and Maria Cristina Rosi1
(1)
Department of Preclinical and Clinical Pharmacology, University of Florence, Florence, Italy
Abstract
The neurochemical alterations underlying the cognitive and behavioral symptoms that constitute the clinical picture of Alzheimer’s disease (AD), which should be reproduced by an animal model of the disease, are briefly described. The ideal animal model of AD and related dementias should include β-amyloid deposition evolving in senile plaques (SP), tau protein hyperphosphorylation resulting in neurofibrillary tangles (NFT), an inflammatory reaction, and the degeneration of the forebrain cholinergic neurons with the ensuing cholinergic hypofunction. However, since the understanding of AD pathogenesis has grown step by step during 30 years of research, simpler models, only reproducing one or a few neurochemical changes, have been gradually developed beginning from the lesion of the cholinergic nuclei and ending with transgenic mice developing SP and NFT. The partial models still maintain their usefulness for understanding the different pathogenetic mechanisms and for developing new drugs. The animal models are validated by the demonstration, through appropriate and specific methods, that they show the neurochemical changes that they are meant to reproduce. The methods are mentioned and briefly described in this chapter, and evidence of their validity is given by quoting significant papers in which they have been used.
Key words
APP miceβ-amyloidbrain inflammationcholinergic hypofunctionneurofibrillary tanglestau hyperphosphorylation1 Neurochemical Changes in Alzheimer Dementia
To validate an animal model, it is mandatory to define the pathological features of the disease that the model should mimic. Of the many forms of dementia existing, we will examine in this chapter the models of Alzheimer’s disease (AD), and briefly mention the models of frontotemporal dementia (FTD). The neurochemical alterations underlying the cognitive and behavioral symptoms that constitute the clinical picture of AD, and that we expect to find in a model, will be briefly described, and are summarized in Table 1.
Table 1
The main neuropathological alterations in AD that should be mimicked by an animal model
Impairment of neurotransmitter systems: |
Degeneration of forebrain cholinergic neurons (↓ ChAT; AChE, ACh) |
Loss of cortical pyramidal neurons (↓ Glu) |
β-Amyloid deposition (plaques, vessels, intracellular) |
Tau protein hyperphosphorylation (NFT) |
Inflammatory reaction |
1.1 Impairment of Neurotransmitter Systems
The first neurochemical change detected postmortem, more than 30 years ago, in the brain of patients affected by AD was a severe decrease in choline acetyltransferase (ChAT) activity in the cerebral cortex and hippocampus (1) This observation was followed by extensive investigations leading to the “cholinergic hypothesis” (2) on which the present therapy of AD was built. It was demonstrated that the decrease in ChAT activity resulted from the loss of cholinergic neurons in the forebrain cholinergic nuclei (3), which was associated with a reduction in acetylcholine (ACh) formation, observed in cortical biopsies from AD patients (4). The ChAT activity decrease correlated with the severity of cognitive impairment (5). Several reviews summarize the changes of the cholinergic systems in AD and related dementias (6–8). The changes also include a decrease in acetylcholinesterase (AChE) activity in the cerebral cortex and hippocampus, an increase in butyrylcholinesterase (BuChE) activity, a decrease in vesicular ACh transporter (VAChT) activity, a loss of α4β2 and α7 nicotinic receptors, and of M2 and M4 muscarinic receptors.
In AD, other neurotransmitter systems are also affected, as reviewed by Hardy et al. (6) and Gottfries (7), although none of them is damaged as extensively and in all patients as the cholinergic system. Loss of noradrenaline, dopamine, serotonin, and changes in their receptor-signal transduction pathways as well as their metabolites have been observed in the brains of AD patients (6, 9). Antemortem investigation of amino acid content in the neocortex of demented patients with AD showed that glutamate is the only significantly reduced amino acid (10). Finally, among the many neuropeptides investigated, a decrease in somatostatin levels was repeatedly found postmortem in the hippocampus and cortical regions of AD subjects (11).
1.2 β-Amyloid and Tau Protein
No matter how important the role of the neurotransmitter system impairment is in AD pathogenesis, it is considered secondary to the deposition of β-amyloid (Aβ) protein and the phosphorylation of tau protein, which are regarded as the biochemical events responsible for the built-up of the senile plaques (SP) and neurofibrillary tangles (NFT), respectively. These are the two histopathological features characterizing AD as described a century ago by Alois Alzheimer. The formation of Aβ from the β-amyloid protein precursor (APP), its amino acid composition, conformation, and role in physiology and pathology have been the subject of many reviews (12–14). From these studies, the “amyloid cascade hypothesis” of AD pathogenesis was formulated (15).
Two years after the discovery of the main component of SP, Grundke-Iqbal et al. (16) demonstrated that the main component of the paired helical filaments that make up the characteristic NFT is an abnormally hyperphosphorylated form of the microtubule-associated protein tau. The extensive studies leading to the understanding of tau phosphorylation and polymerization, and the identification of taupathies, including FTD, are reviewed in (17–19).
1.3 Brain Inflammation
The immunohistochemical demonstration of reactive microglia in AD brains (22) and the epidemiological observations that subjects treated with anti-inflammatory agents had a greatly reduced risk of AD (23) attracted attention towards the large inflammatory reaction associated with SP, triggered by Aβ (24). The inflammatory reaction produces cellular and biochemical changes including microglial and astroglial activation with an increase in phospho-p38 MAPK, inducible cyclooxygenase (Cox 2) and NO synthase expression resulting in the production of prostaglandins, interleukins, NO, free radicals, as summarized by several reviews (25–27). The inflammatory products play an important pathogenetic role in the neuronal neurodegeneration characterizing AD (28, 29) and are considered a potential therapeutic target.
2 Animal Models
The ideal animal model of AD and related dementias should include all the neurochemical changes described in the previous section. However, since the understanding of AD pathogenesis has grown step by step during 30 years of research, simple models, only mimicking one or a few pathogenetic mechanisms, have been gradually developed. These partial models maintain their usefulness for investigating the different pathogenetic mechanisms, and in the drug discovery pipeline for dementia.
2.1 Impairment of Neurotransmitter Systems
None of the monoaminergic, amino acidergic, and peptidergic neurotransmitter systems is so constantly and deeply affected in AD as the cholinergic system. Therefore, several models reproducing the loss of cholinergic neurons and the ensuing functional cholinergic hypofunction have been developed. The models used are: animals in which the cholinergic neurons were lesioned by excitotoxins (30), immunotoxins, such as 192 IgG-saporin (31), Aβ peptides (32), as well as AD mice in which the expression of anti-nerve growth factor (NGF) antibodies was induced (33). Other models in which a cholinergic hypofunction has been detected and investigated are, among many others, aging animals (34); rats with a diffuse brain inflammation induced by bacterial lipopolysaccharide administration (35); and transgenic mice overexpressing Aβ (36). The aims of the models were to investigate the role of cholinergic hypofunction in AD pathogenesis, particularly in the cognitive deficit, mostly to test drugs restoring the cholinergic function and treating AD memory impairment.
A detailed description of the models is given in other chapters of this book; here their neurochemical validation is presented.
2.1.1 Methods to Validate the Cholinergic Deficit
Several markers can be investigated to demonstrate and validate a cholinergic deficit:
1.
Immunohistochemical visualization and quantification of the ChAT-positive forebrain neurons by the use of polyclonal rabbit antibodies (1:200) (Chemicon, Temecula Ca). Thirty-μm-thick coronal slices are prepared from brain removed from deeply anesthetized rats or mice perfused with a 4% paraformaldehyde solution in phosphate buffer. Free floating sections are incubated overnight at 4°C with the primary antibodies. For details of the method, see (37) and (36). Cell counting can be performed manually, using a 20x objective with a calibrated eyepiece grid. The mean area of the ChAT–positive cells can be evaluated by using, e.g., Analysis B Software (Olympus, Hamburg Germany). A local injection of the specific 192 IgG-saporin toxin in rat nucleus basalis may result in the disappearance of 92% of the cholinergic neurons (38). In aging rats (24 months and older), the loss of ChAT-positive neurons shows marked individual and strain differences (for a discussion, see (34) and (39)).
2.
Histochemical visualization of AChE-positive neurons and nervous fibers. This is a valid alternative to the visualization of ChAT-positive neurons, which allows not only to count the AChE-positive neurons but also to examine the network of AChE-positive fibers in the neocortex and hippocampus. Modifications of the classical method of Koelle (40), based on the use of acetylthiocholine as a substrate and its visualization with copper sulfate (see (41) for details), are used either on sections from brain fixed with 4% paraformaldehyde solution in phosphate buffer (42) or prepared with a freezing microtome (43). Alternatively, the direct-coloring Karnovsky–Roots method can be used (44). AChE-positive fiber density was estimated by McGaughy and Sarter (43) by means of a Vanox Olympus Research microscope with a 25x magnification. After intracortical infusions of 192 IgG-saporin toxin, the fiber loss ranged between 40% and 60% of total cortical cholinergic fibers.
3.
Determination of ChAT activity. The usefulness of ChAT determination for assessing the cholinergic dysfunction in animal models of neurodegenerative diseases was recently reviewed by Contestabile (45). ChAT activity is measured by the conversion of 1-[14C]acetyl-coenzymeA to [14C]acetylcholine according to the method of Fonnum (46). [3H]acetyl-coenzymeA has also been used (47). In the standard procedure, brain tissue is homogenized in 20 volumes of 10 mM EDTA buffer (pH 7.4) and 2% (v/v) Triton X-100. Incubation time is 15 min. Ballmaier and coworkers (38) found a ChAT activity of 2.42 ± 0.26 and 2.65 ± 0.29 μmol/h/100 mg protein in the frontal and parietal cortex, respectively, with a decrease ranging between 44% and 60% after 192 IgG-saporin toxin injection in rat nucleus basalis. Comparable levels of ChAT activity and similar post-lesion decreases were observed in the cortex by Robinson and colleagues (48). According to these authors, ChAT activity in the hippocampus was 8.57 ± 0.39 μmol/h/100 mg protein with a 52% decrease after 192 IgG-saporin toxin injection in the medial septum.
In aging rats, investigations on cortical ChAT activity did not reveal consistent changes in the cortex (39), as it should be expected from the absence of a clear cut effect of age on the number of cholinergic neurons in the basal forebrain.
4.
Determination of AChE and BuChE activity in discrete brain areas. The determination of AChE activity is an easy and inexpensive method for detecting and evaluating cholinergic deficits (49). Brain samples of about 50–70 mg are homogenized in 50 mM Na-phosphate buffer and incubated with acetylthiocholine as a substrate according to the method of Ellman (50). AChE activity is expressed as mmol acetylthiocholine iodide hydrolyzed per min/mg protein, measured spectrophotometrically at 412 nm. The radiometric method (51, 52) is an alternative based on the enzymatic hydrolysis of [3H]acetylcholine. The [3H] acetic acid formed is extracted and the radioactivity counted. The correspondence between the two methods was checked by Cerbai et al. (52). For the measurement of BuChE activity, the method of Ellman et al. (50), with modifications suggested by Lockridge (52), has been used. Butyrylthiocholine is added as the substrate, instead of acetylthiocholine, to the homogenate, in the presence of a selective inhibitor of AChE, such as BW 248-C51 (52).
Examples of the sensitivity of AChE and ChAT determinations can be found in the papers of Harkany et al. (53) and Van Dam et al. (54). In the first paper, a 26% decrease in cortical ChAT and 28% in AChE activity was detected 14 days after injection of Aβ1–42 peptide in the rat nucleus basalis. Van Dam et al. (54) found a 30% decrease in ChAT and a 40% decrease in AChE activities in the basal forebrain nuclei of 7–8-month-old APP/SWE (APP23) mice. Gil-Bea et al. (49) found a significant correlation between the decrease in ChAT and AChE activities in the frontal cortex after 192 IgG-saporin injection in the nucleus basalis of rats. Taken together, these findings demonstrate that ChAT and AChE methods are equally useful for evaluating the cholinergic deficit.
5.
Determination of high-affinity choline uptake (HACU). HACU is considered a biochemical marker of the localization and integrity of the cholinergic nerve endings, like ChAT activity (55). However, HACU can also be taken as a measure of the activity of the cholinergic neurons (56). For these reasons, HACU has been used for validating the functional cholinergic impairment induced by lesions of the forebrain nuclei (57). In the first days after the lesion, ChAT and HACU decreases are comparable (47), but after a few weeks the decrease in HACU becomes smaller than that of ChAT due to the compensatory increase in activity of the remaining cholinergic neurons. No decrease in cortical HACU activity has been detected in aging rats (39). In aging transgenic Tg2576 mice expressing the Swedish mutation of human APP, no changes have been detected in ChAT and AChE activities; only an HACU decrease (58) was found, a finding indicating that in these mice there was a functional cholinergic impairment without a morphological damage.
HACU is measured by incubating the tissue homogenate with [3H]choline for 5 min at 37°C followed by centrifugation and counting of the amount of [3H]choline accumulated in the pellet, according to Rossner et al. (47) or Atweh et al. (56). An alternative to HACU is the determination of [3H]hemicholinium-3 (HC-3) binding to choline transport sites, as described by Wenk et al. (59), who observed a 70–80% decrease in [3H]C-3 cortical binding a month after ibotenic or quisqualic lesions of the forebrain neurons.
6.
Measurement of ACh extracellular levels in discrete brain areas of freely moving animals. ACh extracellular levels are the expression of the activity-dependent ACh release from the cholinergic nerve endings. For this reason, the changes in extracellular ACh levels, measured by microdialysis, are considered the most dynamic and sensitive indication of functional integrity of the cholinergic neurons. Therefore, the microdialysis technique coupled with a high-sensitivity HPLC detection and quantification method has been often used for assessing the cholinergic impairment and its recovery in many AD models including aging rats, rats with lesions of the forebrain cholinergic nuclei, and transgenic mice overexpressing Aβ. The procedure for measuring extracellular ACh levels in discrete brain regions in rats or mice is more complex, time–consuming, and expensive than the procedure for measuring ChAT and AChE activity and requires some training. It has been described in detail in the Handbook of Microdialysis edited by Westerink and Cremers (60). In the same handbook, the methodological issues specifically concerning ACh release are discussed by Pepeu and Giovannini (61). The microdialysis probes (commonly CMA, Carnegie Medicine or Hospal) are stereotaxically implanted, either vertically or transversally, under anesthesia. The probes are perfused with artificial cerebrospinal fluid beginning 24 h after implantation. Calcium concentration in the fluid is critical and is usually 1.2 mM. AChE inhibitors – usually neostigmine 0.1–0.5 μM – are added in order to protect ACh from hydrolysis and obtain ACh levels more easily detectable with the HPLC methods commonly available. However, if the sensitivity of the methods is increased, AChE inhibitors can be avoided (52).
In aging rats, the cholinergic hypofunction may result in a 35–40% decrease in ACh release from the cerebral cortex and hippocampus (62, 63). This age-associated hypofunction can be corrected by the administration of AChE inhibitors, such as donepezil, rivastigmine, and metrifonate (64). However, according to Sarter and Bruno (39), aging rats do not always show a decrease in basal ACh release, in comparison to young rats; they do so only when the cholinergic forebrain neurons are partially lesioned and activated by drugs or attention-requiring behaviors. Rat strains, perfusion fluid composition, and different placement of the dialysis probe may explain the variability in detecting the age-dependent decrease in ACh extracellular levels.
A decrease in ACh release from the cerebral cortex and hippocampus has been detected following Aβ1–40 or Aβ1–25 peptides injection in the cholinergic forebrain nuclei (53, 65). These experiments validate Aβ peptide intracerebral injections as a useful experimental tool for investigating the mechanisms of their toxicity in vivo.
In rats in which the forebrain cholinergic nuclei have been destroyed by 192 IgG-saporin toxin, Gil-Bea et al. (66) found a 35% decrease in cortical ACh release and a correlation between decrease in ACh release and the decrease in AChE but not in ChAT activity (49).
A cholinergic hypofunction in the brain of transgenic mice, overexpressing APP, has been demonstrated as well. In 7-month-old TgCRND8 mice, a 36% decrease in the basal ACh efflux and a suppression of the increase in ACh release induced by potassium depolarization and scopolamine was shown by Bellucci et al. (36) in comparison with WT controls. Similar results were obtained by Bales et al. (67) in PDAPP mice; Watanabe et al. (68) found no decrease in basal ACh release in Tg2576 mice tested at any age but observed a marked decrease in potassium-evoked release in 9–11 month-old mice.
In conclusion, the examples described in this section demonstrate that of the many methods available to validate the cholinergic hypofunction in AD models, ChAT and AChE determinations are the simplest and most convenient. However, a decrease in these enzymatic activities is detected only when a consistent loss of cholinergic neurons has occurred, whereas the measure of HACU and ACh extracellular levels detect the cholinergic hypofunction in an earlier stage and make it possible to observe its recovery.
As mentioned above (8), in AD patients changes in muscarinic and nicotinic receptors associated with the cholinergic hypofunction take place. Changes in muscarinic receptors have also been detected in rats with a destruction of the cholinergic neurons (47), but there is not enough information to use this feature for validation of a cholinergic hypofunction.
2.2 AD Animal Models with β-amyloid (Aβ) Plaques
Since the definition of the nature of the SP and the formulation of the “amyloid cascade hypothesis” of AD pathogenesis (15), animal models were sought in which spontaneous Aβ plaque formation occurred or SP formation could be induced. Aging monkeys (69) and dogs (70) are animal species in which SP can be found. Transgenic mice have been generated in which APP overexpression leads to Aβ deposits and SP formation (71). Aβ peptides have been injected intracerebrally to form artificial plaques and investigate their toxicity (37).
The validation of these animal models requires the demonstration of the presence of plaques and their quantification. This can be done by using several histological, immunohistochemical, and biochemical methods.
2.2.1 Congo Red Staining
Congo Red is a commonly used histological dye for amyloid detection (72) since the work of Divry (73). It has been used for detecting Aβ deposits in aging animals (74) and in transgenic mice (75) and for visualizing intracerebrally injected Aβ peptide (37). Amyloid in Congo Red-stained fixed paraffin sections reveals the typical pale pinkish to pink-reddish coloration under normal light microscopy, whereas the dye-stained amyloid gives an apple-green birefringence when viewed under polarized light. At closer inspection under electron microscopy, it reveals the fibril nature of its protein constituents. The original method of Congo Red staining has undergone several modifications to improve its sensitivity, specificity, and reliability. The most common modification is the alkaline Congo Red method described by Puchtler (76). Specificity is improved by using freshly prepared stain and a staining solution fully saturated with sodium chloride. For more details on the procedure, see (77–80).
2.2.2 Fluorescent Staining: Thioflavin S
The thioflavin-S histochemical method is used more frequently than the Congo Red method for Aβ deposits visualization in AD animal models. See Selkoe et al. (81) for aging monkeys, Games et al. (76), Bellucci et al. (36) for transgenic mice. The method allows visualization of insoluble proteinaceous material in a pleated β-sheet conformation. For this reason, it is not specific for amyloid deposits only, but detects insoluble aggregates of hyperphosphorylated protein tau as well (82). Five-μm-thick, paraffin-embedded slices are used. The description of the method can be found in Yamamoto and Hirano (83) and Bellucci et al. (84). The staining (green) is observed using a fluorescence microscope. After photography using imaging software, within 24 h of staining, the total number of plaques in the cerebral cortex and hippocampus can be counted and averaged to quantify the plaque load (85).
2.2.3 Immunohistochemistry
The most specific method for visualizing and quantifying Aβ deposits is their detection by means of polyclonal antibodies raised against the human amyloid peptide followed by quantitative analysis of size, number, and total area occupied by plaques in distinct brain regions by means of imaging analysis software. This method is frequently used for assessing the Aβ load in AD animal models and to investigate environmental conditions and pharmacological treatments that may affect it. A few examples are the studies on the effects on Aβ load of immunization with Aβ in mice (86) and dogs (87), environmental enrichment (88), drugs (85), and nutrients (89). In these papers, the standard method is described. Briefly, free floating cortical and hippocampal sections are incubated overnight with the primary antibody, a polyclonal antibody raised against Aβ1–42. On the second day, the sections are incubated with an anti-mouse secondary antibody and the immunostaining is visualized using the avidin–biotin system and a diaminobenzidine substrate kit. To quantify Aβ plaque burden, the stained sections are digitized under constant light and filter setting. Plaque number, size (maximum area), and total area are determined automatically. Data from four to five sections are then summed to derive representative values of the total plaque area for each animal.
2.2.4 Aβ ELISA
Although the histochemical and immunohistochemical visualization of Aβ deposits is sufficient for validating an animal model of AD, a more precise and reliable method to evaluate the Aβ load, including the intraneuronal Aβ deposits, and the nature of the peptides consists in the application of highly specific Enzyme Linked-Immuno-Sorbent Assay (ELISA) systems for quantification of Aβ1–40 and Aβ1–42. According to standard protocols, brains are rapidly harvested and snap-frozen until biochemical assessment of Aβ concentrations. At the time of use, whole brains or specific brain regions are homogenized or sonicated, and cerebral Aβ is solubilized and extracted. After centrifugation, the supernatant is carefully decanted, eventually diluted if the Aβ content is too high, and finally pipetted into the wells of the ELISA plate. Detailed information on the experimental procedure can be found in (85, 87, 90, 91). Each brain sample is usually analyzed in duplicate or triplicate, with the average value reported for each brain sample. The assay provides sensitive and reproducible detection of Aβ peptides through the development of a colorimetric reaction, whose intensity is directly proportional to the concentration of human Aβ1–40 or Aβ1–42 present in the original specimen.
2.3 Animal Models with Neurofibrillary Tangles
Together with Aβ deposition, the presence of NFT, resulting from intraneuronal aggregation of hyperphosphorylated tau protein, represents the other neuropathological hallmark that characterizes the AD brain. Therefore, an ideal animal model, fully mimicking the neurochemical picture of AD, should develop also NFTs and its validation requires a demonstration of their presence. Transgenic mouse models overexpressing mutated human APP with or without additional expression of mutated PS1/2 (please visit http://www.alzforum.org/res/com/tra/default.asp for a complete overview on the genetically engineered AD mouse models generated so far) closely recapitulate many features of the human pathology, including diffuse and neuritic amyloid deposits, dystrophic neurites and synapses, amyloid-associated neuroinflammation, impaired neurotransmitter system functionality, and cognitive deficits (92, 93). On the other hand, a major limitation consisting in the lack of NFT formation reduces their reliability (94, 95). Although abnormal patterns of tau hyperphosphorylation can be observed (84, 96), no typical flame-shaped NFT are generally detected in mice carrying mutated human APP or mutated PS1/2. On the contrary, tangle-like structures occur in the brains of the anti-NGF mice (33) indicating that, as with rodent amyloid, rodent tau is able to form pathological structures itself. The development of several (mutated) tau models (97), the crossing of tau and amyloidosis models (98, 99), and the recent generation of triple models, which featured enhanced amyloid deposition, tau phosphorylation, NFT-like formation, and overt neuronal loss in AD-relevant brain regions (100, 101), seem to overcome this hurdle.
< div class='tao-gold-member'>
Only gold members can continue reading. Log In or Register a > to continue
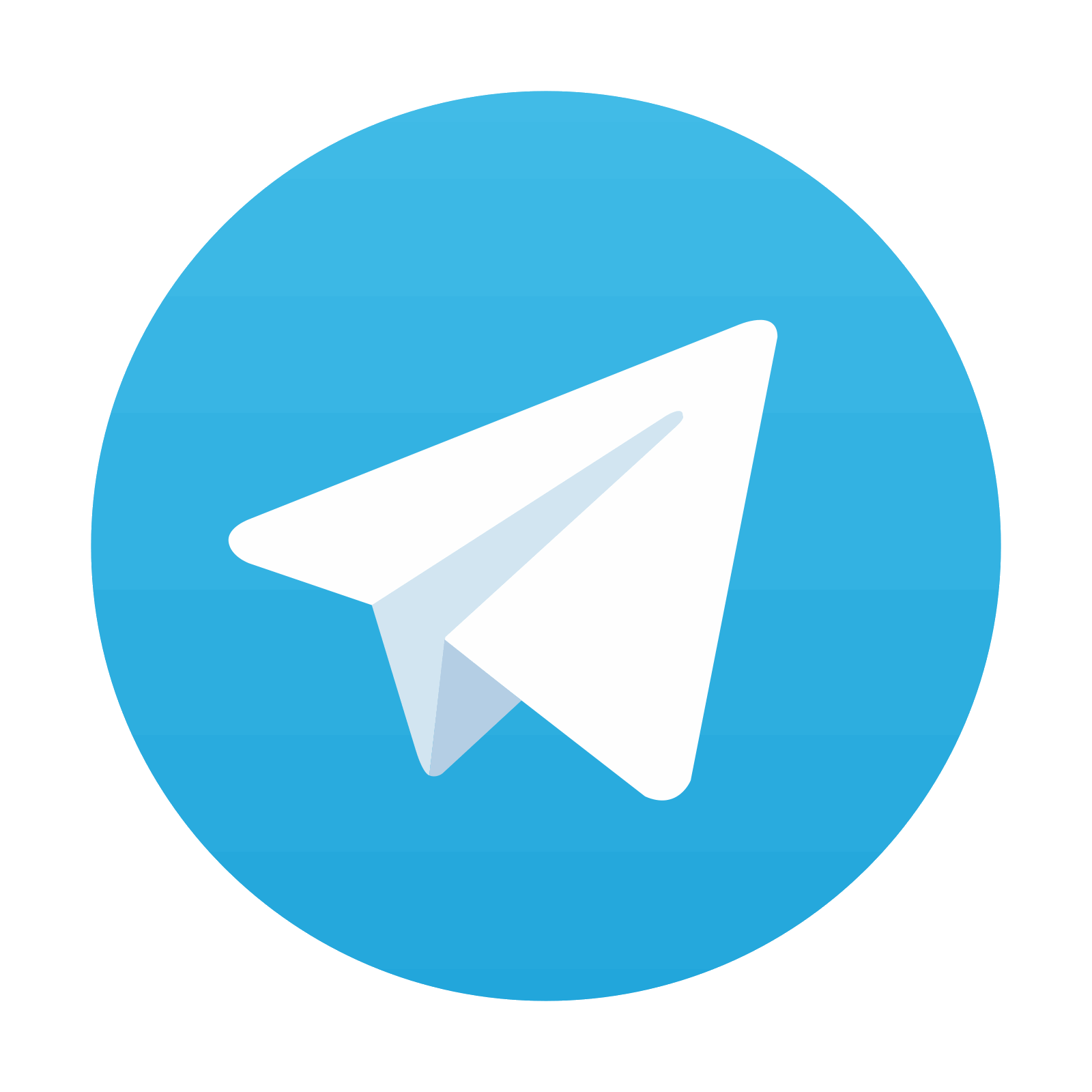
Stay updated, free articles. Join our Telegram channel
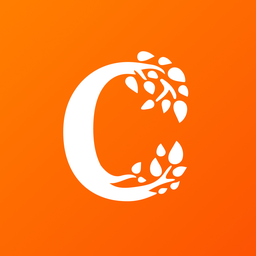
Full access? Get Clinical Tree
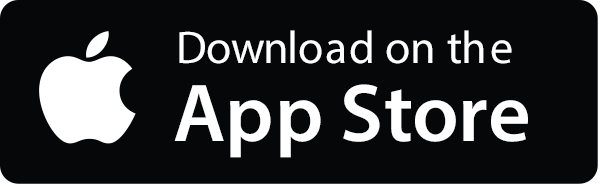
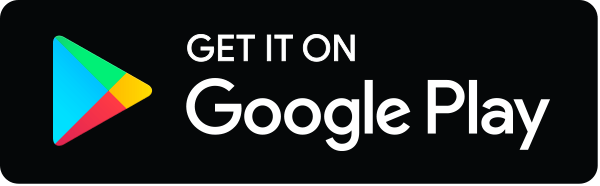