemostatic system (Pike et al., 2005). Serpins have a common mechanism of action that involves trapping and irreversibly inactivating the proteases through the formation of a covalent bond followed by the removal of the serpin-enzyme complex from the circulation by the action of receptors, which specifically recognize the inhibited conformation of the serpin (Gettins, 2002). One of the functions of serpins is to neutralize any of the procoagulant enzymes that escape from the vicinity of a blood clot and thus prevent inappropriate thrombus formation. AT is a 58-kDa single-chain glycoprotein synthesized in the liver and endothelial cells. Structural similarities exist in the AT molecule across many species (Frost et al., 2002; Johnstone, 2000). Although AT can inhibit several serine protease of the coagulation system including thrombin, FIXa, FXa, FXIa, and FXIIa, the principal targets are considered to be thrombin and FXa (Pike et al., 2005). The AT molecule has two functional sites: the reactive site for serpins and a binding a site for glycosaminoglycans (GAGs), such as heparin and heparan sulfate. GAGs serve as potent cofactors that enhance the rate of thrombin inhibition from one that is nonphysiological to a physiologically relevant level. In its unactivated, or native state, the AT molecule contains a reactive center loop that is partially inserted into its Aβ-sheet. When thrombin comes in contact with AT, it cleaves a specific bond in the reactive center loop causing it to become fully inserted into the Aβ-sheet and at the same time translocating thrombin from the top of the AT molecule to the bottom (Munoz and Linhardt, 2004). In this way, a 1:1 stoichiometric complex is formed through covalent bonding between the active site serine of thrombin and the reactive site arginine of AT. The interaction between GAGs, like heparin, and AT involves several steps. First, a low-affinity interaction occurs forming ion pairs between a cluster of positively charged amino acids, such as arginine and lysine, in the D-helix of the AT molecule and specific spatially defined negatively charged sulfo- and carboxyl groups in the heparin pentasaccharide sequence (Huntington et al., 1996). This binding induces a conformation change in AT that results in both stronger heparin binding and the expulsion of the reactive center loop of AT from the Aβ-sheet (Munoz and Linhardt, 2004). In effect, heparin facilitates the diffusion of thrombin and AT toward each other through the formation of a tertiary complex between heparin and AT. For example, in the presence of heparin the inhibition of thrombin by AT is accelerated 1000-fold from 4.4 min to 0.27sec and the rate of inhibition of FXa by AT is accelerated 10,000-fold (Olson and Shore, 1982; Pike et al., 2005). The formation of the FXa-AT-heparin complex requires the presence of calcium ions to overcome the negative effects of the Gla-domains in FXa (Rezaie, 1998). Because clot-bound thrombin and FXa are protected from inactivation by AT, the physiological role of AT is not to cause the cessation of clotting but rather to localize the clot and prevent it from spreading too far beyond the site of damage (Weitz et al., 1990). It also functions to prevent thrombin formation from occurring on undamaged endothelium. Heparan sulfate is an extracellular component of the extracellular matrix of all animal cells, whereas heparin is localized to the granules of mast cells and is only released locally in an allergic reaction. In the vasculature, only heparan sulfate in the outer monolayer of endothelial cells possesses the AT binding sites that can accelerate the inhibitory action of AT, whereas the heparan sulfate contained in the underlying layer of smooth muscle cells lacks AT binding sites (Munoz and Linhardt, 2004). Thus, when thrombin comes in contact with AT-heparan sulfate complex on the “healthy” intact vascular wall, the T-AT complex irreversibly inactivates it. However, when the vessel wall is damaged, thrombin is so inefficiently inhibited by AT, the conversion of fibrinogen to fibrin and hence clot formation can proceed (Pike et al., 2005). Heparin cofactor II (HCII) is a specific thrombin inhibitor that has a similar mechanism of action as AT, but it is dissimilar both antigenically and in its cofactor requirements (Johnstone, 2000). HCII appears to be synthesized exclusively by the liver as a 65.6-kDa glycoprotein with a unique amino-terminal extension that contains two tandem repeats rich in acidic amino acids with two sulfated tyrosines (Inhorn and Tollefsen, 1986). These repeats are homologous to the carboxyl-terminal sequence of hirudin, the specific thrombin inhibitor from the medicinal leech. Although heparin, heparan sulfate, and dermatan sulfate are all physiological activators of HCII, many different polyanions, including polyphosphates, polysulfates, and polycarboxylates, are also able to accelerate HCII inhibition of thrombin up to 1000-fold (Tollefsen, 2004). Although the physiological function of HCII has not been fully elucidated, it has been suggested that it may function as a thrombin-inhibitor reserve when AT levels become subnormal such as occurs in DIC (see Section IV.B.4; Tran and Duckert, 1984). Experimental animal studies and human clinical studies indicate that HCII may have a protective antithrombotic effect following arterial oxidative damage (Tollefsen, 2004). As summarized in Table 10-6, a number of other serpins participate in modulating hemostasis (Silverman et al., 2001). The broad spectrum protease inhibitor known both as α1-antitrypsin and α1-protease inhibitor (α1-PI) can inhibit thrombin, FXa and FXIa, APC, and plasmin, although its main physiological function is as a circulating antitryspin inhibitor. However, α1-PI appears to be a more effective inhibitor of FXIa than AT (Scott et al., 1982). It circulates as a 50-kDa glycoprotein and contains the characteristic reactive site loop of a serpin (Long et al., 1984). α2-Macroglobulin circulates as a large 650-kDa glycoprotein composed of four identical subunits. This protein can bind one or two molecules of a protease depending on its size. Small proteases such as trypsin maximally form 2:1 complexes with α2-M, whereas larger proteases such as plasmin form 1:1 complexes. It has been suggested that α2-M is one of the major physiological inhibitors of FXa in vivo (Fuchs and Pizzo, 1983). α2-M-protease complexes are rapidly removed from the circulation by the liver through interaction with the lipoprotein receptor-related protein that serves as a large multifunctional endocytosis receptor (Narita et al., 1998). This receptor protein is also responsible for the removal of other proteases such as tissue-type plasminogen activator and thrombin activatable fibrinolysis inhibitor (TAFI) (Orth et al., 1992; Warshawsky et al., 1994). C1 inhibitor is a 104-kDa single-chain glycoprotein that exhibits structural homology with other members of the serpin superfamily. It appears to be the main circulating inhibitor of FXIa, although it can also suppress the activity of FXIIa and kallikrein but not FXa or plasmin (Harpel et al., 1985; Pixley et al., 1985). As its name suggests, the main physiological role of C1 inhibitor is to modulate the complement system. Protein C inhibitor (PCI), also known as plasminogen activator inhibitor-3, inactivates the proteolytic activity of numerous serine proteases including thrombin, FXa, FXIa, APC, tissue-type plasminogen (tPA) activator, and urokinase-type plasminogen activator (uPA) (Meijers et al., 2002). The mature PCI protein is 57 kDa and is found in several body fluids as well as plasma (Pike et al., 2005). Like HCII, several GAGs and polyanions can enhance the activity of PCI. In the presence of heparin, a ternary complex is formed between PCI and the active protease through a GAG binding region localized on the H-helix region of PCI (Pratt and Church, 1992). However, even in the presence of heparin, the inhibitory effects of PCI for thrombin and FXa are still modest compared to those of AT and HCII (Pike et al., 2005). Although PCI can inhibit APC in the presence of heparin and calcium ions, the more physiologically relevant role of PCI in modulating the protein C anticoagulant system is the result of its ability to inhibit the activity of the thrombin-thrombomodulin (T-TM) complex (Yang et al., 2003). As described later, this complex is key to the conversion of the inactive zymogen protein C to its active form. The protein C anticoagulant system regulates thrombin formation by modulating the activity of the cofactors FVIIIa and FVa (Dahlback, 1995). This inhibitory system is composed of several components: protein C (PC), thrombomodulin (TM), endothelial cell protein C receptor (EPCR), and protein S (Table 10-6). Protein C, the key component, is a vitamin K-dependent, 62-kDa glycoprotein that is synthesized by the liver and circulates as a biologically inactive molecule consisting of a 40-kDa heavy chain and a 20-kDa light chain joined by a disulfide bond (Stenflo and Fernlund, 1982). As occurs with other vitamin K-dependent proteins, PC lacks biological activity unless it has undergone vitamin K-dependent posttranslational carboxylation of glutamine residues in the N-terminal region of the molecule. Thrombin cleavage of this N-terminal region converts PC to its proteolytic active form, APC (Esmon, 2003). For thrombin to activate PC at a physiologically relevant rate, it requires the presence of the cofactor thrombomodulin (TM) so that T-TM complexes can form. TM is a 58.7-kDa transmembrane glycoprotein that is present on vascular endothelial cells with the highest concentrations being present in microvascular beds (Esmon, 2003). In the presence of TM, the rate of PC activation is increased by more than 1000-fold (Van de Wouwer et al., 2004). The TM molecule is organized into five distinct domains. A short cytoplasmic tail at the C-terminus is followed by a membrane-spanning region that contains serine/threonine-rich domains that serve as glycosylation sites, which support its attachment to chondroitin sulfate (CS) in the extracellular matrix. The membrane-spanning domain is followed by a domain that consists of six epidermal growth factor (EGF)-like repeats, which determine the biological activity of TM. The N-terminal region of TM includes two amino acid sequences that play no role in its hemostatic function. Rather, the anticoagulant activity of TM is conferred by EGF domains 4, 5, and 6, which are also the areas that are involved in the ability of TM to enhance thrombin inhibition by PCI (Tsiang et al., 1992). The EGF5 and EGF6 domains have been identified as thrombin binding sites (Van de Wouwer et al., 2004). For APC to act efficiently as an anticoagulant, the presence of the cofactor, protein S, at the endothelial cell surface is also required. Protein S is a single-chain polypeptide that is synthesized by hepatocytes, endothelial cells, and megakaryocytes in forms that vary in size from 69 to 84 kDa (Johnstone, 2000). In plasma, the majority of protein S circulates bound to the complement regulatory protein C4b-binding protein and only about 30% is in the free, more biologically active form (Dahlback, 1995). Unlike the other vitamin K-dependent hemostatic proteins, protein S is not a zymogen and functions solely as a cofactor to promote the formation of the inhibitory complex that consists of APC-protein S-calcium on the negatively charged phospholipids (PL) that are exposed on damaged endothelium and activated platelets (Walker, 1984). These PLs are also those that are involved in the formation of the tenase complex and the prothrombinase complex (see Section II.C.3). Hence, protein S effectively acts as a mediator to increase the number of APC molecules bound to the PL, to bring them in closer contact with FVIIIa and FVa, and thus to accelerate the rate at which APC can cleave these proteins to produce their inactive forms, FVIIIai and FVai (Dahlback, 2000). The reduction in FVIIIa and FVa cofactor activity effectively reduces the rate of thrombin formation. APC can only inhibit FVIII when it is a component of the tenase complex because it is protected from proteolysis as FVIII circulates in plasma bound to vWF (see Section II.C.2). In contrast, circulating FV, like FVa, can bind to membrane PLs where it can be cleaved to generate an anticoagulant form of FV that functions in synergy with protein S as an APC cofactor in the inactivation of FVIIIa (Dahlback, 2000). In this way, FV can, like thrombin, function as both a procoagulant and an anticoagulant cofactor in hemostasis. It is now recognized that TM is not the only cofactor expressed on endothelial cells that can function as a cofactor for thrombin-induced PC activation. EPCR is constitutively expressed in endothelial cells, particularly in large blood vessels, and is structurally similar to the major histocompatibility class 1/CDI family of molecules that are involved in immunity and inflammation (Di Cera, 2003; Esmon, 2003; Van de Wouwer et al., 2004). Not only does EPCR accelerate thrombin-mediated activation of PC, but it also concentrates APC near the surface of the vessel wall (Stearns-Kurosawa et al., 1996). When APC is generated, it remains bound to EPCR for only a short time before it becomes associated with protein S on the surface of activated platelets or damaged endothelium (Van de Wouwer et al., 2004). After APC has caused the inhibition of FVIIIa and FVa, it is itself inactivated by several serpins including, α1-protease inhibitor, α2-M, and protein C inhibitor (Table 10-6). The APC pathway can also be down-regulated by inflammatory cytokines such as IL-1β and TNFαє, which reduce the expression of both TM and EPCR (Esmon, 2003). It would appear that TM can exert multifunctions in the regulation of hemostasis. For example, when TM is bound to CS on the extracellular matrix, not only is the PC cofactor activity of TM enhanced but the rate of neutralization of thrombin by both heparin-AT and by PCI is accelerated (Koyama et al., 1991). TM also exhibits antifibrinolytic activity through the ability of both EGF domains 3 through 6 of the thrombin-TM complex to activate TAFI (see Section II.D.3) (Nesheim, 2003). It is essential that the coagulation pathways are counterbalanced by a functional fibrinolytic cascade so that blood fluidity can be maintained in the vascular system (Welles, 1996). Like the coagulation pathway, the fibrinolytic system is normally latent but can be fully activated within a minute or two after stimulation (Nesheim, 2003). The major enzymatic component of this fibrin degradation system is plasmin, which, like thrombin, is a serine protease but with broader substrate specificity (Lijnen, 2002). In addition to cleaving specific sites on polymerized fibrin, under some pathophysiological conditions plasmin can also degrade fibrinogen, FV, and FVIII and activate metalloproteinases (Darien, 2000b). The fibrinolytic system shares several biochemical characteristics with the coagulation system. These include an initiation phase that involves the liberation of tissue-type plasminogen activator (tPA) from damaged endothelium; the conversion of the circulating zymogen, plasminogen, to the active protease, plasmin; and positive feedback reactions that increase the concentration of plasmin in the area of a clot (Fig. 10-3). The system is down-regulated by both protease inhibitors, such as plasminogen activator inhibitor type 1 (PAI-1) and antiplasmin (AP), and by a reaction mediated by the thrombin-TM complex (Table 10-6) (Coughlin, 2005a; Nesheim, 2003). The ability of fibrin to act as an effective cofactor for enhanced plasmin activity is an important factor in localizing the physiological activity of this protease to the site of a fibrin clot (Medved and Nieuwenhuzen, 2003). Nonphysiological activators of fibrinolysis, such as streptokinase, have only proved to be effective in dissolving fibrin clots in human plasma. FIGURE 10-3 Major components of the fibrinolytic system. Black lines represent activation reactions, and red lines represent inhibitory reactions. Abbreviations: AP, antiplasmin; PAI-1, plasminogen activator inhibitor-1; TAFI, tissue activatable fibrinolytic inhibitor; T-TM, thrombin-thrombomodulin complex; tPA, tissue-type plasminogen activator; scuPA, single-chain urokinase-type plasminogen activator; uPA, urokinase-type plasminogen activator. Plasminogen is secreted by the liver as a single-chain 92-kDa glycoprotein with glutamine as the N-terminal amino acid (Glu-plasminogen). It is composed of five kringle-like domains containing “lysine-binding sites” and a C-terminal domain homologous to other trypsin-like proteases (Ponting et al., 1992). The kringle domains mediate the interaction of plasminogen, and its active form plasmin, with substrates, inhibitors, and cell membranes. Consequently the kringle domains are important in both regulation of plasminogen activation and the localization of the proteolytic activity of plasmin to the appropriate physiological site (Wu et al., 1990). Plasminogen can be cleaved by plasmin at several sites resulting in lysine as the N-terminal amino acid (Lys-plasminogen) and the release of an 8-kDa N-terminal polypeptide. Lys-plasminogen, in the absence of fibrin, is converted to plasmin at a faster rate than Glu-plasminogen. The cleavage of an Arg-Val peptide bond in single-chain plasminogen converts it to plasmin, which now consists of two polypeptide chains linked by a disulfide bond (Dobrovolsky and Titaeva, 2002). The N-terminal heavy A chain contains the kringle domains that convey substrate specificity to plasmin, whereas the C-terminal light B chain contains the catalytic site. tPA is synthesized and secreted by endothelial cells as a 70-kDa single-chain active enzyme. It is the only protease of the hemostatic system secreted in an active form (Dobrovolsky and Titaeva, 2002). The molecule is composed of five domains: the N-terminal domain, which is homologous to the finger-like domains of fibronectin, is followed by a region homologous to the epidermal growth factor (EGF) domain, two kringle-like domains similar to those of plasminogen, and a C-terminal domain homologous to other trypsin-like proteases (van Zonneveld et al., 1986). The finger-like kringle domains possess two distinct fibrin-binding sites. Plasmin, kallikrein, and FXa can all cleave tPA to form a two-chain form of the molecule that, in the presence of fibrin, activates plasminogen at the same rate as single-chain tPA (Dobrovolsky and Titaeva, 2002). Stimuli such as exercise, venous occlusion, and intravenous injection of vasoactive drugs can increase the release of tPA from endothelial cells, whereas inflammatory mediators such as tissue necrosis factor α (TNFα) and interleukin-1 (IL-1) cause a decrease in free plasma tPA levels. Recombinant tPA is currently being used to induce thrombolysis in human patients with myocardial infarctions and other thromboembolic problems (Dobrovolsky and Titaeva, 2002). A trypsin-like protease capable of activating plasminogen was originally isolated from human urine and named urokinase. It is now known that many cell types can synthesize and secrete a single-chain 54-kDa glycoprotein, which was initially referred to as prourokinase. Current nomenclature designate these proteins as two forms: single-chain urokinase-type plasminogen activator (scuPA) and two-chain urokinase-type plasminogen activator (tcuPA). The scuPA molecule is composed of three domains. The N-terminal domain is homologous to the EGF domain, the middle protein is homologous to the kringle domain of plasminogen, and the C-terminal domain is homologous to trypsin-like proteases (Dobrovolsky and Titaeva, 2002). Both plasmin and kallikrein can cleave a Lys-Lys peptide bond in uPA to form fully functional tcuPA. Thrombin cleaves an Arg-Phe bond to produce a variant tcuPA molecule that appears incapable of directly activating plasminogen until after it has been further hydrolyzed by plasmin cleavage of a Lys-Ile bond (Dobrovolsky and Titaeva, 2002). Although initially considered to be a major component of the plasma fibrinolytic system, it is now recognized that a more important physiological function of uPA may be its ability to activate metalloproteinases that degrade cellular matrix components inducing pericellular proteolysis (Lijnen, 2002). uPA mediates this effect through its ability to bind to specific cellular receptors (uPARs), which bring uPA into close contact with cell-bound plasminogen and thus promote plasmin generation on the cell surface. Thus, uPAR plays an important role in cell migration and the tissue remodeling processes that are required following vascular damage and thrombus formation. The major inhibitor components of fibrinolysis are shown in Table 10-6. PAI-1 is the major endogenous inhibitor of fibrinolysis, or thrombolysis, as it is effective in blocking the conversion of plasminogen to plasmin by either tPA or uPA (Kruithof, 1998). PAI-1 is a 52-kDa-glycoprotein synthesized and secreted by endothelial cells in its active form. In plasma, PAI-1 is present in two forms: an active form in which the reactive site is exposed on the surface of the molecule and a latent form in which the reactive site is concealed within the protein globule (Dobrovolsky and Titaeva, 2002). The change of active PAI-1 to its latent form occurs spontaneously in plasma. Although the physiological mechanisms that induce the reverse transformation are not fully understood, it appears that latent PAI-1 can be reactivated by phospholipid vesicles containing PS or phosphatidylinositol (PI). This indicates that at sites of vascular injury, activated platelets may be able to activate latent PAI-1 (see Section II.B.2.a). Hence, the local concentration of active PAI-1 can be significantly increased at sites of thrombus formation as approximately 90% of PAI-1 circulates as one of the constituents of platelet α-granules. Thus, PAI-1, along with the action of thrombin activatable fibrinolysis inhibitor (TAFI, see Section II.D.4.c), is a contributing factor in the stabilization of the fibrin matrix. It has been shown in people that circulating PAI-1 levels vary more than any other component of the fibrinolytic system. This is likely due to the ability of a wide variety of substances to stimulate PAI-1 production. These include insulin, TNFα, IL-1, transforming growth factor β (TGFβ), and thrombin (Tsikouris et al., 2002). Endotoxin and TNFα have been shown to stimulate PAI-1 production in the liver, kidney, lung, and adrenals of mice. At least in human and murine plasma, PAI-1 exhibits the characteristics of an acute phase protein. In various disease states, a companion serine proteinase inhibitor of tPA known as plasminogen activator inhibitor type-2 (PAI-2) can appear in the circulation (Dobrovolsky and Titaeva, 2002). PAI-2 is synthesized by placenta, monocytes, and macrophages and is an effective inhibitor of t-PA. Antiplasmin (AP, also referred to α2-antiplasmin, α2AP) is the principal inhibitor of plasmin in the circulation. The liver secretes it as a single-chain 70-kDa protein. AP possesses the conserved core structure of the serpin family of proteins, and its amino acid sequence is highly conserved across species (Coughlin, 2005a). Human AP is 80% identical with the bovine protein and 74% identical with murine AP. In people, 30% of AP circulates in the form of Met-AP and 30% as Asn-AP. The latter is a truncated form that has lost a 12-amino acid sequence from the N-terminal end of the protein. Both Met-AP and Asn-AP inhibit plasmin at similar rates, but Asn-AP has a higher affinity for fibrin than Met-AP (Dobrovolsky and Titaeva, 2002). The N-terminal portion of AP is cross-linked to fibrin by a transglutaminase reaction catalyzed by FXIIIa. The amount of AP cross-linked to fibrin is a more important determinant of the rate of clot lysis than is the amount of free plasmin in the circulation (Coughlin, 2005a). The function of TAFI is to down-regulate the onset of fibrinolysis once the fibrin clot has successfully stopped blood loss. TAFI is a single-chain, 46-kDa plasma protein that is classified as a zinc-containing metalloproteinase (Bajzar, 2000). It can be cleaved by thrombin, plasmin, and trypsin to yield an activation peptide and an enzyme, TAFIa, that exhibits carboxypeptidase activity. Thrombin, by itself, is a relatively poor activator of TAFI, but in the presence of TM its catalytic efficiency is increased over 1000-fold (Nesheim, 2003). This enhancement of TAFI activation is dependent on the formation of a ternary complex between thrombin-TAFI and the EGF 3 through EGF 6 domains of the TM molecule. Like the thrombin-TM-mediated activation of PC, the activation of TAFI is calcium dependent (Bajzar et al., 1995). Plasmin is also a physiological activator of TAFI with a catalytic efficiency eight times that of thrombin. However, the ability of plasmin to activate TAFI is reduced to only one-tenth that of thrombin-TM in the presence of glycosaminoglycans, such as those found in the extracellular matrix (Mao et al., 1999). Although it is important that fibrinolysis occurs after wound healing has been initiated, it is equally important that the degradation of the clot not occur prematurely. This is achieved because, in the initial stages of thrombus formation, the thrombin-induced release of tPA from endothelial cells is effectively opposed by the localized activation of TAFI. Through its affinity for and its ability to modify partially degraded fibrin, TAFIa functions to block the ability of tPA to activate plasminogen (Fig. 10-3). Following partial degradation by plasmin, the newly exposed lysine residues of fibrin bind both tPA and plasmin with high affinity promoting fibrinolysis (see Section II.D.3). TAFIa catalyzes the release of both arginine and lysine residues from the partially degraded fibrin thus reducing both tPA and Glu-plasminogen binding, which has the effect of reducing plasmin formation. The reduced binding of Glu-plasminogen to TAFIa modified fibrin has been correlated with an increase in clot lysis time (Sakharov et al., 1997). Both TAFI and TAFIa are cross-linked to fibrin by FXIIIa, which localizes these molecules to the fibrin clot. TAFIa is an unstable molecule, which decays rapidly at 37°C such that its half-life is approximately 8 min (Nesheim, 2003). To date no physiological inhibitor of TAFI has been identified (Bajzar, 2000). PAI-1 is the major inhibitor of both tPA and uPA (Tsikouris et al., 2002). It inhibits plasminogen activation by first forming a reversible complex with tPA that then cleaves PAI-1 at its reactive site to release the C-terminal peptide of the inhibitor. Further proteolytic activity of tPA is blocked because the carboxyl group of one of the cleaved PAI-1 arginine residues remains covalently bound to a serine residue at the reactive center of tPA (Dobrovolsky and Titaeva, 2002). Because of the relatively high levels of PAI-1 in the circulation, most of the tPA released by endothelial cells is inactivated before it can interact with plasminogen. Hence, in the absence of fibrin plasminogen is only slowly converted to plasmin. Once tPA becomes bound to fibrin, it is protected from the inhibitory effects of PAI-1 and plasmin formation can proceed rapidly. Antiplasmin is the major physiological inhibitor of plasmin irrespective of whether the plasmin is bound to fibrin or is free in the circulation. In plasma, the reaction between AP and plasmin is rapid and the formation of a stable 1:1 bimolecular complex between the two molecules results in the irreversible inactivation of plasmin (Darien, 2000b). Because AP is partially degraded by plasmin, other circulating protease inhibitors (Table 10-6) can act as plasmin inhibitors, particularly when the capacity of AP is exceeded by high concentrations of free plasmin. These reactions are important in preventing systemic lytic states from developing such as occurs in certain disease states (see Section IV.B.4). When fibrin is formed, it binds plasminogen and AP in equimolar amounts. AP is bound via its N-terminal portion, which leaves the C-terminal portion free to bind to one of the kringle domains of the plasmin molecules that form in response to tPA activity (Coughlin, 2005a). This modulates plasmin such that the proteolytic cleavages do not initially destroy the fibrin network but rather open new affinity binding sites for additional tPA and plasminogen. As this first phase of fibrinolysis proceeds, more plasmin is generated on the partially degraded fibrin, which can induce the second phase that produces final degradation of the fibrin meshwork. The existence of the two sequential phases of fibrin degradation may be one of the mechanisms that provide temporary stability of fibrin clots at sites of vascular damage (Dobrovolsky and Titaeva, 2002). The common laboratory assays available to assess the integrity of the hemostatic system can be divided into those assessing primary hemostasis (platelet quantity and function) and those assessing secondary hemostasis (clotting factor activity and fibrin formation and dissolution). Proper collection of plasma that is free from clots and activated platelets is very important for all hemostasis testing. To obtain the best sample, minimal pressure should be placed on the vein and a single clean venipuncture performed, to avoid release of excessive TF, which would activate the clotting mechanism, consume coagulation factors, and therefore artifactually prolong the clotting times. The stopper should be removed from the Vacutainer and blood slowly expelled from the syringe into the tube because excessive vacuum turbulence may activate platelets. Ideally, the first few drops of blood collected should be discarded because they most likely would contain some TF. The blood should be gently mixed with the citrate, to ensure anticoagulation, but not too aggressively mixed so as to activate hemostasis. Several reports have compared the effect on various hemostatic parameters with respect to the concentration of citrate used and the volume of blood to citrate obtained. Historically, 3.8% sodium citrate tubes only were available, but recently 3.2% sodium citrate tubes have become the standard in Europe and are now available in the United States and elsewhere (Morales et al., 2007). One study determined that for some coagulation assays there can be significant differences between the two types of tubes (Stokol et al., 2000a), whereas another more recent study (Morales et al., 2007) suggests that there is no difference in any common coagulation assay between the two concentrations. It is recommended that each laboratory specify the citrate concentration preferred and develop reference intervals based on results from samples collected in the defined manner. The time a sample is stored before assessment may also have an impact on the test result. Most often samples are analyzed relatively quickly (i.e., within a few hours or at least the same day), but there may be an occasion when a sample cannot be assessed until the following day or two, or, depending on the assay, samples may be stored until sufficient numbers are collected and then run as a batch. Although longer than generally considered acceptable, a recent paper (Furlanello et al., 2006) found that samples could be kept at room temperature for up to 48 h without any significant change from immediate analysis, whereas storage at either room temperature or refrigeration was not recommended beyond 48 to 72 h. The typical recommendation is to perform assays within 4 h of sampling, after centrifuging and removal of plasma or to freeze the sample until analysis can be performed. Plasma samples can be safely stored at –70°C for up to 6 months with minimal change in results except for the aPTT (Bateman et al., 1999a; Iazbik et al., 2001). Platelets can be enumerated from either an EDTA or sodium citrate-anticoagulated blood sample. Heparinized blood is not suitable as marked platelet clumping often occurs. Assessment is best performed within 4 to 5 h, but the count may remain relatively stable for up to 24 to 48 h if the sample is refrigerated (Stockham and Scott, 2002). Manual or automated methods can be used for platelet quantity assessment. Manual methods include estimation of platelet quantity from a stained blood smear and hemacytometer chamber counting. Most automated hematology analyzers using impedance or light scattering technology can also enumerate platelets. Platelet clumping must be avoided for any platelet quantification method, or underestimation of the platelet count will occur. This can happen in any species with traumatic sampling and tends to occur most frequently in cats and cattle. Therefore, it is critical that a peripheral blood smear be examined for the presence of clumps; this also provides an opportunity to ensure appropriate cell morphology and to perform an estimate of platelet numbers as a cross-check of an automated determination. The mean platelet volume (MPV) is frequently determined by automated analyzers and reflects the average volume of all cells that are enumerated as platelets. For an accurate determination, platelet clumping needs to be avoided, and the cells should be unactivated and circular in shape without pseudopod formation. Variables such as time to analysis, storage temperature, and anticoagulant used can all impact MPV assessment. Generally, there tends to be an inverse relationship between platelet concentration and MPV; the value is higher when platelet concentration is low, and it is lower when numerous platelets are present. This has been documented in healthy cats (Weiser and Kociba, 1984.) An increase in MPV is a favorable finding in an animal with thrombocytopenia, as it is usually a reflection of active thrombopoiesis. Excessively large platelets are often observed in Cavalier King Charles spaniels, but the corresponding platelet concentration also tends to be lower, making overall platelet mass similar to other breeds (Smedile et al., 1997). Retraction of a blood clot depends on platelets, so defects in platelet quantity or quality will prolong the time required for whole blood to clot in a tube. Measurements of clot retraction are available, but this test is limited by subjectivity and is not often performed. Although typically assessed as an indicator of platelet function, a sufficient quantity of platelets also needs to be present to form a platelet plug in a standardized mucosal incision. Once thrombocytopenia is present, the BMBT may be prolonged simply from lack of platelets. See Section III.B.2.a for further details. The BMBT is an in vivo test of platelet function, which also depends on adequate platelet concentration and properly functioning endothelium. The incision is small enough that secondary hemostasis is not required to stop blood flow; formation of a platelet plug alone will stop the bleeding. A standardized incision using a commercially available device is made on the buccal mucosa of the lip and the time to clotting recorded in seconds or minutes. Time to clotting can vary substantially within and between observers, making this a less than ideal test, but most healthy dogs clot in less than 4 min (Sato et al., 2000). It is, however, useful as a quick and inexpensive screening test for congenital or acquired thrombopathia, which can be followed up by more specific testing if the result is prolonged. Skin and toenail bleeding time tests have also been described but are even less standardized than the BMBT and are not recommended. The propensity of platelets to bind to each other in the presence of certain agonistic substances can be exploited as a method to determine appropriate platelet function. Platelet aggregometers trace the optical density of a standardized platelet preparation after the addition of an aggregating substance such as ADP, epinephrine, or collagen. There are marked species differences with respect to concentration and type of agonist required to promote aggregation. Platelet aggregation is technically demanding, time consuming, and therefore generally limited to specialized or research laboratories. The Platelet Function Analyzer-100 (Bayer) has revolutionized assessment of platelet function in human medicine. The ability to quickly assess aperture closure time in a whole blood sample in a clinical setting has eliminated all the limitations of time-consuming and technically demanding platelet aggregometry. The PFA-100 simulates high shear flow blood vessel conditions and, with the addition of either epinephrine or ADP as an agonist on a collagen membrane, determines how quickly a platelet plug can close an aperture and arrest blood flow. The method has been evaluated for use in the dog (Couto et al., 2007), and the collagen/ADP cartridge has been found to be useful to screen for platelet dysfunction in von Willebrand disease (Mischke and Keidel, 2003), NSAID administration (Gaal et al., 2007; Neilsen et al., 2007), and during endotoxemia (Yilmaz et al., 2005). There are single reports evaluating the instrument for horses where it was determined it was sensitive enough to detect decreased platelet function related to ASA administration (Segura et al., 2005) and in a pig model of severe hemorrhagic shock (Arnaud et al., 2006). The one-stage prothrombin time (OSPT) assesses the plasma activity of prothrombin, FV, FVII, and FX after addition of calcium and activator known as tissue thromboplastin. The test therefore gives information about the tissue factor (extrinsic) pathway and the common pathway. Different sources of thromboplastin will result in different clotting times, and although the use of homologous tissue is ideal, human source reagents can be used effectively (Hall, 1970; Mischke and Nolte, 1997; Mischke et al., 2003). Clotting times tend to be rapid in domestic animals, often less than 10 sec, which reduces the sensitivity of the assay for detecting minor abnormalities. Dilution of reagents has been advocated as a way to extend clotting times to 10 to 15 sec. As a screening test, the OSPT is not sensitive to minor coagulation abnormalities, and it generally takes loss of>70% activity of one or more factors to prolong the clotting time. Modification of the test to assess individual factor activity can be achieved using specific factor deficient plasma, and several assays have been shown to have similar ability to detect factor deficiencies (Mischke, 2002). The effects of common interferences (lipid, hemoglobin, bilirubin) on the PT assay have been studied (Moreno and Ginel, 1999). These authors found that bilirubin caused a statistically significant prolongation of the OSPT but that clinical significance associated with this prolongation was unlikely. Point-of-care analyzers are now available that can quickly perform the OSPT at the animal’s cage side and have been evaluated in dogs (Tseng et al., 2001). The International Normalized Ratio (INR) is frequently used in place of the OSPT in human medicine for monitoring people on warfarin anticoagulant therapy because different thromboplastins have variable sensitivities to warfarin-induced changes in factor activity (Stockham and Scott, 2002). The International Sensitivity Index (ISI), which is specific for an individual reagent, is incorporated into the INR calculation and is meant to correct for reagent variation. ISI values are not widely available for domestic animal species, so the INR is not frequently used in veterinary medicine. The activated partial thromboplastin time (aPTT) assay detects fibrin clot formation after addition of an activating agent, partial thromboplastin (phospholipids that lack TF), and recalcification of plasma. It assesses the activity of prothrombin, FV, FVIII, FIX, FX, FXI, and FXII, therefore providing information about the integrity of the contact activation (intrinsic) and common pathways (see Section II.C.3). Activating agents include substances such as kaolin and ellagic acid, and clotting times can vary between species and depending on the specific activators used. Pooled plasma from a similar species is required as a control to avoid interpretive errors. There can be significant variations in the assay method and reagents used, so it is crucial that each laboratory define its own species-specific reference interval (Evans and Flynn, 1992; Johnstone, 1984). Similar to the OSPT, the test is relatively insensitive, requiring loss of activity of 70% of at least one factor before prolongation of the clotting time. Common interferences (lipid, hemoglobin, bilirubin) may interfere with an optical method and provide an erroneous result. Hemoconcentration was demonstrated to prolong aPTT in one study, emphasizing the importance of the blood-anticoagulant ratio for accurate testing (O’Brien et al., 1995). The aPTT can additionally be used to monitor cats and dogs receiving heparin therapy (Greene and Meriwether, 1982; Mischke, 2003). The assay can be modified to detect specific factor deficiencies (Deniz et al., 1995; Mischke, 2000). The activated clotting time (ACT) is similar to the aPTT in that it provides information about the same portions of the clotting mechanism (intrinsic or common pathways), but it is a less sensitive test. It is a point-of-care test performed on whole blood, where a compound in the tube, typically siliceous earth, activates the contact (intrinsic) pathway (Stockham and Scott, 2002). It depends on endogenous platelets in the sample as the phospholipid source for clotting. Because of this, although not well documented, severe thrombocytopenia may result in a prolonged ACT. It is used primarily as a screening test for coagulopathies, but it requires loss of greater than 95% loss of activity of one or more factors before it is prolonged, so disease is often advanced by the time the ACT is abnormal. The ACT is the time in seconds to first visible clot formation while gently inverting the tube after initial incubation for 60 sec at 37°C either in a heating block or in a human axilla. The ACT is used in humans and sometimes in animal patients to monitor therapeutic heparinization. Reference values have been published for cats (Bay et al., 2000), but each facility should follow a standard protocol and ideally define its own reference interval. Automated ACT methods are available on some point-of-care instruments that offer OSPT and aPTT, but they are not exactly the same as the tube ACT (phospholipid is added) and provide no advantage over an automated aPTT (Tseng et al., 2001). The thrombin clotting time (or simply thrombin time) assesses the ability of thrombin to convert fibrinogen to fibrin, so it is abnormal when there are quantitative or qualitative abnormalities in fibrinogen. Variations of the test are available, so a reference interval specific for the laboratory and species should be developed (Mischke and Jacobs, 2001). The Clauss method is specifically used to derive a concentration of fibrinogen in a plasma sample (see Section III.B.5.b). Fibrin-fibrinogen degradation product (FDP) assays measure the breakdown split products of either fibrinogen or fibrin, and increased values indicate enhanced fibrinogenolysis or fibrinolysis. Elevated FDPs are used to help confirm the presence of DIC (see Section IV.B.4). Several human assays are available, including both serum and plasma-based assays, but the plasma latex agglutination test appears more sensitive for detecting DIC in dogs (Boisvert et al., 2001; Stokol et al., 1999). FDP assays have also been used in cats and horses. D-dimers and x-oligomers are small fragments created when fibrinolysis occurs. They are products of fibrin degradation, not fibrinogen degradation, so are more specific for enhanced fibrinolysis. There are currently no available animal-specific assays, but there appears to be sufficient cross-reactivity between human and canine proteins as some human-based semiquantitative immunological latex agglutination methods have been used in canine patients (Griffin et al., 2003; Nelson and Andreasen, 2003; Stokol, 2003; Stokol et al., 2000b). D-dimers have been assessed in horses, predominantly in an attempt to document DIC related to colic (Dallap et al., 2003; Heidmann et al., 2005; Monreal, 2003; Sandholm et al., 1995). Specific factor activity is traditionally assessed using coagulometric methods. Depending on the factor, modifications of the OPST (for extrinsic or common pathway factors) or the APTT (for intrinsic factors) are used where the activity of the factor in patient plasma is measured in a sample mixed with plasma known to be deficient in the factor. If the patient sample is deficient in the factor in question, the OSPT or APTT, as appropriate, does not correct (Stockham and Scott, 2002). The activity of the factor in the patient’s plasma is derived from a standard curve created from serial dilutions of pooled homologous plasma from clinically healthy animals that has been added to the factor-deficient plasma. Factor-deficient plasmas for domestic animal species are not generally available, so methods must be validated using human reagents. Chromogenic methods are now available and used quite extensively in human medicine. However, the expense, the necessity to use a 96-well plate, and the limited shelf life of reagents once the kit is opened limit extensive use of this methodology for veterinary medicine unless samples can be batched. For the heat precipitation method, EDTA-anticoagulated blood is centrifuged in a microhematocrit tube and heated for 3 to 9 min at 56°C, and then the amount of precipitate formed is quantified as a measure of fibrinogen. This method is useful only as a crude estimate of an increased concentration of fibrinogen and is no longer routinely used. Derivation of fibrinogen concentration from the Clauss thrombin clotting time (Stockham and Scott, 2002) is more often performed at veterinary labs, because routine fibrin-based clotting assays are usually offered. Fibrinogen concentration is inversely proportional to the time it takes for thrombin to form fibrin from fibrinogen, so it depends on the amount of fibrinogen present when excessive thrombin is used to negate the effects of any inhibitors such as heparin or fibrin degradation products (Stockham and Scott, 2002). The time to clot formation is recorded in seconds and then converted to mg/dl or g/l based on a standard curve. A fibrinogen antigen assay is available but is not a functional test. Therefore, if the antigen assay is not decreased but the TCT is abnormal, dysfibrinogenemia is likely. Assessment of von Willebrand factor (vWF) protein is most commonly determined by detecting antigen concentration in plasma, but additional tests are available if more detailed information is needed to manage the animal. See Section IV.A.1.b for more details. As an indicator of anticoagulant status, there are both functional and antigenic assays for the detection of antithrombin (AT) in plasma. Functional assays can be either thrombin or FXa based, where the more AT there is, the less activity of either of these enzymes there will be. A standard curve from homologous pooled plasma must be prepared to determine the test plasma activity. Both manual and automated methods are available. The activity of AT is stable, and in one study values remained relatively unchanged after 6 months of storage of plasma at–70°C and after 6 weeks of storage of whole blood at 4°C (Green, 1988). The same study suggested that FXa-based assays may be more appropriate because heparin cofactor II activity may be measured in some thrombin-based assays and falsely elevate the AT measurement. Hemoglobin in the sample may interfere with the ability to accurately determine plasma AT activity in some chromogenic assays (van der Merwe and Reyers, 2007). Several additional assays of activation of coagulation and fibrinolysis have been examined on a limited basis in veterinary medicine (Roncales and Sancho, 2000). Most of these tests are used widely in human medicine but need more thorough evaluation and standardization before being offered as routine tests for domestic animals. Some of the proteins or complexes tested include activated clotting factors such as FVIIa and FVIIa, activation peptides such as fibrinopeptides A and B, and indicators of fibrinolysis such as plasmin-antiplasmin complexes and PAI-1. Thrombin-antithrombin complexes (TAT) have been measured as an indicator of coagulation activation in dogs with malignant neoplasia (Maruyama et al., 2005) and horses with colic (Topper and Prasse, 1996). The Russell viper venom time (RVVT) test is an additional assay used occasionally to provide information about common pathway abnormalities (Stockham and Scott, 2002). The time to clot formation depends on activation of FX by the venom of a Russell’s viper. This test is not routinely used. Proteins induced by vitamin K antagonism or absence (PIVKA) are inactive vitamin K-dependent clotting factors produced by hepatocytes when this important cofactor is unavailable (see Section IV.B.5). The Thrombotest PT is a modification of the prothrombin time that is sensitive, but not entirely specific, to the presence of these abnormal proteins (Center et al., 2000). Thromboelastography (TEG) provides a global assessment of hemostasis and specifically provides general information about the rate of fibrin polymerization and overall clot strength. Analysis of the TEG tracing can give a summary of platelet function, coagulation proteins, and fibrinolysis (Luddington, 2005). TEG has traditionally been used in human medicine to facilitate blood product use decisions in patients undergoing liver transplant or cardiac surgery. Its use has been minimally evaluated in veterinary medicine (Donahue and Otto, 2005). One study used TEG to document hypercoagulability in dogs with parvoviral enteritis (Otto et al., 2000). Routine use of TEG suffers from lack of standardization, although a group recently developed TEG values for clinically healthy dogs (Wiinberg et al., 2005). Although von Willebrand disease (vWD) is the most common inherited bleeding disorder in both humans and dogs, it has also been reported in swine and cats (Denis and Wagner, 1999; Thomas, 1996). Human vWD was described initially in the 1920s by Erik von Willebrand, and since then it has been classified into three distinct variants (Ewenstein, 1997). Two are quantitative deficiencies (types I and III), with a decrease in circulating vWF, and one is a qualitative defect (type II) (Ewenstein, 1997). Human type II vWD is further divided into four subtypes (A, B, M, and N) (Ewenstein, 1997). The first incidence of vWD in dogs was reported in 1970 in a family of German shepherd dogs (Denis and Wagner, 1999). Since then, as in humans, three types of vWD have been identified and are summarized in Table 10-7. Whereas types I and III vWD share phenotypic characteristics with the respective human types, the qualitative defect (type II) is characterized by a decrease in high-molecular-weight vWF multimers without further subclassification. Although modes of inheritance have been identified, the actual genetic defect is unknown for many of the affected breeds (Denis and Wagner, 1999).
I. INTRODUCTION
II. MECHANISMS OF HEMOSTASIS
A. Role of Vascular Endothelium
Antithrombotic Properties
Prothrombotic Properties
Prostacyclin (PGI2)
Tissue factor release/expression
Nitric oxide
von Willebrand factor
Thrombomodulin
Plasminogen activator inhibitor-1 (PAI-1)
Tissue factor pathway inhibitor (TFPI)
Factor V
Heparan sulfate
Platelet activating factor
Tissue plasminogen activator
P-selectin expression
B. Platelets
1. Platelet Production
2. Platelet Structure
Effector
Major Actions
Tyrosine and serine/threonine kinases (e.g., Src, Syk, Erk, PKC)
Enzyme activation including PLC, PLA2, MLCK
↑ intracellular calcium
Calmodulin mediated activation of MLCK
Activation of PLC, PLA2, PKC
Activation of phosphodiesterase → ↓ cAMP
PLCα, PLCβ
Hydrolysis of PI to IP3 and DAG
IP3
Activation of calcium-dependent ATPase → release of calcium from dense granules
DAG
Activation of PKC → integrin conformation changes → ↑ affinity of GPIIb-IIIa for fibrinogen
PLA2
Hydrolysis of PC → release of AA and lyso-PAF
3. Platelet Activation
a. Immobilized Agonists
b. Soluble Agonists
c. Intracellular Signaling Mechanisms
d. Negative Regulation of Aggregation
Effector
Major Actions
PGI2
↑ adenylyl cyclase activity → ↑ cAMP
Inhibition of PI hydrolysis → ↓ IP3 and ↓ DAG
cAMP
↓ free cytosolic calcium
Inhibition of PI hydrolysis
Suppression of adhesion and aggregation responses
NO/cGMP/cGKI
Inhibition of ADP2Y12 receptor activation
Inhibition of GPIIb-IIIa receptor activity
PECAM-1
Inhibition of collagen stimulated calcium
Mobilization, granule secretion, and aggregation
C. Coagulation Proteins, Complexes, and Thrombin Activation
1. Overview
Traditional Designation
Alternate Name(s)
Factor I
Fibrinogen
Factor II
Prothrombin
Factor III
Tissue factor
Factor V
Proaccelerin, accelerator globulin
Factor VII
Proconvertin
Factor VIII
Antihemophiliac factor
Factor IX
Christmas factor
Factor X
Stuart factor
Factor XI
Plasma thromboplastin antecedent
Factor XII
Hageman factor
Factor XIII
Fibrin stabilizing factor
Prekallikrein
Fletcher factor
2. Complexes of the Procoagulant System
a. Tissue Factor-FVIIa Complex
b. Tenase Complex
c. Prothrombinase Complex
3. Mechanisms of Thrombin Formation
4. Fibrin Clot Formation
5. Inhibitors of Coagulation
a. Tissue Factor Pathway Inhibitor (TFPI)
b. Serine Protease Inhibitors (Serpins)
c. Protein C Anticoagulant System
D. Fibrinolysis
1 Overview
2. Components of the Fibrinolytic System
a. Plasminogen
b. Plasminogen Activators
3. Mechanism of Fibrinolysis
4. Inhibitors of Fibrinolysis
a. Plasminogen Activator Inhibitor Type-1 (PAI-1)
b. Antiplasmin (AP)
c. Thrombin Activatable Fibrinolytic Inhibitor (TAFI)
5. Regulation of Plasminogen Activation and Plasmin Activity
III. LABORATORY ASSESSMENT OF HEMOSTASIS
A. Quality Control and Reagent Variation
B. Testing of Hemostasis
1. Assessment of Platelet Quantity
a. Platelet concentration and volume
b. Clot Retraction
c. Buccal Mucosal Bleeding Time
2. Assessment of Platelet Quality
a. Buccal Mucosal Bleeding Time
b. Platelet Aggregometry
c. PFA-100
3. Screening Tests of Hemostasis
a. OSPT
b. aPTT
c. ACT
d. TCT
4. Assessment of Fibrinolysis
a. FDPs
b. D-dimer
5. Assessment of Specific Hemostasis Proteins
a. Prothrombin, FV, FVII, FVIII, FIX, FX, FXI, FXII
b. Fibrinogen
c. von Willebrand Factor
d. Antithrombin
C. Additional Assays
IV. DISORDERS OF HEMOSTASIS
A. Hereditary Disorders
1. Von Willebrand Disease
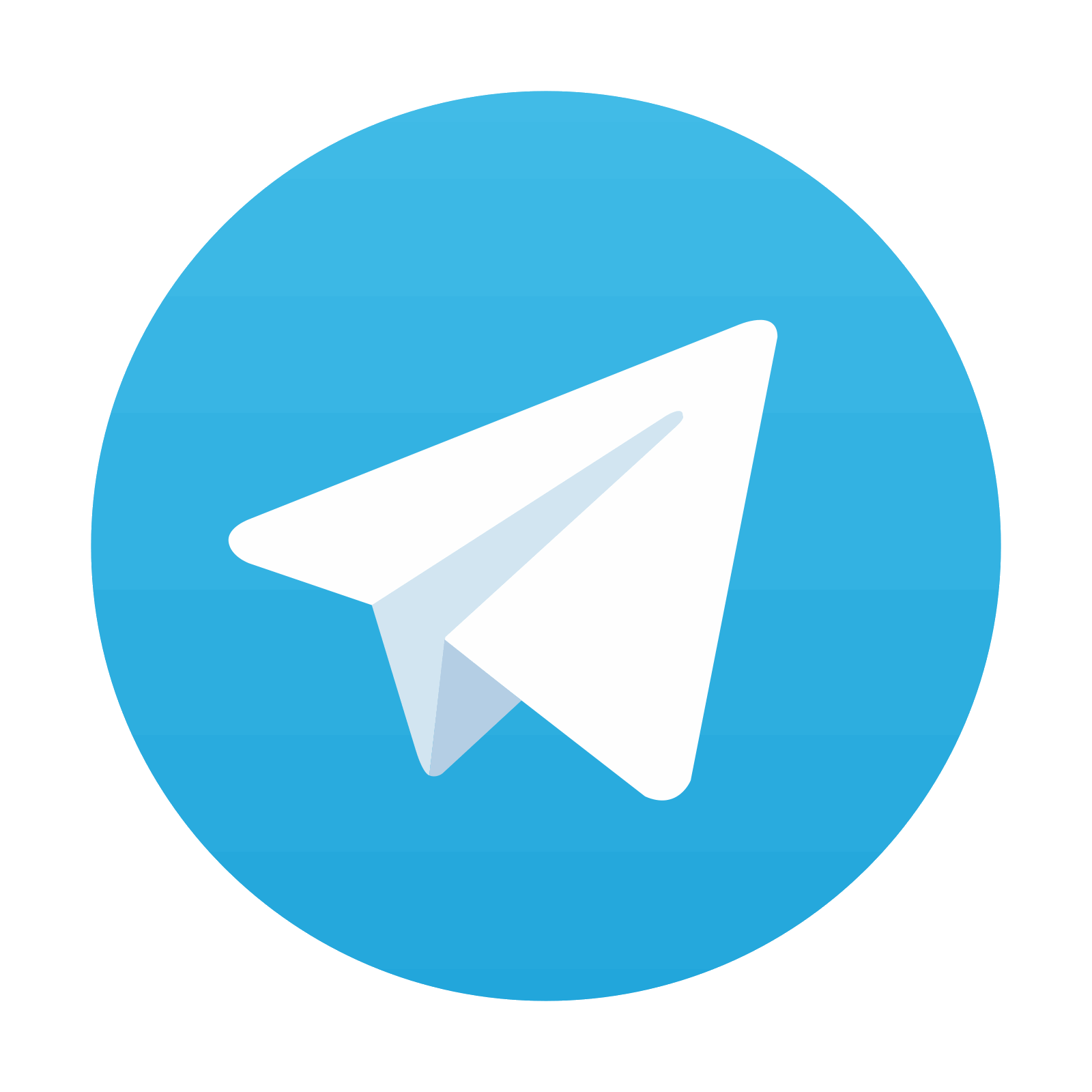
Stay updated, free articles. Join our Telegram channel
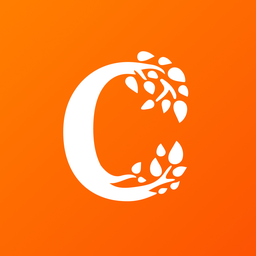
Full access? Get Clinical Tree
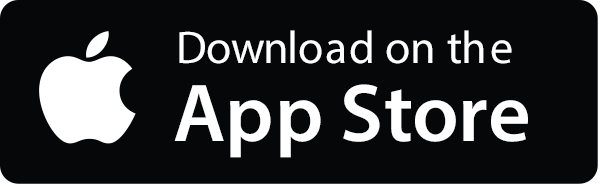
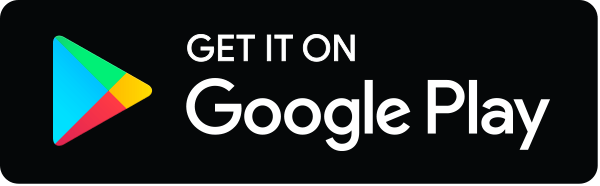