Chapter 3 Carbohydrate Metabolism and Its Diseases IV. METABOLISM OF ABSORBED CARBOHYDRATES V. INTERRELATIONSHIPS OF CARBOHYDRATE, LIPID, AND PROTEIN METABOLISM B. Influence of Glucose Oxidation on Lipid Metabolism VI. INSULIN AND CARBOHYDRATE METABOLISM D. Insulin Action on Biochemical Systems E. Physiological Effects of Insulin F. Other Pancreatic Islet Hormones VII. BLOOD GLUCOSE AND ITS REGULATION B. Indirect Monitoring of Blood Glucose IX. DISORDERS OF CARBOHYDRATE METABOLISM X. DISORDERS OF RUMINANTS ASSOCIATED WITH HYPOGLYCEMIA C. Biochemical Alterations in Body Fluids The biochemical mechanisms by which the chemical energy contained in foodstuffs are made available to the animal are collectively described as metabolism. Thus, the description of the metabolism of a foodstuff encompasses the biochemical events that occur from the moment of ingestion to its final breakdown and excretion. Classically, these biochemical events have been divided into the metabolism of the three major constituents of food; carbohydrates, proteins, and lipids. The metabolism of the lipids and proteins is discussed in their individual chapters. The major function of ingested carbohydrates is to serve as energy sources, and their storage function is relatively minor. Carbohydrates are also precursors of essential intermediates for use in synthetic processes. When the metabolic machinery of an animal is disrupted, a disease state prevails (e.g., diabetes). The literature of the biochemistry of metabolism and disease continues to expand as the minute details of individual and overall reaction mechanisms are continually clarified. Additionally, modern molecular approaches have significantly increased our understanding of disease mechanisms and remain fertile fields for investigations into the disease processes. This chapter presents a basis for the better understanding of the biochemical mechanisms underlying those diseases associated with carbohydrate metabolism. The intricate details of carbohydrate metabolic reactions may be found in the many books on fundamental biochemistry. The digestion of carbohydrates in the animal begins with the initial contact of the carbohydrates in their foodstuffs with the enzymes of salivary juice. Starch of plant foods and glycogen of meat are split into their constituent monosaccharides by the action of amylase and maltase. This activity ceases as the food matter passes into the stomach, where the enzymatic action is destroyed by the hydrochloric acid. Within the stomach, acid hydrolysis may occur, but the stomach empties too rapidly for complete hydrolysis to take place. Thus, only a small portion of the ingested carbohydrate is hydrolyzed before passing into the small intestine. In the small intestine, digestion of carbohydrate takes place quickly by the carbohydrate splitting enzymes contained in the pancreatic juice and in the succus entericus. Starch and glycogen are hydrolyzed to glucose by amylase and maltase; lactose to glucose and galactose by lactase; and sucrose to glucose and fructose by sucrase. The monosaccharide products of enzymatic hydrolysis of carbohydrates, glucose, fructose, and galactose, are the principal forms in which absorption occurs in the monogastric animal. The monosaccharides are almost completely absorbed through the mucosa of the small intestine and appear in the portal circulation as the free sugars. Absorption into the mucosal cell occurs by a sodium-dependent active transport mechanism using a glucose co-transporter. The absorbed glucose then leaves the mucosal cell by a facilitated diffusion process in the presence of a glucose transporter, GLUT-2. Glucose and galactose are absorbed rapidly, whereas fructose is absorbed at about half the rate of glucose with a portion being converted to glucose in the process. Other monosaccharides (e.g., mannose) are absorbed slowly at a rate consistent with a diffusion process. As a result, free sugars appear in the portal circulation for transport to the liver. Glucose transporters (GLUT) are known to be involved in many tissues—for example, GLUT-1 is the hepatocyte/RBC transporter and is widely distributed; GLUT-2 is in hepatocytes, β cells, and mucosal cells; GLUT-3 is the brain transporter; GLUT-4 is the only insulin-responsive glucose transporter; GLUT-5 is in the intestine; GLUT-6 is not available; and GLUT-7 is within cell organelles (Winter and Signorino, 2002). Glucose transporters have been identified and numbered beyond GLUT-8 and up to GLUT-12 (Piroli et al., 2004) but remain to be confirmed. Liver cells are readily permeable to glucose. This process is facilitated by glucose transporter (GLUT) proteins within the plasma membrane, in particular, GLUT-2 is the transporter in the liver cell plasma membrane (Thorens et al., 1988). Within the liver, there are several pathways by which the immediate fate of the hexoses is determined. Glucose, fructose, and galactose first enter the general metabolic scheme through a series of complex reactions to form glucose phosphates (Fig. 3-1). The enzyme, galactose-1-P uridyl transferase, which catalyzes the reaction FIGURE 3-1 Pathways for hexose metabolism. Abbreviations: ATP, adenosine triphosphate; UTP, uridine triphosphate; UDP-G, uridine diphosphate glucose; DHAP, dihydroxy acetone phosphate; GA-3-P, glyceraldehyde-3-phosphate. is blocked or deficient in congenital galactosemia of humans. The glucose phosphates are then converted to and stored as glycogen, catabolized to CO2, and water or, as free glucose, returned to the general circulation. Essentially, intermediate carbohydrate metabolism of animals evolves about the metabolism of glucose, and the liver is the organ of prime importance. Glycogen is the chief storage form of carbohydrate in animals and is analogous to the storage of starch by plants. It is found primarily in liver and in muscle, where it occurs at about 3% to 6% and about 0.5%, respectively (Table 3-1). Glycogen is composed solely of α-D-glucose units linked together through carbon atoms 1 and 4 or 1 and 6. Straight chains of glucose units are formed by the 1–4 links and these are cross-linked by the 1–6 links. The result is a complex ramification of chains of glucosyl units with branch points at the site of the 1–6 links (Fig. 3-2). The internal chains of the glycogen molecule have an average length of four glucosyl units. The external chains beyond the last 1–6 link are longer and contain between 7 and 10 glucose units. The molecular weight of glycogen may be as high as 4 × 106 Mr and contain about 20,000 glucosyl units. FIGURE 3-2 Glycogen structure. Note that hydrolysis of a 1–6 link by the debrancher enzyme yields a mole of free glucose. In Table 3-2, the amount of carbohydrate available to meet the theoretical requirements of a hypothetical dog is shown. The amount present is sufficient for about half a day. It is apparent that the needs of the body that must be met continually are satisfied by alternate means and not solely dependent on continuous ingestion of carbohydrates. During and after feeding (postprandial), absorbed hexoses are converted to glucose by the liver and enter the general circulation. Excesses are stored as glycogen or as fat. During the fasting or postabsorptive state, glucose is supplied by the conversion of protein (gluconeogenesis) and by the breakdown of glycogen (glycogenolysis). The continued rapid synthesis and breakdown of glycogen, (i.e., turnover) is well illustrated by the biological half time of glycogen, which is about a day. TABLE 3-2 Carbohydrate Content of a Doga aBody weight, 10kg; liver weight, 300g; muscle weight, 5 kg; volume of blood and extracellular fluid, 2.2 liters. The initial reaction required for the entrance of glucose into the series of metabolic reactions, which culminate in the synthesis of glycogen, is the phosphorylation of glucose at the C-6 position. Glucose is phosphorylated with adenosine triphosphate (ATP) in liver by an irreversible enzymatic reaction catalyzed by a specific glucokinase (GK): Glucokinase (GK) (also called hexokinase-IV [HK-IV]) is one of the four hexokinase isoenzymes that occurs in all tissues. Glucokinase (GK) or HK-IV, which is glucose specific, is the predominant isoenzyme found in liver. The nonspecific hexokinase-I (HK-I) is the isoenzyme found in red cells, brain, and nerve tissue. Liver contains both GK (HK-IV) and HK-I, but GK is the predominant isoenzyme. GK has a high Michaelis constant (Km = 2 × 10–2 mol G/l) indicating a low affinity for glucose. The rate of the phosphorylation reaction catalyzed by GK is therefore controlled by the glucose concentration. The activity of GK is increased by glucose feeding and by insulin and is decreased during fasting and in insulin lack (i.e., diabetes). In this regard, GK is an inducible enzyme whose activity is increased by glucose or by insulin. The nonspecific HK-I is found in all tissues including liver, brain, and erythrocytes and has a low Michaelis constant (Km = 5 × 10–5 mol G/l), indicating a high affinity for glucose. HK-I catalyzes phosphorylation in all tissues, therefore, it is not controlled by glucose concentration. The activity of HK-I is not affected by fasting or by carbohydrate feeding, diabetes, or by insulin. Therefore, in contrast to GK, HK-I is not an inducible enzyme. Glycogen is synthesized from this glucose-1-phosphate (G-1-P) through a series of reactions involving the formation of uridine derivatives. Uridine-di-phosphoglucose (UDP-G) is synthesized by the transfer of glucose from G-1-P to uridine triphosphate (UTP). This reaction is catalyzed by the enzyme UDP-G-pyrophosphorylase (UDP-G-PPase): In the presence of a polysaccharide primer and the enzyme glycogen synthase (glucosyl transferase), the glucose moiety of UDP-G is linked to the polysaccharide chain by an α-1–4 link: Through repeated transfers of glucose, the polysaccharide chain is lengthened. When the chain length of the polysaccharide reaches a critical level between 11 and 16 glucosyl units, the brancher enzyme, α-glucan glycosyl 4:6 transferase, transfers the terminal 7 residue portion from an α-1–4 linkage to an α-1–6 linkage. The newly established 1–6 linkage thus becomes a branch point in the expanding glycogen molecule. The remaining stub can again be lengthened by the action of glycogen synthase. Approximately 7% of the glucose units of the glycogen molecule are involved in these branch points. The breakdown of liver glycogen to glucose (glycogenolysis) takes place via a separate pathway. The key initiating and regulating factor in glycogenolysis is the action of epinephrine on liver and muscle glycogen and of glucagon on liver glycogen only. The mechanism of action of glucagon and epinephrine is through a series of reactions that culminate in the phosphorolytic cleavage of the 1–4 glucosyl links of glycogen. In the liver cell, glucagon and epinephrine stimulate the enzyme adenylate cyclase to form 3′–5′ cyclic adenosine monophosphate (cAMP) from ATP. cAMP in turn activates a protein kinase, which in its turn activates liver phosphorylase (LP), the phosphorolytic enzyme. As with many enzymes, LP is present in an inactive form, dephospho-liver phosphorylase (dLP), which is converted to its active form, LP (Cherrington and Exton, 1976) by the protein kinase, phosphorylase kinase. The action of the LP is to cleave the 1–4 glucosyl links of glycogen by the addition of orthophosphate in a manner analogous to a hydrolytic cleavage with water, hence the analogous term “phosphorolysis.” Phosphate is added to the C-1 position of the glucose moiety while H+ is added to the C-4 position of the other. cAMP is also a key regulating factor in cellular processes in addition to LP activation. It is required for the conversion of inactive muscle phosphorylase b to active muscle phosphorylase a, again via phosphorylase b kinase. The actions of other hormones known to be mediated by activating adenylate cyclase and cAMP include ACTH, LH, TSH, MSH, T3, and insulin. From these findings, a general concept of hormone action has evolved in which the hormone elaborated by the endocrine organ is described as the first messenger and cAMP within the target cell is the second messenger. Glucagon acts only on liver glycogen whereas epinephrine acts on both liver and muscle glycogen. In liver, glucagon promotes the formation and release of glucose by increasing glycogenolysis and decreasing glycogenesis. In liver, the hydrolysis of G-6-P is catalyzed by the enzyme glucose-6-phosphatase (G-6-Pase) to release free glucose, thus promoting hyperglycemia. Additionally, glucagon promotes hyperglycemia by stimulation of hepatic gluconeogenesis and thus glucagon is a potent hyperglycemic factor. With muscle glycogen, however, because the enzyme G-6-Pase is absent from muscle, glycogen breakdown in muscle results in the production and release of pyruvate and lactate rather than glucose. Mainly lactate and some pyruvate are transported to the liver where glucose is resynthesized via reverse glycolysis (Cori cycle; Section IV.D). The continued action of LP on the 1–4 linkages results in the sequential release of glucose-1-P (G-1-P) units until a branch point in the glycogen molecule is reached. The residue is a limit dextrin. The debrancher enzyme, amylo-1-6-glucosidase, then cleaves the 1–6 linkage, releasing free glucose. The remaining 1–4 linked chain of the molecule is again open to attack by LP until another limit dextrin is formed. Thus, by the combined action of LP and the debrancher enzyme, the glycogen molecule is successively reduced to G-1-P and free glucose units. G-1-P is converted to G-6-P by the reversible reaction catalyzed by phosphoglucomutase (PGM, Section IV.C.1, Reaction II). The G-6-P is then irreversibly cleaved to free glucose and phosphate by the enzyme G-6-Pase, which is found in liver and kidney. The free glucose formed can, unlike its phosphorylated intermediates, be transported out of the hepatic cell and enter the general circulation, thereby contributing directly to the blood glucose pool. In muscle tissue, there is no G-6-Pase, and muscle glycogen cannot supply glucose directly to the circulation by glycogenolysis. Muscle glycogen contributes to blood glucose indirectly via the lactate or Cori cycle (Section IV.D). The series of reactions described are illustrated schematically in Figure 3-3. FIGURE 3-3 Summary of liver glycogen metabolism. In muscle, phosphorylase a is the active form and phosphorylase b is the inactive form. Abbreviations: UDP, uridine diphosphate; LP, liver phosphorylase. The biochemical basis of the glycogenolytic and hyperglycemic action of glucagon and epinephrine was discussed in Section IV.C.2. These hormone actions are the bases for the epinephrine and glucagon stimulation tests, which are used to assess the availability of liver glycogen and the sensitivity of the carbohydrate regulatory mechanisms to these hormones. Many other hormones influence carbohydrate metabolism to a greater or lesser degree in keeping with the concept that carbohydrate metabolism is a totally integrated metabolic mechanism. One of the results of successful insulin therapy is a restoration of the depleted glycogen reserve. The mechanism of insulin action on carbohydrate metabolism continues to be a subject for intense study and is discussed more fully in Section VI. Briefly, the primary role of insulin is to promote glucose entry into peripheral cells, mainly muscle and fat cells, and to enhance glucose utilization by liver cells by its effect on enzyme systems at control points in the glycolytic pathways. In the presence of insulin, glucose removal from the blood is enhanced by shifting the direction of glucose metabolism toward utilization by increasing glycogen synthesis and glucose uptake and oxidation. The result is a hypoglycemia. Promotion of liver glycogen storage is also one of the effects of the glucocorticoids. This effect may be attributed to their enhancement of gluconeogenesis, hyperglycemia, decreased glycogenolysis, and decreased glucose oxidation. A tendency toward a mild hyperglycemia is also present in hyperthyroid states, as the result of an overall increase in carbohydrate metabolism. Thyroxine is thought to increase the sensitivity of the liver cell to the action of epinephrine, thereby increasing glycogenolysis and promoting hyperglycemia. Increased glycogenolysis, gluconeogenesis, and the hyperglycemia may also be the compensatory result of an increased rate of tissue metabolism. In rats made hyperthyroid, hepatic G-6-Pase activities are increased, which would enhance hepatic glucose production and hyperglycemia in the hyperthyroid states. An additional factor contributing to the overall tendency for hyperglycemia is the stimulation of glucose absorption by the gastrointestinal tract by thyroxine. In systemic disease, changes in glycogen concentrations in tissues or organs are generally observed as decreases. Depletion of liver glycogen stores is seen in diabetes mellitus, starvation, bovine ketosis, ovine pregnancy toxemia, or in any condition with nutritional carbohydrate deficiency or increased carbohydrate turnover. Pathological increases in liver glycogen occur in the rare glycogen storage diseases (GSD) and are described in Section IX.D. Carbohydrate in the form of glucose is the principal source of energy for the life processes of the mammalian cell. All cells require a constant supply of this indispensable nutrient, and only relatively small changes are tolerated without adverse effects on the health of the animal. Glucose is not oxidized directly to CO2 and H2O but rather through a series of stepwise reactions involving phosphorylated intermediates. The chemical energy of glucose is “stored” through the synthesis of “high-energy” phosphate bonds during the course of these reactions and used in other metabolic reactions. The details of the individual reactions in the pathways of glucose catabolism have been largely elucidated, but emphasis here is being placed on the interrelationships of the pathways rather than on the details of the individual reactions. The fundamental conversion required to initiate the oxidation of glucose by a cell is its phosphorylation to form G-6-P. This reaction has been described in Section IV.C.1. The G-6-P formed as a result of the GK (HK-IV) catalyzed reaction is central to glucose catabolism. There are at least five different pathways that G-6-P can follow: free glucose, glycogenesis, glycolysis, hexose monophosphate, and glucuronate pathway. a. Free Glucose The simplest direction for G-6-P is a reversal of phosphorylation by a separate enzyme catalyzed reaction in which G-6-P is hydrolyzed to form free glucose and inorganic phosphate. This reaction is catalyzed by the enzyme G-6-Pase: This is an irreversible reaction that opposes the previously described unidirectional GK (HK-IV) reaction. These two opposing and independently catalyzed enzyme reactions are the site of metabolic control for glucose because the balance of these enzyme activities regulates the net direction of the reaction. Significant amounts of G-6-Pase are found only in liver and to a lesser extent in the kidney. This is in accord with the well-known function of the liver as the principal source of supply of glucose for the maintenance of blood glucose concentration. The G-6-Pase activity is generally higher than the GK activity for most of a 24-hour day except for a few hours after each meal. This means that for most of the day, the liver is supplying glucose rather than using glucose. Muscle G-6-P, however, because of the absence of G-6-Pase, does not contribute glucose from its glycogen to blood directly. Muscle G-6-P does, however, contribute glucose to blood indirectly via the lactate or Cori cycle. Lactate formed in muscle by glycolysis is transported to the liver, where it is resynthesized to glucose and its precursors as outlined in Figure 3-4. b. Glycogenesis This pathway for G-6-Pase leading to the synthesis of glycogen is discussed in Section IV.C.1. c. Anaerobic Glycolysis One of the three oxidative pathways of G-6-P is the classic anaerobic glycolytic or Embden-Meyerhof pathway (EMP). The intermediate steps involved in this pathway of breakdown of G-6-P into two three-carbon compounds are summarized in Figure 3-5. A mole of ATP is used to phosphorylate fructose-6-phosphate (F-6-P) to form fructose-1,6-diphosphate (F-1,6-P). This phosphorylation reaction is also irreversible and catalyzed by a specific kinase, phosphofructokinase (PFK). The opposing unidirectional reaction is catalyzed by a specific phosphatase, fructose-1,6-diphosphatase (F-1,6-Pase). These opposing PFK and F-1,6-Pase catalyzed reactions are a second site of metabolic control regulated by the activities of these two highly specific enzymes. At this point in the process, starting from glucose, a total of two high-energy phosphates from ATP have been donated to form a mole of F-1,6-P. FIGURE 3-4 The lactate or Cori cycle. Muscle cells are devoid of glucose-6-phosphatase, therefore muscle glycogen contributes indirectly to blood glucose by this pathway. F-1,6-P is next cleaved to form two three carbon compounds as shown in Figure 3-5. The next step is an oxidative step catalyzed by the enzyme glyceraldehyde-3-phosphate dehydrogenase (GA-3-PD) with oxidized nicotinamide adenine dinucleotide (NAD+) as the hydrogen acceptor. During the process, the molecule is phosphorylated. In the succeeding steps, the molecule is dephosphorylated at the points indicated, and a mole of ATP is generated at each point. A third site of control of glycolysis is the irreversible formation of pyruvate catalyzed by the enzyme pyruvate kinase (PK). In the reverse direction, two enzymatic reactions operate. Pyruvate carboxylase (PC) first catalyzes the carboxylation of pyruvate to oxaloacetate (OAA), and the OAA is then converted to phospho-enol-pyruvate (PEP) by the enzyme PEP carboxykinase (PEP-CK) (Figs. 3-5 and 3-8). Thus, the overall conversion of a mole of glucose to 2 moles of pyruvate requires 2 moles of ATP for the initial phosphorylations and a total of 4 moles of ATP are generated in the subsequent dephosphorylations. This net gain of 2 moles of ATP represents the useful energy of anaerobic glycolysis. For repeated function of the glycolytic pathway, a supply of NAD+ must be available for use in the oxidative (GA-3-PD) step. Normally in the presence of molecular O2 (i.e., aerobic glycolysis), reduced NADH is reoxidized via the cytochrome system: FIGURE 3-5 The glycolytic or classic Embden-Meyerhof pathway (EMP). Note that 2 moles of ATP are used and 4 moles of ATP are generated. Abbreviations: ATP, adenosine triphosphate; DHAP, dihydroxy acetone phosphate; GA-3-P, glyceraldehyde-3-phosphate; NAD+, nicotinamide adenine dinucleotide; Pi, inorganic phosphate. which provides a continuous source of NAD+. In the absence of O2 (i.e., anaerobic glycolysis), NADH is reoxidized to NAD+ in the reaction catalyzed by lactate dehydrogenase (LDH) where pyruvate is reduced to lactate and the NADH is the H+ donor. Therefore, by this “coupling” of the LDH system to the GA-3-PD system, anaerobic breakdown of glucose to lactate proceeds in the absence of 02. As noted earlier, this anaerobic system generates only 2 moles of ATP and when compared to the 36 moles of ATP generated in aerobic glycolysis, anaerobic glycolysis is not very efficient. This alternate route of G-6-P oxidation has been variously referred to as the pentose phosphate pathway (PPP), direct oxidative pathway, Warburg-Dickens scheme, the hexose monophosphate pathway (HMP), or the hexose monophosphate shunt. The initial step of the shunt pathway involves the oxidation of G-6-P at the C-1 position to form 6-phosphogluconate (6-PG) as summarized in Figure 3-6. The reaction is catalyzed by glucose-6-phosphate dehydrogenase (G-6-PD) and in this pathway, oxidized nicotinamide adenine dinucleotide phosphate (NADP+) serves as the hydrogen acceptor. In the second oxidative step, 6-P-G is oxidatively decarboxylated by 6-phosphogluconate dehydrogenase (6-P-GD) to yield a pentose phosphate, ribulose-5-phosphate (Rib-5-P), again in the presence of NADP+. Thus, in the initial reactions, which are essentially irreversible, 2 moles of NADPH are formed. In this pathway, only the C-1 carbon atom of the glucose molecule is evolved as CO2. By contrast, glucose catabolism via the glycolytic scheme results in the loss of both the C-1 and C-6 carbon atoms as CO2 when pyruvate is oxidatively decarboxylated to form acetyl-CoA. This difference in CO2 evolution is used to study partitioning of glucose metabolism through the glycolytic (EMP) pathway and the HMP shunt pathway in domestic animals. The subsequent metabolism of the Rib-5-P in the HMP shunt is also shown in Figure 3-6. As a result of the series of transformations, F-6-P and GA-3-P are formed, which serve as recycling links into the glycolytic pathway. For continued functioning of the HMP shunt pathway, a supply of NADP+ must be available to act as the hydrogen acceptor. Oxidized NADP+ is regenerated from NADPH via the cytochrome system in the presence of O2 so the HMP pathway is an aerobic pathway of glucose oxidation. Reduced NADPH is also required as a hydrogen donor in the synthesis of fatty acids. Through generation of NADPH, the HMP shunt route of carbohydrate metabolism is linked to that of fat synthesis. Accordingly, glucose oxidation through the HMP shunt pathway is essential for the synthesis of fat. In general, the HMP pathway is the major source of the NADPH, which maintains the reductive environment for all biosynthetic processes using NADPH as a cofactor. FIGURE 3-6 The pentose phosphate pathway (PPP) or the hexose monophosphate pathway (HMP). Abbreviations: NADP+, nicotinamide adenine dinucleotide phosphate; TK, transketolase; TA, transketolase; TA, transaldolase; C*O2, is derived from C1 of glucose. FIGURE 3-7 Glucuronate pathway or the C6 oxidation pathway. Note that vitamin C is synthesized via this pathway. This is an alternate pathway of G-6-P oxidation, which has been named the uronate pathway, the glucuronate pathway, or the C6 oxidative pathway. This pathway is shown in Figure 3-7. The initial steps of this pathway involve the formation of uridine diphosphoglucose (UDPG), which, as noted earlier, is an intermediate in glycogen synthesis. G-6-P is first converted to G-1-P, which then reacts with uridine triphosphate (UTP) to form UDPG. This product is then oxidized at the C6 position of the glucose moiety in contrast to the C1 position, which is oxidized in the HMP shunt pathway. This reaction requires NAD+ as a cofactor and the products of the reaction are uridine diphosphoglucuronic acid (UDPGA) and NADH. This UDPGA is involved in a large number of important conjugation reactions in animals (e.g., bilirubin glucuronide formation, synthesis of mucopolysaccharides [chondroitin sulfate], which contain glucuronic acid, and generally in detoxification reactions). UDPGA is cleaved to release D-glucuronate and UDP. D-glucuronate is next reduced to L-gulonate in a reaction catalyzed by the enzyme gulonate dehydrogenase (GUD), with NADPH as the hydrogen donor. The L-gulonate may be converted to a pentose, L-xylulose, or to vitamin C. When converted to L-xylulose, the C-6 carbon of L-gulonate is oxidatively decarboxylated and evolved as CO2. The L-xylulose is then reduced to xylitol, catalyzed by the enzyme L-xylulose reductase. This is the enzyme that is deficient in pentosuria of humans. As shown in Figure 3-7, xylitol is converted to D-xylulose, which is then phosphorylated to D-xylulose-5-P and a cyclical pathway involving the HMP shunt pathway may occur. L-gulonate is also converted by enzyme-catalyzed reactions to L-ascorbate in those species that can synthesize their own vitamin C (i.e., all domestic animals). The enzyme, L-gulonolactone oxidase (GLO), is lacking in humans, nonhuman primates, and guinea pigs, and therefore vitamin C must be supplied in their diets. The enzyme is present only in the liver of the mouse, rat, pig, cow, and dog. In the dog, the liver GLO activity is low and the ascorbate hydrolytic activity is high so dogs may have additional needs for vitamin C during stress (e.g., wound healing, postsurgical stress). For vitamin C synthesis, D-galactose may be an even better precursor than D-glucose. This pathway is also included in Figure 3-7. The metabolic pathways described thus far are those of the carbohydrates. In analogous fashion, the breakdown of fats and of proteins also follows independent pathways leading to the formation of organic acids. Among the organic acids formed from lipids are acetyl-CoA (AcCoA), acetoacetate (AcAc), and 3-OH-butyrate (3-OH-B) from the β-oxidation of fatty acids. From proteins, pyruvate, oxaloacetate (OAA), and α-ketoglutarate (α-KG) are formed from transamination of their corresponding α-amino acids. Direct deamination of amino acids is also a route of formation of organic acids. These organic acid intermediate metabolites are indistinguishable in their subsequent interconversions. Thus, the breakdown of the three major dietary constituents converges into a final common pathway, which also serves as a pathway for the interconversions between them. a. Pyruvate Metabolism The pathway for breakdown of glucose to pyruvate has been described in Section IV.D.1. Pyruvate, if it is not reduced to lactate, is oxidatively decarboxylated in a complex enzymatic system requiring the presence of lipoic acid, thiamine pyrophosphate (TPP), coenzyme A (CoA), NAD+, and pyruvate dehydrogenase (PD) to form AcCoA and NADH. Pyruvate may follow a number of pathways as outlined in Figure 3-8. The conversion of pyruvate to lactate was described in Section IV.D.1. By the mechanism of transamination or amino group transfer, pyruvate may be reversibly converted to alanine. The general reaction for an amino group transfer is FIGURE 3-8 Pathways of acetate and pyruvate metabolism. where the amino group of an amino acid is transferred to the α position of an α-keto acid and as a result, the amino acid is converted to its corresponding α-keto acid. This reaction requires the presence of vitamin B6 as pyridoxal phosphate and is catalyzed by a specific transferase, in this case alanine aminotransferase (ALT). Serum levels of several of these transferases (e.g., ALT and aspartate aminotransferase [AST]) have been particularly useful in the diagnosis and evaluation of liver and muscle disorders, respectively. These aspects are discussed in the individual chapters on liver and muscle function. The energetics of the reaction from phosphoenolpyruvate (PEP) to form pyruvate and catalyzed by pyruvate kinase (PK) are such that this is an irreversible reaction, as is the PD catalyzed conversion of pyruvate to AcCoA. A two-step separate pathway to reverse this process is present at this step so this is a fourth site of directional metabolic control. Through a CO2 fixation reaction in the presence of NADP+-linked malate dehydrogenase (MD), malate is formed from pyruvate. Malate is then oxidized to OAA in the presence of NAD+-linked MD. OAA may also be formed directly from pyruvate by the reaction catalyzed by pyruvate carboxylase (PC). OAA formed by either route may then be phosphorylated and decarboxylated to form PEP in a reaction catalyzed by PEP carboxykinase (PEP-CK). Thus, a pathway in the reverse direction of the PK reaction is present for gluconeogenesis from lower intermediates. These pathways for pyruvate metabolism are outlined in Figure 3-8, which includes the dicarboxylic acid cycle. FIGURE 3-9 Tricarboxylic acid cycle. The pathway for the entry of propionate into the metabolic scheme is also included. The asterisks give the distribution of carbon in a single turn of the cycle starting with acetyl-CoA. Note the randomization of carbon atoms at the succinate step. b. Tricarboxylic Acid Cycle AcCoA formed by the oxidative decarboxylation of pyruvate also has a number of metabolic routes available. AcCoA occupies a central position in synthetic and in oxidative pathways as shown in Figure 3-8. The oxidative pathway leading to the breakdown of AcCoA to CO2 and H2O follows a cyclical pathway that is the tricarboxylic acid (TCA) cycle, citric acid cycle, or the Kreb’s cycle. The major steps involved are given in Figure 3-9. In a single turn of the cycle, a mole of AcCoA enters, 2 moles of CO2 are evolved, and a mole of OAA is regenerated. The regenerated OAA may then condense with another mole of AcCoA, and the cycle continues. Citric acid is a symmetrical molecule that behaves asymmetrically as shown in Figure 3-9. Also, the CO2 that is evolved is derived from that portion of the molecule contributed by OAA during each turn of the cycle. The expected distribution of carbon atoms from AcCoA in one turn of the cycle is also given in Figure 3-9. During one turn of the cycle, a randomization of carbon atoms occurs at the succinate level such that CO2 derived from the carboxyl group of acetate will be evolved during the next turn of the cycle. In the process, 3 moles of NAD+ and a mole of a flavin nucleotide (FAD) are reduced, and a mole of ATP is generated as noted in Figure 3-9. In animal tissues, there is a cytoplasmic NADP+-linked isocitric dehydrogenase (ICD), which is not associated with the mitochondrial NAD+-linked ICD or other enzymes of the TCA cycle. The NADP+-ICD is another enzyme used as an aid to diagnose liver disease. According to Figure 3-9, the TCA cycle is a repetitive process based on the regeneration of OAA at each turn. In addition, other metabolic pathways are available for intermediates in the cycle. Reversal of the transamination reactions previously described to form aspartate or glutamate would result in a withdrawal of OAA and α-KG, respectively, from the cycle. By decarboxylation, OAA may also be withdrawn to form PEP, and malate may form pyruvate and thence other glycolytic intermediates as shown in Figure 3-8. Continued losses of these intermediates into other metabolic pathways would theoretically result in a decrease in the rate of operation of the cycle. A number of metabolic pathways are known whereby the losses of cycle intermediates may be balanced by replacement from other sources and are shown in Figure 3-8. The amino acids, aspartate and glutamate, may function as sources of supply as well as routes for withdrawal. The CO2 fixation reactions, which are the reversal of the reactions previously described, may also function as important sources of supply. A fourth CO2-fixing reaction is especially important in ruminants because propionate is a major product of rumen fermentation and is a major supplier of intermediates for the TCA cycle. Propionate is one of the three major fatty acids, with acetate and butyrate, involved in ruminant metabolism. The energy of carbohydrate breakdown must be converted to high-energy phosphate compounds to be useful to the organism; otherwise the energy is dissipated as heat. The total available chemical energy in the reaction is about 50 kcal/mole or about 7% of the 690 kcal/mole, which is available from the complete oxidation of glucose to CO2 and water. The useful energy of anaerobic glycolysis is represented by the net gain of 2 moles of ATP and the available energy of each is about 7 kcal. Thus, the efficiency of glycolytic breakdown of glucose to pyruvate is 14 kcal or 28% of the available 50 kcal or only 2% of the total available 690 kcal in glucose. The major portion of the energy of glucose is generated in the further aerobic oxidation of pyruvate to CO2 and H2O. In the oxidative or dehydrogenation steps, NADH or NADPH (FAD in the succinate step) is formed. In the presence of molecular O2, these compounds are reoxidized to NAD+ or NADP+ in the cytochrome system. In the sequence of reactions of this system, 3 moles of ATP are formed per mole of NADH or NADPH oxidized to NAD+ or NADP+. This transfer of energy to ATP is known as oxidative phosphorylation, or ox-phos. The yield of high-energy phosphate bonds in the form of ATP in the system per atom of oxygen consumed (½ O2) is conventionally referred to as the P:O ratio, which in this case is 3. Table 3-3 presents a balance sheet of the ATPs formed in the various steps, and 36 of the total 38 ATPs are generated in aerobic glycolysis. The complete oxidation of a mole of glucose to CO2 and water yields 690 kcal, and therefore the net gain of 38 ATPs in anaerobic plus aerobic glycolysis represents 266 kcal for an overall efficiency of 38%. In comparison, the efficiency of the modern internal combustion engine is about 20%. The pathways by which the breakdown products of lipids and proteins enter the common metabolic pathway have been described in previous sections. The principal points at which carbohydrate carbon may be interconverted between amino acids and fatty acids are outlined in Figure 3-10. Thus, certain amino acids (glycogenic) can serve as precursors of carbohydrate through the transamination reactions, and by reversal of these transaminations, carbohydrates can serve as precursors of amino acids. FIGURE 3-10 Interrelationships of carbohydrate, protein, and lipid metabolism. The relationship between carbohydrate and lipid metabolism deserves special mention for the carbohydrate economy, and the status of glucose oxidation strongly influences lipid metabolism. A brief description of lipid metabolism follows, and greater detail may be found in the chapter on lipid metabolism. Intracellular fatty acids are either synthesized in the cytoplasm or taken up as free fatty acids. Fatty acid oxidation begins in the cytoplasm with the activation of fatty acids to form fatty acyl-CoA. The activated fatty acyl-CoA is bound to carnitine for transport into the mitochondria where fatty acyl-CoA is released for intramitochondrial oxidation. The classical β-oxidation scheme for the breakdown of fatty acids whereby two-carbon units are successively removed is a repetitive process involving four successive reactions. After the initial activation to form a CoA derivative, there is (1) a dehydrogenation, (2) a hydration, (3) a second dehydrogenation, and (4) a cleavage of a two-carbon unit. The result is the formation of AcCoA and a fatty acid residue shorter by two carbon atoms. The residue can then recycle to form successive AcCoA molecules until final breakdown is achieved. In the case of odd-chain fatty acids, propionyl-CoA is formed in the final cleavage reaction. The hydrogen acceptors in the oxidative steps are NAD+ and FAD. The further oxidation of AcCoA to CO2 and water proceeds in the common pathway of the TCA cycle. In the process, 2 moles of CO2 are evolved per mole of AcCoA entering the cycle. Therefore, fatty acids could not theoretically lead to a net synthesis of carbohydrate. Net synthesis of carbohydrate from fatty acids would require the direct conversion of AcCoA into some glucose precursor (i.e., pyruvate). The reaction however, is irreversible and the only route by which fatty acid carbon could theoretically appear in carbohydrate is through the TCA cycle intermediates, and this occurs without a net synthesis. The pathway for fatty acid synthesis is separate from that of the β-oxidation mechanism for fatty acid breakdown. Malonyl-CoA is first formed by the addition of CO2. Subsequently, two carbon units from malonyl-CoA are sequentially added to the growing chain with a loss of CO2 at each addition. At each step, there is also a reduction, dehydration, and a final reduction to form a fatty acid that is two carbons longer than the previous one. The synthesis of fatty acids also requires NADPH as the hydrogen donor rather than NADH or FADH. The major source of NADPH is during the oxidation of glucose in the HMP shunt pathway. NADPH concentration is also high in the cytoplasm of liver and adipose cells where HMP shunt activity is also high. The availability of this NADPH is the basis for the linkage of carbohydrate oxidation to lipid synthesis. AcCoA is also the precursor of cholesterol and the ketone bodies: AcAc, 3-OH-B, and acetone. The synthesis of cholesterol proceeds through a series of reactions beginning with the stepwise condensation of 3 moles of AcCoA to form β-hydroxy-β-methyl glutaryl-CoA (HMG-CoA). As shown in Figure 3-10, HMG-CoA is a common intermediate for the synthesis of cholesterol and ketone bodies in the liver cell. In liver, a deacylating enzyme is present, which cleaves HMG-CoA to yield AcCoA and free AcAc. This is the HMG-CoA cycle. The free AcAc then diffuses out of the cell and enters the general circulation. For further oxidations to occur, AcAc is “reactivated” with CoA in extrahepatic tissues (muscle) by the transfer of CoA from succinyl-CoA to form AcAcCoA. Increased ketogenesis and ketonemia are the net result of alterations in metabolic pathways or enzymes that favor the accumulation of AcAcCoA. Prime examples are diabetes mellitus and bovine ketosis. The increased mobilization and utilization of fatty acids are a well-known requisite for ketogenesis under conditions of starvation and diabetes. Under these same conditions, lipid synthesis from AcCoA is also depressed. The net effect of either or both of these alterations favors the accumulation of AcCoA and thus ketogenesis. Increased ketogenesis is always associated with an increased rate of gluconeogenesis in association with an increased activity of the key gluconeogenic enzyme, PEP-carboxykinase (PEP-CK). The increased rate of gluconeogenesis in turn depletes OAA. There is an increase in the NADH/NAD ratio, which would promote the conversion of OAA to malate, thereby depleting OAA. With the depletion of OAA and subsequent OAA deficiency, there is an insufficient condensing partner for AcCoA for the Kreb’s cycle. The AcCoA is then readily diverted to ketone bodies. Hepatic ketogenesis is regulated by the rate limiting transfer of FFA across the mitochondrial membrane. Carnitine acyl transferase, the enzyme system responsible for the mitochondrial uptake of FFA, is increased in diabetes and contributes to the ketogenesis. In addition to the separation of the biochemical pathways for lipid oxidation and lipid synthesis, an anatomical separation of lipid metabolism is also present. The liver is the major site of fatty acid oxidation and the adipose tissue is the major site of lipid synthesis. Adipose tissue, in vitro, converts glucose carbons to fatty acids faster than does liver tissue. It is well known that, with excessive carbohydrate feeding, fat depots in the body increase. Fasting, on the other hand, depresses the respiratory quotient (R.Q.) indicating that the animal is now using body fat as the energy source. During fasting, plasma FFA also increase, and when carbohydrate is supplied, they decrease. The presence of glucoseboth stimulates lipogenesis and spares fatty acid from oxidation. In diseases with an inability to utilize glucose (e.g., diabetes), depression of lipogenesis is a characteristic finding. When there is adequate glucose oxidation (e.g., successful insulin therapy in diabetes), lipid synthesis is restored and the animal regains its weight. In those conditions with decreased glucose use or availability (e.g., diabetes, starvation, ruminant ketosis), there is an increased release of glucose precursors (amino acids) from muscle and FFA from adipose tissues mediated by activated hormone-sensitive lipases (HSL) (Khoo et al., 1973). The amino acids and FFA are transported to the liver where the amino acids follow gluconeogenic pathways. Fatty acids follow pathways toward oxidation and ketogenesis and, additionally, glucagon promotes hepatic ketogenesis. There is also an underutilization of ketones in the peripheral tissues of dogs (Balasse and Havel, 1971; Foster and McGarry, 1982). The net result is an overproduction of glucose and ketones in liver and an underutilization of both in the peripheral tissues. The internal secretions of the anterior pituitary, adrenal cortex and medulla, and the pancreas are closely associated with carbohydrate metabolism. The pituitary and adrenal factors were discussed in Section IV.C, together with glucagon. More detailed information is available in the chapters on pituitary and adrenal function. Since the successful extraction of insulin by Banting and Best in 1921, a vast amount of literature has accumulated on its role in carbohydrate metabolism and continues to this day. The fine details of insulin action are still being studied, and a basic understanding of the major biochemical events that occur in animals with and without insulin has evolved. The elucidation of the insulin structure by Sanger in 1959 was soon followed by the discovery of its precursor, proinsulin, and its structure was quickly known. It has been the subject of many reviews (Raptis and Dimitriadis, 1985; Steiner, 2004). Proinsulin is a single-chain looped polypeptide linked by disulfide bridges (Fig. 3-11). It varies in length from 78 amino acid residues in the dog to 86 for the human, horse, and rat. Its m.w. is near 9000 daltons. Proinsulin is synthesized in the pancreatic β-cells on the rough endoplasmic reticulum (RER) and transported and stored in the secretory granules on the Golgi apparatus. There, the central connecting polypeptide or C-peptide is cleaved from the chain by proteolytic enzymes, and the two linked end fragments are the monomeric insulin molecule. C-peptide has an m.w. of 3600 daltons and is devoid of biological activity. FIGURE 3-11 Insulin and proinsulin. Proinsulin is the coiled polypeptide. When the connecting C-peptide (open circle) is removed, the insulin molecule (solid circle) is released. In the pancreatic cells, as the insulin moiety is cleaved from the proinsulin, it crystallizes with zinc for storage in the β-cell granules. The dense central inclusions of these insulin secretory granules consist mainly of crystalline insulin. Insulin release is stimulated by glucose, amino acids, hormones (glucagon, gastrin, secretin, pancreozymin), and drugs (sulfonyl ureas, isoproterenol). Insulin release is inhibited by hypoglycemia, somatostatin, and many drugs, such as dilantin and phenothiazines. The liver is the primary site of insulin degradation, and the kidney is a secondary site. The half-life of insulin in the circulation is between 5 and 10 min (Steiner, 1977). The A chain of insulin consists of 21 amino acids and the B chain of 30 amino acid residues (Fig. 3-11). The m.w. of the insulin monomer is 6000 daltons and is the smallest unit possessing biological activity. Under physiological conditions, 4 molecules of insulin are linked together to form a tetramer, the active molecule. Insulin obtained from various species differs in amino acid composition in Chain A or Chain B or both (Table 3-4). Differences occur within species also as rats and mice (Markussen, 1971) have two nonallelic insulins. These structural differences among the various species of animals are not located at critical sites, however, because they do not affect their biological activity. They do, however, affect their immunological behavior. The amount of insulin stored in the pancreata of various species also differs. The dog stores about 3.3 units per gram of pancreas, which amounts to about 75 I.U. in a 10-kg dog. This amount, if suddenly released, would be fatal. Insulin release is affected by glucose, mannose, leucine, other amino acids, ketone bodies, and fatty acids. This release is mediated by glucagon, a hormone, which increases cAMP and potentiates the insulin response. The sulfonylureas are effective as pharmacological agents to release insulin, the basis for their therapeutic use. TABLE 3-4 Species Variation in Amino Acid Sequence of Insulina From Renold and Cahill (1966) and Naithani et al. (1984). aThese are the sites of variation on the A chains and the B chains. Ala = alanine; Asp = aspartic acid; Glu = glutamic acid; Ileu = isoleucine; Met = methionine; Ser = serine; Thr = threonine.
I. INTRODUCTION
II. DIGESTION
III. ABSORPTION
IV. METABOLISM OF ABSORBED CARBOHYDRATES
A. General
B. Storage as Glycogen
C. Glycogen Metabolism
1. Glycogenesis
2. Glycogenolysis
3. Hormonal influences on glycogen metabolism
4. Glycogen in Disease
D. Catabolism of Glucose
1. Pathways of Glucose-6-Phosphate Metabolism
d. Hexose Monophosphate Pathway
e. Glucuronate Pathway
2. Terminal Oxidation: Aerobic Glycolysis
3. Carbon Dioxide Fixation in Animals
4. Energy Relationships in Carbohydrate Metabolism
V. INTERRELATIONSHIPS OF CARBOHYDRATE, LIPID, AND PROTEIN METABOLISM
A. Lipid Metabolism
1. Oxidation of Fatty Acids
2. Synthesis of Fatty Acids
3. Synthesis of Cholesterol and Ketone Bodies
B. Influence of Glucose Oxidation on Lipid Metabolism
VI. INSULIN AND CARBOHYDRATE METABOLISM
A. Proinsulin and Insulin
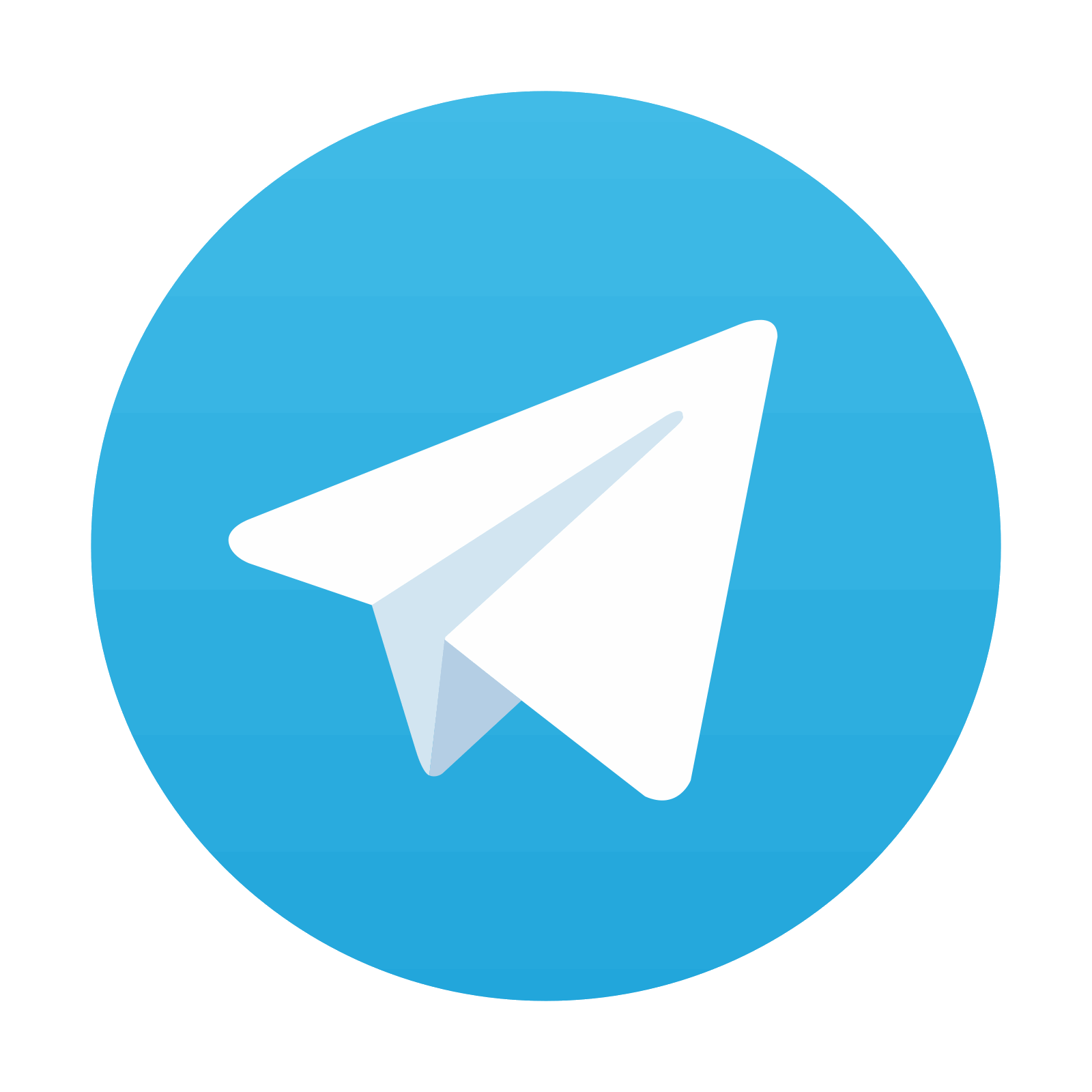
Stay updated, free articles. Join our Telegram channel
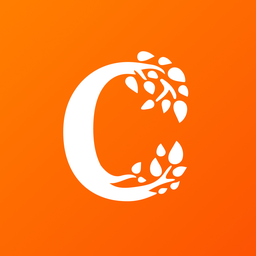
Full access? Get Clinical Tree
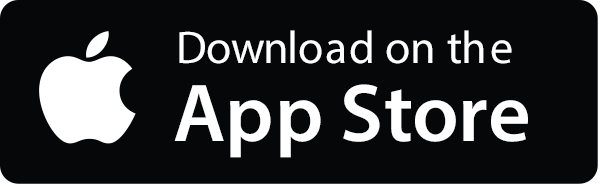
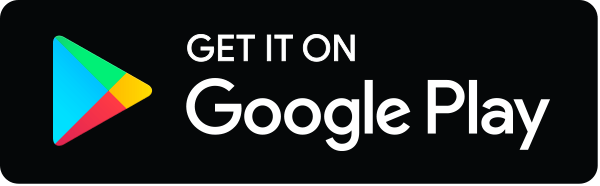