Chapter 22 Trace Minerals A. General Properties of Minerals B. Typical Configurations of Metal Complexes C. Disorders of Cobalt Metabolism D. Copper Metabolism, Absorption, and Transport D. Manganese Metabolism, Absorption, and Transport F. Evaluation of Manganese Status C. Molybdenum Metabolism, Absorption, and Transport C. Selenium Metabolism, Absorption, and Transport D. Disorders of Selenium Metabolism E. Evaluation of Selenium Status D. Zinc Metabolism, Absorption, and Transport Of the 103 elements in the periodic table, about 30 are presently considered essential or important for the normal health and growth of animals. Of these, 16 are often designated as essential trace elements, a classification initially based on the difficulty of measuring such elements with precision in biological tissues. Although the development of new instrumentation has greatly facilitated measurement, the term trace elements has been retained and is still commonly applied to those elements that occur in the body at concentrations in the submicromolar to micromolar range (Fraga, 2005; O’Dell and Sunde, 1997; Reilly, 2004; Ullrey, 2002). This chapter focuses on six elements—cobalt (Co), copper (Cu), manganese (Mn), molybdenum (Mo), selenium (Se), and zinc (Zn)—to illustrate the concepts important to trace element metabolism and disease (Reilly, 2004). These elements have been chosen because there is evidence that perturbations in their metabolism are relatively common. Other biologically active trace elements, although potentially important, often require special conditions or long periods of deprivation before signs of deficiency are recognized, and exposures at 10 to 100 times normal intakes are required before toxic signs are observed (Subcommittee on Dairy Cattle, National Research Council [NRC], 2001; Subcommittee on Mineral Toxicity in Animals, NRC, 1980). In conventional settings, deficiencies of elements such as vanadium, chromium, silicon, nickel, and tin are rarely encountered. Further, arguments for their nutritional essentiality remain controversial. If there is a nutritional need, it is likely to be in the microgram per kilogram of diet range, whereas the relative need for Co, Cu, Zn, Mn, Mo, and Se approaches, or exceeds, amounts in the milligram per kilogram of diet range (Tables 22-1 and 22-2). Most elements accumulate in tissues to some extent. The essential elements, however, are distinguished in that they are intimately associated with the functions of specific organic molecules, mostly proteins with enzymatic properties. When metals function to facilitate enzymatic catalysis, they typically fall into two categories, metalloenzymes and metal-enzyme complexes. Stability constants that define metal binding dictate whether metalloenzyme or metal-enzyme complex is the best designation (Reedijk and Bouwman, 1999; Taylor, 2002). For metalloenzymes there is often good stoichiometry between the moles of metal bound per mole of protein or protein subunit following purification. Metalloenzymes have metal-binding constants of 108 to 109 or greater. Metal protein complexes have constants of 105 or less. Metals in such complexes are easily dissociated upon dialysis of the complex (Reedijk and Bouwman, 1999). TABLE 22-1 Trace Elements Essential for the Development and Health of Mammals and Birds a Deficiency induced experimentally using purified diets in a rigidly controlled environment; “deficiency” is often dependent on and a function of the controlled environment. Trace elements that are nutritionally essential are localized to the fourth and fifth rows of the periodic table. All have incompletely filled d orbitals, except for Cu and Zn. How a given metal facilitates catalytic functions is related in part to its ability to engage in redox (the loss or gain of an electron[s]) or modulate an energy excitable transition state during a catalytic event. Such modulations in protein transition states are sometimes referred as entasis. The entasis (structural dictating) domains of most proteins utilize O, S, or N as electron donors (Riordan and Valle, 1974). When associated with given complexes, if one or two outer shell valence electrons are involved, the result is one oxidation state (e.g., Na + 1, Ca+2, or Mg+2); two or more valence electrons can result in two oxidation states (Fe+2 or Fe+3). The use of electrons in orbitals other than the outermost valence orbitals (e.g., transition metals), however, can have a variety of oxidation states. Some metals can also function as a Lewis acid (i.e., can accept electrons from a base). Enzymes that utilize this property act as acid catalysis (e.g., Zn and the hydrolysis of phosphate esters by alkaline phosphatase (Taylor, 2002). TABLE 22-2 Cobalt, Copper, Manganese, Molybdenum, Selenium, and Zinc Requirements for Young and Adult Dogs, Swine, Sheep, and Beef and Dairy Cattle Expressed as Mg per Kg of Ration for Adequate Intakesa a Values were taken from several industrial and NRC sources (Committee on Animal Nutrition, NRC, 1985; Subcommittee on Dairy Cattle Nutrition, NRC, 2001; Subcommittee on Laboratory Animal Nutrition, NRC, 1995; Subcommittee on Mineral Toxicity in Animals, NRC, 1980; Subcommittee on Swine Nutrition, NRC, 1998; The Salt Institute, www.saltinstitute.org/index.html). b For ruminant animals, the Cu:Mo ratio should exceed 5 or more given the interactions and negative effects Mo has on Cu availability (see text). In simple metal complexes, the basicity of the electron donating group and the ability to approach the metal ion (steric effects) are the primary factors that influence stability. However, when the electron donor groups are bound together into a single molecule capable of binding a given metal, the importance of steric effects is greatly increased and stability depends on a number of other factors including the size and number of rings formed. Five- or six-membered rings have more stability; usually five-membered rings are more stable than six-membered rings. Four-membered rings are rare. Rings larger than six members are less unstable. The stability of calcium complexes of ethylenediamine tetraacetic acid (EDTA) and its homologues with increasing number of C atoms in the bridge between N atoms is as follows: Such complexes are called chelates. Note that the stability also increases as the number of rings increases: Although a further discussion of ligand basicity and steric effects is beyond the scope of this chapter, knowledge of the following relationship can be useful where various coordinating groups are compared in order of decreasing affinity for given metal ions: These relationships are important to the types and shapes of complexes that metal ions form, their stabilities, and their redox potentials. FIGURE 22-1 Distribution of elements in seawater, bacteria, fungi, plants, and animals relative to the earth’s crust plotted as a function of the ionic potential (see text). A reasonable question to ask is why are certain elements more nutritionally important than others? From an evolutionary perspective, relative abundance and, in many cases, the ability to form complexes with catalytic (redox) potential are among the most important reasons. Figure 22-1 shows the distribution of elements in seawater, bacteria, fungi, plants, and animals (Banin and Navrot, 1975). That the patterns are similar suggests common chemical principles have persisted as a part of natural selection. To the extent that the ionic potential of an element is related to its relative abundance in seawater, one can argue that evolutionary choice as it relates to metal utilization is based in part on chemical properties that dictate its interaction with water. For example, when the ionic potential is high (>10), the positive ion appropriates one or more oxygen ions, freeing the hydrogen and forming an oxyanion. Oxyanions are generally soluble; thus, relative to the earth’s crust, the log enrichment is high. This is a characteristic of the nonmetals in the upper right corner of the periodic table (e.g., C, N, O, S, P). Such elements are also the smallest in size to form stable multiple bonds. With time carbon can become a carbonate ion in water and eventually CO2 (O = C = O, a gas) when oxidized further. Contrast that with silicon, carbon’s tetravalent homologue in period 4 of the periodic table. Silicon in water forms silicates, which easily polymerize and are oxidized to the end product silicon dioxide or quartz, a solid, because of the inability to form stable multiple bonds: For elements with low to intermediate ionic potential values, such as silicon (IP < log 0.5), the log of the enrichment factor is often in the range of –1 to + 1, indicating small enrichment or even depletion relative to the crust. Metals with low, but positive, intermediate, or slightly negative ionic potentials tend to form hydroxides in water, most with low solubility. Many of the essential trace elements fall in this category. Finally, elements with negative ionic potentials tend to form hydrated shells and interact by organizing water structure, a role that is important to understanding the functions of Na, K, Mg, and Ca in cells. As an additional perspective, it can be generalized that dietary requirements across species (Table 22-2 and Figure 22-2) are more similar than dissimilar (Rucker, 2007). This is particularly the case when given requirements are expressed per unit of energy consumed or per unit weight of ration. Figure 22-2 shows the relationship for selected mineral requirements and metabolic body size. The requirements of trace elements scale allometrically in a manner that is similar in principle to scaling algorithms (e.g., kWt3/4) for basal metabolism. If a set of common principles was involved in the selection of the elements important to life, it follows that nutrition requirements would be influenced by the same principles (e.g., all cells utilize in principle the same metabolic strategies). Indeed, a strong case can be made that when expressed per unit of food-derived energy or relative to metabolic body size, requirements for essential elements are similar for a diverse array of species. As substances important to catalyst and entasis, it follows consequently that their relative nutritional needs are also driven by factors and principles important to energy utilization. FIGURE 22-2 Relationship of mineral requirements and metabolic body size. Log plots of the daily intake of selected minerals for mice, rats, chickens, dogs, humans, and pigs versus their respective body weights in kilograms. The data for individual minerals plotted in this fashion result in reasonably linear plots with slopes that range from 0.6 to 0.8. For any given mineral, plots of daily intake versus units of body weight are not linear and require polynomial equations to describe the function (insert). Why do deficiencies or excess occur? Nutritional deficiencies obviously result when the intake of essential nutrients consistently falls below the minimal requirement (i.e., a primary deficiency). In animal nutrition this is regrettably common given the tendency to feed monotonous diets or foods common to a given region. Secondary mineral deficiencies can also arise through a variety of mechanisms that include poor bioavailability, interactions with other competing substances, and genetic influences (e.g., polymorphisms that dictate an increased need for given nutrients; Keen, 1996). Table 22-3 provides a list of several mechanisms underlying the development of deficiencies and common interactions that will be amplified in each of the sections that follow. A large animal (50 to 100 kg) can contain 1 to 2 mg of Co with liver containing about 0.1 mg (1.7 μmol), skeletal muscle 0.2 mg (3.4 μmol), bone and hair 0.3 mg (5.1 μmol) each, and adipose tissue 0.4 mg (6.8 μmol) (Smith et al., 1987). In tissues, Co is normally found associated with vitamin 12 or cobalamin in all animals that require preformed vitamin B12. Cobalt in most tissues is low (picomolar concentrations), with liver, heart, and bone containing the highest tissue levels. Low normal serum cobalamin is approximately 2 to 3 ng cobalamin/ml (1.5 to 2.3 nmol/liter). In contrast to Zn, Cu, and Fe, Co does not accumulate with fetal age and it is not stored to any appreciable degree in the adult animal (Ammerman and Goodrich, 1983; Keen, 1996). With toxicity, tissue Co can increase over 10-fold in cattle (Barceloux, 1999; Domingo, 1989; Lauwerys and Lison, 1994). TABLE 22-3 Potential Causes of Trace Element Deficiencies Cobalt is novel because there is no evidence that any organism needs the cobalt ion, either in the free form or as a simple protein complex. Cobalt in the form of a specific complex, vitamin B12 or one of the cobalamides, is essential for animals and many bacteria. Plants contain cobalt, but there is no evidence that it occurs as a cobalamide. Vitamin B12 is also unique among vitamins in that plants do not produce it. The role of rumen microflora in the economy of ruminant animals makes ionic cobalt of particular significance to this group of animals. Although one cannot dismiss the possibility that some organisms require cobalt other than that in a corrinoid (B12-related) complex, there is no such evidence at present and this discussion will hinge primarily around the metabolism and metabolic function of cobalt as it exists in the cobalamides. In aqueous solution, Co is generally in the + 2 or + 3 oxidation state. Cobaltous ion forms complexes with both octahedral and tetrahedral geometry (Burgess, 1999; Kerber and Goldberg, 2006). All Co complexes are octahedral and most involve nitrogen as the electron donor. The skeletal structure of the cobalamides can be visualized by representing the corrin ring of cobalamides with a planar ring and the ligands by X and Y (see Chapter 23). Both the cobalamides and cobaloximes (simpler cobalt corrin-like structures) act as the catalytic site for intramolecular mutations and single carbon transfer reactions (Frausto da Silva and Williams, 1991). Such reactions are important to tissue and cellular growth; as such, Co is primarily associated with erythropoiesis, granulopoiesis, and glucose homeostasis. Two important enzymes that require vitamin B12 as a cofactor are (1) methylmalonyl-CoA mutase, which catalyzes the molecular rearrangement of methylmalonyl-CoA to succinyl-CoA: and (2) 5′-methyltetrahydrofolate-homocysteine methyltransferase, which demethylates 5′-methyltetrafolate and regenerates methionine and tetrahydrofolate. The former reaction is critical for glucose homeostasis in ruminants because a primary gluconeogenic precursor for these animals is propionic acid. Because propionic acid has three carbons, propionyl-CoA cannot enter b-oxidation nor the citric acid cycle; thus, in most vertebrates it is carboxylated to D-methylmalonyl-CoA, isomerized to L-methylmalonyl-CoA, and rearranged to yield succinyl-CoA via the vitamin B12-dependent step shown previously. Succinyl-CoA is an intermediate of the citric acid cycle and can be readily incorporated there. Another aspect of Co in mammalian systems is the potential pharmacological effect of high doses of Co on erythropoietin production (Katsuoka et al., 1983). Whether physiological concentrations of Co influence erythropoietin production is not known. Dietary requirements (as B12) in most animals are usually met either by ingestion of animal tissues or products or by coprophagy. Because of the rumen microflora, ruminants can be fed ionic Co, and the microbes will synthesize cobalamin for absorption. Nevertheless, the relative inefficiency of vitamin B12 production in the rumen and poor absorption of B12 predispose ruminants to deficiency. Between 2 and 5 mg/day (1.5 to 3.9 μmol/day) of CoCl for sheep and 20 to 30 mg/day (15.4 to 23.1 μmol/day) for cattle are required for normal B12 production (Kennedy et al., 1995; Kincaid et al., 2003). In rats, 80% of orally administered Co appeared in the feces. High amounts of iron in the diet can depress Co absorption and vice versa. Co absorption is enhanced in iron deficiency (Domingo, 1989). Excretion of Co is primarily via the kidneys and is linearly related to the ingested dose. Additional sites of excretion have been reported to be the large intestine in chicks and the bile and small intestine in mammals. Absorption of vitamin B12 depends on normal gastric parietal cell synthesis of intrinsic factor and a healthy ileal mucosa for the binding and transport of the vitamin B12. Response to therapy and a ration containing less than 0.08 mg Co/g (1.4 μmol/g) of diet is diagnostic of Co deficiency in ruminants. In sheep, hepatic vitamin B12 levels of less than 0.1 μg/g (0.07 nmol/g), or serum levels of less than 0.3 ng/ml (0.2 nmol/liter), are associated with impending or frank deficiency. Thus, vitamin B12 status can be used to assess Co adequacy in sheep. Although measurement of plasma cobalamin levels has been considered sufficient for assessment of Co status in sheep, it has been suggested that liver cobalamin should also be included in assessment studies as plasma levels do not always reflect soft tissue levels (Mills, 1987). In this regard, it should be considered that the measurement of plasma cobalamin is complicated by the presence of cobalamin analogues that interfere with the assay (Halpin et al., 1984). Therefore, the measurement of plasma methylmalonate levels is used as an indirect indicator of the functional cobalamin status of the animal. The increase in methylmalonate is due to a reduction in the activity of mehylmalonyl-CoA mutase, for which adnosylcobalamin is a cofactor (discussed earlier). The signs and biochemical lesions that are manifested in Co deficiency are referable to a deficiency of vitamin B12. The pathways that are most severely impaired are those of purine biosynthesis and glucogenesis. Deficiencies of Co have been correlated with a reduction in blood glucose and an increase in methylmalonic acid excretion. A primary defect in Co deficiency underlying the previous discussion has been attributed to a reduction in the activity of methylmalonyl CoA mutase in ruminants. The hepatic lipidosis observed in Co-deficient animals has been attributed to a reduction in choline biosynthesis caused by a reduction in the activity of homocysteine methyltransferase. Vitamin B12 deficiency reduces the regeneration of methionine, which can impair choline biosynthesis. With a reduction in the activity of this enzyme, Co deficiency can result in a reduction in the regeneration of tetrahydrofolic acid, which can then result in the development of megaloblastic anemia and pancytopenia secondary to impaired purine biosynthesis. A reduction in tetrahydrofolate reduces the available methyl donors and therefore reduces purine biosynthesis (see Chapter 23). The primary signs of Co deficiency are referable to pernicious anemia, granulocytopenia, and chronic wasting, which is secondary to aberrations in amino acid, nucleic acid, carbohydrate, and potentially, lipid metabolism. Another disorder, white liver disease, is a Co-responsive disease of sheep that is characterized by unthriftiness, listlessness, weight loss, ocular discharge, anemia, pale liver, and occasionally, photosensitivity and neurological dysfunction (Kennedy et al., 1997; Mitchell et al., 2007; Smith et al., 1987). Toxicity in animals under natural conditions has not been reported. Toxic levels appear to be at least 300 to 1000 times the requirement in most species. Cases of toxicity are invariably the result of accidental oversupplementation to prevent deficiency or inhalation of Co sources. Doses in excess of 4-mg/kg body weight (68 μmol/kg) can be toxic for sheep and ingestion of greater than 1.1 mg/kg (18.7 μmol/kg) in cattle can be toxic. The major clinical signs include polycythemia, anorexia, fatty infiltration of the liver, pulmonary edema, and depressed grow. Noteworthy, cases of Co intoxication from ingestion of beer have been reported in humans (Barceloux, 1999; Lauwerys and Lison, 1994). In humans, signs of Co toxicity are hyperthyroidism, thyroid hyperplasia, cardiomegaly, and heart failure. Symptoms related to inhalation toxicosis are mostly referable to the lungs and skin with hypersensitivity, dermatitis, and pulmonary fibrosis being the major lesions (Mitchell et al., 2007; Watson, 1998). A large animal can contain 50 to 120 mg (780 to 1889 μmol) of Cu. In the adult, about one-third of the total body Cu is found in the liver and brain. Most nonruminant species have liver Cu concentrations that are between 2 and 10 μg/g (0.032 to 0.16 μmol/g). Skeletal muscle, although considered low in Cu, represents about one-third of the total body Cu because of its mass. The remainder of the Cu is fairly evenly distributed throughout the body. The highest concentrations of Cu (>5 μg/g; >0.079 μmol/g) are normally present in liver, brain, heart, and hair. Tissues with intermediate levels or Cu (1.5 to 5 μg/g; 0.024 to 0.078 μmol/g) include kidney, pancreas, skin, muscle, and bone. Tissues with low concentrations of Cu (<1.5 μg/g; <0.024 mol/g) include pituitary, thyroid, thymus, ovary, and testis (Keen et al., 2003; Mason, 1979; Smart et al., 1981; Stern et al., 2007). The normal range of Cu in the blood of most healthy animals is between 0.5 and 1.5 μg/ml (7.9 to 23.6 μmol/liter). In most species, plasma/serum and whole blood Cu concentration are similar. Birds, fish, and marsupials are characterized by blood Cu concentrations that are about half that of other species (Mason, 1979). Ruminants have a high storage capacity for Cu in their livers, often exceeding two-thirds of the total body pool. Typical liver Cu concentrations in sheep and cattle range from 20 to 150 μg/g (0.315 to 2 to 36 μmol/g). Similarly high liver Cu levels have been reported for ducks and some fish. The high liver Cu in ruminants compared to nonruminants is thought to reflect a higher retention rather than a difference in dietary intake of Cu or absorption. Copper concentrations in other ruminant tissues are similar to those reported for nonruminants. For most species, the newborn is characterized by liver Cu concentrations that are markedly higher than those found in adults. However, the Cu in most other tissues tends to be higher in the adult than in the newborn (Stern et al., 2007). It has been suggested that the high liver Cu in the newborn can represent a reserve pool of Cu for the rapidly growing neonate. Several cases of Cu deficiency have been reported in premature infants. In sheep, liver Cu in the newborn is lower than in adults, and in cattle, newborn and adult liver Cu is similar (Keen, 1996). For all species studied, the pigmented tissues of the eye are particularly high in Cu, with very high levels associated with the melanins. The function of this Cu in the eye is not known. Except in certain disease states and in deficiency, the body Cu content is homeostatically controlled, and there is little accumulation of Cu in the eye (Krajacic et al., 2006). Copper has two major functions. It can be a structural component in macromolecules acting as a coordination center. It is also a common redox cofactor for a number of oxidases and monooxygenases that are essential for life, owing to its ability to cycle between reduced and oxidized states. Perturbations in the activity of these enzymes because of poor Cu dietary status can be linked to specific biochemical steps and lesions. For example, poor growth, reproduction, skeletal, and vascular formation can result from a lack of lysyl oxidase and cytochrome C oxidase. Impaired immunity, neurological function, oxidant defense, and depigmentation resulting from Cu deprivation can also be linked to specific enzymes such as Cu-Zn superoxide dismutase (CuZnSOD), which catalyzes the dismutation of the superoxide anion; dopamine β-hydroxylase, responsible for noradrenalin and adrenaline production; and melaninase, responsible for melanin production (Keen et al., 2003; Rucker et al., 1998; Stites et al., 2000; Tinker and Rucker, 1985). A diverse array of physiological symptoms, particularly during the perinatal period, can occur including hypotension, muscle hypotonia, hypothermia, and hypoglycemia. Moreover, elastin and collagen from Cu-deficient animals have an elevated content of lysine and a low content of various cross-linking amino acids. Loss of cross-linking results in defects in the elastic properties of arteries and decreases in bone strength and the tensile strength of various connective tissues. A reduction in CuZnSOD can increase hydrogen peroxide and superoxide radicals that can irreversibly oxidize proteins, nucleic acids, lipids, and carbohydrate components within cytoskeletal structures and the cell wall. Changes in Cu status, particularly in the fetus and neonates, have been associated with perturbations in nitric oxide (NO) metabolism, a key signaling molecule to endothelial cell responsiveness (e.g., contraction and relaxation). Moreover, when an increase in hydroxyl radical occurs, because of a reduction in CuZnSOD activity, the reaction of peroxide radicals with NO can produce peroxynitrite. Peroxynitrite, another potent oxidant, can cause ATP depletion and peroxynitrite-induced nitration of tyrosine residues on proteins. Many of the neurological signs and endothelial changes associated with Cu deficiency are thought to be the result of altered NO metabolism (Schuscha, 1997; Yang et al., 2007) and peroxynitrite-induced lesions (Fig. 22-3). Other Cu-containing enzymes include tryptophan oxygenase, ascorbate oxidase, tyrosinase, amine oxidases, peptidyl-glycine-α-amidating monooxygenase, and possibly some fatty acid desaturase enzymes such as C18 Δ°-desaturase. It has been suggested that Cu can also be involved in a nonenzymatic manner in neuropeptide release from the brain. Copper absorption from diets is relatively efficient, although some dietary constituents can affect bioavailability. Copper hydroxides, iodides, glutamates, and citrates are more easily absorbed than molybdates, sulfates, and phytates. High intakes (100 or more mg/kg of diet) of Ag and Zn can interfere with intestinal copper transport. Moreover, the extended use of supplements that contain iron can negatively affect copper status. Cu absorption is greater in neonates than in adults (Committee on Copper in Drinking Water, 2000; Stern et al., 2007). Another interaction that has attracted attention involves Cu, Mo, and sulfate. Particularly in ruminants, dietary sulfate intensifies the harmful effects of Mo on Cu absorption. CuSO4 and Na2MoO4 react to form an insoluble complex referred to as a thiomolybdate, which renders Cu biologically less active and less bioavailable (see Section V). Nutritional Cu deficiency occurring outside of the laboratory has been well documented in a variety of species including humans, cattle, sheep, pigs, and horses. The recommended minimal daily requirements for Cu for a number of species are presented in Table 22-2. Given that the uptake of Cu from a diet can be influenced by other dietary factors as well as the physiological state of the animal, under some conditions, a diet cannot contain sufficient Cu for the animal even though the level of Cu in the diet is at the level suggested in the NRC tables. Food items that are high in Cu include nuts, dried legumes, dried vine, and dried stone foods (300 to 400 μg/g; 4.72 to 6.30 μmol/g). Food items considered low in Cu content (<1 μg/g; 0.016 μmol/g) include dairy products and sugar, refined cereals, fresh fruits, and nonleafy vegetables contain about 7 μg Cu/g (0.11 μmol/g). Copper in typical animal feeds can range from 20 μg/g (0.315 μmol/g) (cottonseed meal) to 2 (0.032 μmol/g) (corn), with the concentration being highly dependent on soil conditions and fertilizer practices. Copper is absorbed in all segments of the gastrointestinal tract. For most species, absorption occurs in the upper small intestine, but in sheep considerable absorption also occurs in the large intestine. Absorption of Cu is about 30% to 60% with a net absorption of about 5% to 10% owing to the rapid excretion of newly absorbed Cu into the bile. A delicate balance between Cu uptake and efflux maintains copper homeostasis (Cromwell et al., 1989; Gooneratne et al., 1989; O’Dell and Sunde, 1997; Reilly, 2004; Stern et al., 2007; Theile, 2003). Cu uptake occurs through both high- and low-affinity transport systems (Fig. 22-4). Environmental factors can influence the response to transporters. Most important are factors that influence solubility and redox state. Cu exists in two different valence states; the cupric ion (Cu+1) is the primary substrate for the transport systems that take Cu across plasma membranes. Reduction (Cu+2→Cu + 1) is catalyzed by plasma membrane reductases (Theile, 2003). However, the cuprous ion (Cu+2) in the intestinal lumen is more soluble than the cupric ion (Cu + 1). Chemical reduction of luminal contents (e.g., by reducing agents such as ascorbic acid) can decrease the amount of Cu that is bioavailable (i.e., affectively delivered to the surface of intestinal cells). It is important, however, to note several caveats. Observations in humans suggest that the effects of ascorbic acid on Cu absorption are modest and probably occur only at the extremes of ascorbate intake (Jacob et al., 1987; Lonnerdal, 1998; Stern et al., 2007). FIGURE 22-3 Major sources and regulation of reactive oxidant species (ROSs) in cells. Depending on the stage of development and condition, on a per mole basis ROSs are normally generated at rates of 1% to 4% of the oxygen consumed or greater when ROS regulation is disrupted. A major source of ROS is derived from superoxide anion (O2–) generated in the mitochondrion (A) because of uncoupling from the cytochrome oxidase system during mitochondrial electron transport. In addition to water and oxidized metabolites as products, superoxide anion and H2O2 are produced. Enzymes, such as catalase and MnSOD (localized in the mitochondria) control excess production of O2– and H2O2. When there is subsequent leakage from mitochondria, an increase in the cellular pool of ROS occurs owing both to ROSs from mitochondria and production from other organelles and cytosol (B). Sources of ROSs from other organelles and cytosol are the products from reactions catalyzed by NADH and NAPH oxidases, xanthine oxidase (activated during ischemic injury), lipoxygenase, and P450 monooxygenase enzymes (localized mainly in the smooth endoplasmic reticulum and responsible for the metabolism of xenobiotics, drugs, and secondary metabolites). In addition, excess ROS can potentially alter nitric oxide metabolism (e.g., formation of peroxynitrites) and cause the generation of organic and lipid peroxides and so-called Fenton products (C). For example, “RH,” used to depict numerous aromatic and lipid-derived possibilities as potential reactants, can be converted to ROOH, potential organic peroxide-containing products. Excess superoxide anion, ROOH, OH-, OH¨, and other ROSs can then damage proteins, nucleic acids, and lipids, particularly lipid structures in cell and organelle membranes. Important to this discussion, metals can act as both catalysts for ROS formation and cofactors for antioxidant enzymes that modulate influence ROS metabolism. For example, (1) MnSOD and CuZn SOD (superoxide dismutases) cause the dismutation of excess superoxide anion to peroxide, (2) catalase (contains Fe) and glutathione peroxidase (contains Se) can control excess of both hydrogen and organic peroxides. As an additional defense, metal chaperones (see text), metallothionein (MT), or ferritin control “free” metal ion concentrations in cells that are capable of redox (D). Redox metals (Fe or Cu) can act as Fenton catalysts and promote the homolytic cleavage of H2O2 to OH¨ and OH-, which are highly damaging oxidants. Reduction in the concentration or sequestration of metals (depicted by the dashed line) markedly reduces potential Fenton products. As a final line of defense, tocopherols and polyphenolic and related phytochemicals, which localize to lipid membranes, provide additional ROS defense. FIGURE 22-4 Cellular transport. Features important to the transport of copper, manganese, selenium, and zinc are summarized. For features related to cobalt (and vitamin B12), refer to the vitamin chapter and for molybdate see Figure 22-5. (a) Dietary copper is presented to intestinal cells in the form of protein and amino acid complexes, and in plasma, Cu is bound to a number of proteins; however, it is most often associated with albumin and ceruloplasmin. The transport and cellular metabolism of Cu depend on a series of membrane proteins and smaller soluble peptides that constitute a functionally integrated system for maintaining cellular Cu homeostasis. The high-affinity copper transporter (CTR1) of the plasma membrane mediates nearly all Cu uptake under low copper conditions. It is one of a family of proteins involved in Cu transport that is transcriptionally induced at low copper levels and degraded at high copper levels. Associated with this transporter is a copper reductase that maintains Cu in the + 1 state (its most soluble form) while in the vicinity of the transporter. Next, Cu is transferred to chaperones whose functions are to carry copper to specific proteins within the cell (e.g., COX-17→cytochromes, ATOX→vesicular P-ATPases, CCS→SOD). Copper egress (efflux) is accomplished by a novel process, the transport of copper into secretory vesicles via post-Golgi processing. This occurs coincidently with efflux of specific apocuproproteins (see text) that are localized to the same vesicles. On the membrane of the vesicles, two membrane-bound Cu-transporting ATPase enzymes, ATP7A and ATP7B, catalyze an ATP-dependent transfer of Cu to intracellular compartments or expel Cu from the cell (from the Golgi to and from the cell membrane). In response to a high level of cellular Cu, there is recycling of the vesicles at higher rates to more effectively remove copper. Within the vesicles, apocuproproteins can also become activated. Consequently, secreted cuproproteins with enzyme activity, such as lysyl oxidase or ceruloplasmin, often reflect Cu status or dietary intake. Some evidence also suggests DMT or Nramp transporters important to iron transport can play a minor role in copper uptake. (b) Intestinal and systemic cellular manganese transport is mediated mostly by divalent metal transporter 1 (DMT1) and is up-regulated in iron deficiency. Within the body, Mn bound to transferrin is taken up by transferrin receptors. Unlike other transition metals, which are not found in “free” ion forms in cells, the behavior of Mn is analogous to Mg (i.e., dissociable ion exists). Less is know currently about specific chaperones than for copper or zinc. Excess Mn in cells is sequestered on ferritin. A Golgi-derived ATPase has been described to facilitate the movement of Mn from and to the nucleus and cis- and trans-Golgi compartments. Although the evidence is indirect that other types of ion channels and vesicular egress play a role in Mn cellular transport, given that MnO4 anion can be transported in addition to Mn+2 and Mn+3, a role for oxyanion transport is indicated. (c) Selenium is delivered to cells via amino acid and oxyanion transporters and when present in plasma via processes that recognize selenoprotein P. The selenite and selenate forms must first be reduced (via a glutathione reduction system) to HSe- before Se can be utilized as a cofactor. Selenomethionine, if not incorporated into protein, can also be eventually converted to HSe-. Next, for incorporation into specific Se-proteins (e.g., GPx, 5′-ID, or Se-protein P), HSe- is phosphorylated (requires ATP). Then following transfer to Ser-tRNAUGA to form Se-Cys-tRNAUGA, the stage is set for translation of Se-containing proteins. Regarding cellular efflux, Se is lost from cells as secreted Se-proteins, such as selenoprotein P, Se-cystathionine, or as volatile forms of methylated Se (e.g., CH3-Se-CH3). (d) Zinc uptake and cellular translocation are controlled by two large families of metal transporters for which there more than two dozen variants. More specifically, the two solute-linked carrier (SLC) gene families encode the zinc transporters: ZnT (SLC30) and Zip (SLC39). The ZnT transporters reduce intracellular zinc availability by promoting zinc efflux from cells or into intracellular vesicles, whereas Zip transporters increase intracellular zinc and promote extracellular zinc uptake. The ZnT and Zip transporter families exhibit unique tissue-specific expression and differential responsiveness to dietary Zn intake and to physiological stimuli. Temporary influxes of Zn are buffered by the induction of metallothionein. DMT1 and over-ion channels can play minor roles in Zn transport. In addition to the transporters, cellular chaperones specific for Cu deliver Cu to specific cellular proteins (Fig. 22-4). Other important features of Cu regulation include the role of metallothionein, a divalent metal-binding protein for Cu, Zn, Hg, and Cd, which acts to buffer shifts in the cellular concentrations of Cu (and Zn). Cu egress or transport out of cells is controlled by P-ATPase Cu-transporters that are located on the surface of vesicles that arise from Golgi processing. Although a change in Cu status does not appear to alter Cu-transporting P-ATPase gene expression, it can affect the movement of copper-containing vesicles to and from that outer cell membrane. Cu homeostasis must be coordinated, as the release of free Cu ions can cause damage to cellular components by catalyzing the generation of reactive oxidant species (ROS). Free cuprous ions (and ferric) react readily with hydrogen peroxide to yield deleterious hydroxyl radicals. Accordingly, Cu homeostasis is regulated tightly, and unbound Cu is extremely low in concentration (∼one atom/cell). For example, Atox1, a chaperone that delivers Cu into egress or efflux pathways, docks with a Cu-transporting ATPase (ATP7B in the liver or ATP7A in other cells). ATP7B directs Cu to plasma ceruloplasmin or to biliary excretion in concert with another chaperone, Murr1, the protein missing in certain types of canine Cu toxicosis. ATP7A directs Cu within the trans Golgi network to the proteins dopamine β-monooxygenase, peptidylglycine α-amidating monooxygenase, lysyl oxidase, and tyrosinase, depending on the cell type (Stern et al., 2007; Theile, 2003). From the intestine, a case can be made for the transport of Cu on albumin and in the form of low-molecular-weight complexes (e.g., histidine) to target tissues, particularly the liver. From the liver, ceruloplasmin seems to aid in the transport of Cu to other tissues. Ceruloplasmin is the predominant Cu-containing protein in mammalian serum, a glycosylated multi-Cu ferroxidase that carries >95% of total serum Cu. Surprisingly, whereas ceruloplasmin can function in Cu transport, the absence of ceruloplasmin has not been shown to alter Cu levels in the peripheral tissues. Such observations come from what is known about individuals and animal models that have aceruloplasminemia, a genetic disorder of ceruloplasmin deficiency. Moreover, analbunemic rats do not have significantly impaired Cu metabolism. Apparently Cu movement from serum proteins to the cell surface reductase-Ctr transporters is not highly specific (Hellman and Gitlin, 2002). A number of pathologies are associated with Cu deficiency that represent perturbations in the functions of Cu outlined earlier. For example, anemia (microcytic hypochromic or normocytic hypochromic) is probably the most frequent sign associated with chronic Cu deficiency. Cu deficiency results in impaired normal iron absorption (O’Dell and Sunde, 1997), mobilization, and utilization, partly because of Cu’s role as a redox cofactor in various membrane associated ferrioxidases that oxidize Fe+2 and Fe+3, thereby promoting the transfer of iron to transferrin. Reduced aminolevulinic acid dehydrase, important to the first step in heme synthesis, is also decreased in Cu deficiency. Heinz body anemia caused by ROS can also develop with a significant depression of CuZnSOD activity (O’Dell and Sunde, 1997). Cardiovascular defects have been associated with Cu deficiency since the 1950s. In cattle, Cu deficiency can result in severe degeneration of the myocardium with fibrosis (“falling disease”). Sudden death is a frequent observation and is thought to be due to acute heart failure. Cardiac failure associated with cardiac hypertrophy has also been reported in Cu-deficient rats. A number of biochemical lesions can underlie the changes in the heart observed with Cu deficiency (e.g., decreased cytochrome oxidase activity, abnormalities in cardiac and vessel wall elastin and collagen structure because of lysyl oxidase, and low cardiac norepinephrine levels, which can decrease coronary resistance and reduce systolic pressure) (Tinker and Rucker, 1985). In experimental Cu deficiency in young animals, such as turkey poults, aortic aneurysms can occur (Savage et al., 1966). This is the result of poor biomechanical properties of elastic fibers (constitutes as much as 50% of the total protein in aorta) that are weakened as a result of defective and reduced cross-linking. Such elastin is also susceptible to degradation and lost. Contrast this condition with normal elastin that normally has a biological half-life best measured in months to years (Tinker and Rucker, 1985). In addition, skeletal defects have been reported in Cu-deficient dogs, sheep, chicks, cattle, foals, and humans. The primary biochemical lesion underlying development of bony lesions in Cu-deficient animals is again a reduction in the activity of lysyl oxidase leading to a reduction in the cross-linking of bone collagen, thus reducing bone stability and strength analogous to the situation with elastin (Tinker and Rucker, 1985). An increased rate of tissue lipid peroxidation is another mechanism by which Cu deficiency can contribute to a wide variety of lesions. In addition to the depression in CuZnSOD activity, Cu deficiency can reduce the activity of selenium(Se)-dependent glutathione peroxidase (GPx). Thus, two major components of the cells’ antioxidant defense system can be affected by Cu deficiency. Another lipid alteration is hypercholesterolemia (Engle et al., 2000; Gooneratne et al., 1989; Keen et al., 2003; Mills, 1987; Schuscha, 1997). In Cu-deficient animals, total cholesterol and free cholesterol levels are elevated and are associated with high concentrations of high- and low-density lipoproteins (HDL and LDL). The lipid composition of HDL isolated from Cu-deficient animals has been reported to be similar to that of Cu-supplemented animals, but the HDL has been shown to be enriched in apo F. A primary biochemical lesion underlying the hypercholesterolemia is a reduction in hepatic HDL binding that results in a slower turnover of HDL and leads to an accumulation of apo E-rich HDL. Both of these phenomena, lipid peroxidation and abnormal lipid transport, are important features that underlie abnormal membrane function.
I. INTRODUCTION
A. General Properties of Minerals
Subject to Natural
Deficiencies
Experimentally
Produceda
Cobalt
Arsenic
Copper
Chromium
Iodine
Fluoride
Iron
Nickel
Manganese
Silicon
Molybdenum
Vanadium
Selenium
Lithium
Zinc
Boron
B. Typical Configurations of Metal Complexes
C. Biological Perspectives
1. Trace Elements as Essential Components in Evolution and Animal Diversity
2. Utilization of Trace Elements and Metabolic Regulation and Metabolism
II. COBALT
Cause
Mechanism
Food processing
Loss of elements because of refining
Dietary interactions
Competitive interactions between elements
Drug interactions
Impaired absorption or increased excretion; redistribution of elements among tissue pools; decreased absorption (chelators, laxatives); increased excretion (diuretics)
Disease or genetic
Increased requirement because of a block in the normal metabolism of the element (primary); increased requirement because of decreased absorption or increased excretion secondary to the disorder (secondary)
A. Cobalt Function
B. Absorption and Transport
C. Disorders of Cobalt Metabolism
III. COPPER
A. Copper Distribution
B. Copper Functions
C. Dietary Copper
D. Copper Metabolism, Absorption, and Transport
1. Cellular Transport and Regulation
2. Systemic Regulation of Cu
3. Disorders of Cu Metabolism-Cu Deficiency
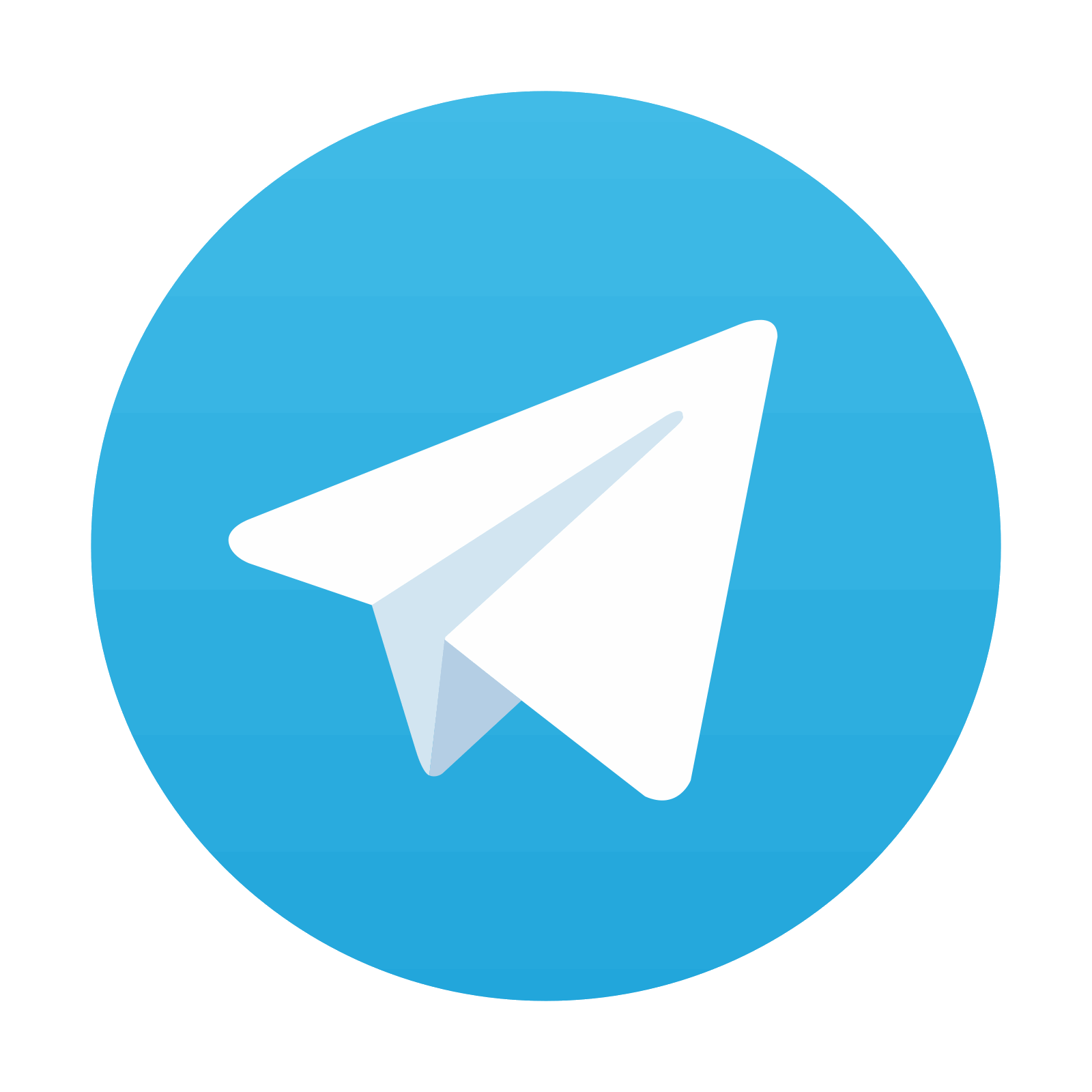
Stay updated, free articles. Join our Telegram channel
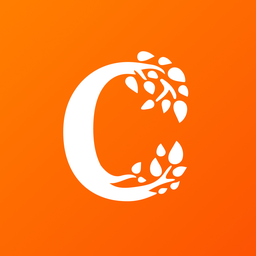
Full access? Get Clinical Tree
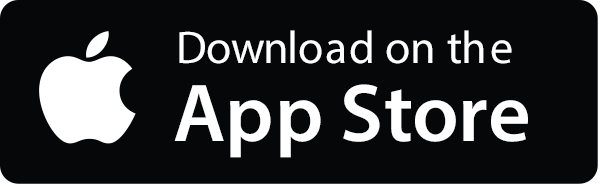
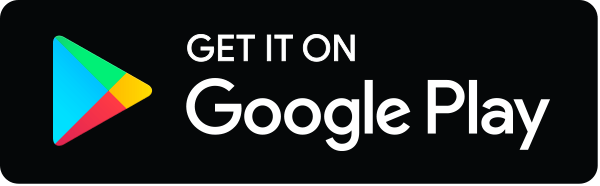