CHAPTER 8 Exercise requires derivation of energy for muscular contraction from the conversion of stored chemical energy to mechanical energy. This process is relatively inefficient, and about 80% of the energy released from energy stores is lost as heat. Effective dissipation of this heat load is required if life-threatening elevations in body temperature are to be avoided. The physiologic mechanisms that result in this heat dissipation, governed by the thermoregulatory system, are essential if the horse is to function as an athletic animal (Åstrand and Rodahl, 1979; Brody, 1945). The primary means of heat dissipation in the horse is evaporation of sweat, particularly in warm ambient conditions. Evaporative cooling is an efficient mechanism enabling horses to perform a variety of athletic events, with only relatively minor elevations in body temperature. However, exercise-induced heat stress can occur when heat production during exercise exceeds heat dissipation and body temperature reaches critical levels. This is likely when animals are forced to exercise in adverse environmental conditions (i.e., high temperature and humidity), when they have been inadequately conditioned, or when they are suffering an impairment of the thermoregulatory system (anhidrosis) (Hafez, 1986; McCutcheon and Geor, 1998). Careful preparation for athletic events, monitoring during events, and early recognition of impending signs of heat stress will minimize the risk of development of life-threatening hyperthermia. Examples of the effectiveness in devising strategies for horses coping with a combination of adverse environmental conditions with large exercise-induced heat loads occurred prior to the Olympic Games in Atlanta. Equestrian events were held in the summer when heat and humidity were high. By utilizing acclimatization strategies, advanced cooling techniques, conducting events when ambient temperatures and solar heat gain was minimized, and modifications to the length of competition, heat illness was avoided in horses performing in those competitions (Jeffcott and Kohn, 1999). Horses have coped with temperatures as variable as 58°C in Northern Australia to at least –40°C in western Canada, Scandinavia, and Russia. Despite large fluctuations in environmental temperature, horses are able to maintain their internal body temperature within a very narrow range by elaborate thermoregulatory mechanisms. The basis of this thermoregulatory control mechanism is via alterations in blood flow that allows regulation of heat flow between the animal and its environment (Cymbaluk and Christison, 1990). Heat will flow from one area to another by four basic mechanisms: (1) radiation, (2) convection, (3) conduction, and (4) evaporation (Monteith, 1973; Yousel, 1985). Homeothermy requires that heat produced or gained from the environment equals heat loss to the environment, as indicated by the following equation (Yousel, 1985): Radiation involves the movement of heat between objects without direct physical contact via electromagnetic radiation of two distinct types: (1) Short–wave, or solar, radiation is received from the sun by any object exposed to sunlight. (2) Long–wave radiation is emitted and absorbed by the surfaces of all organisms and relates to heat interchanges between an animal and its surroundings (Åstrand and Rodahl, 1979). Convection occurs within all fluids (including gasses such as air) due to the mixing of particles within that milieu. Temperature differences within the fluid result in a difference in the density of the fluid particles. Warm particles are less dense and will rise, whereas cold particles fall. Free convective heat transfer takes place at the surface of a solid body within a fluid medium, which is at a different temperature. This type of transfer takes place continuously between the surface of the body and the surrounding air. Forced convective heat transfer occurs if there are fluid movements induced by gross pressure differences, for example, caused by wind blowing across the body surface. Free convection at the skin surface can result in significant heat losses if ambient air temperatures are low (Åstrand and Rodahl, 1979). A hair coat will entrap a layer of air close to the skin and resist convective heat transfer; this is the purpose of a hair coat. Wind will increase forced convective losses by disrupting this insulating layer. The hair coat of the horse is generally fine and sleek in summer to aid heat loss, whereas in winter a thick hair coat develops, in association with acclimatization to cold stress, resulting in increased insulation. Direct transfer of heat between surfaces that are in contact occurs as a result of conduction (Åstrand and Rodahl, 1979). In the standing horse, most of this transfer is to air, which has poor thermal conductivity, and thus, conductive heat transfer plays a small role in the total heat balance. However, this route of heat transfer can become significant if the animal is lying on a cool or wet surface or, indeed, if the horse is repeatedly bathed in a cold fluid such as water. A number of behavioral strategies are utilized by animals to affect conductive heat exchange. Changes in posture can alter the surface area available for heat exchange. For example, by lying down and drawing the limbs close to the body, the surface area can be reduced considerably. In contrast, morphologic adaptation by burros and mules (long ears, short legs, and lean body conformation) increases available surface area for convective heat loss and may help increase heat tolerance (Bligh, 1973). The extremities of animals, the limbs and head, have a high surface area to mass ratio, and thus, maximal conductive heat exchange can occur at these sites. Changes in surface temperature at these sites can be affected by alterations of skin blood flow. The skin temperature of the horse, reflecting skin blood flow, varies in direct proportion to ambient temperature, with greatest temperature changes occurring at the extremities (Bligh, 1973). At low ambient temperature, local vascular shunts direct blood away from the extremities to reduce the rate of convective heat loss, whereas at high temperatures, vasodilation occurs to promote heat loss. An example of how horses utilize this mechanism is seen when skin temperature falls from 26°C at an ambient temperature of 25°C to 17°C at 15°C (ambient) and even further vasoconstriction occurs at an ambient temperature of 5°C, with skin temperature decreasing to 10°C (McCutcheon and Geor, 1998; Rowell, 1986). Evaporation is the principal means by which homeotherms such as horses lose heat in warm environments via the physiologic processes of panting, sweating, and insensible perspiration. The conversion of water from liquid to vapor is an endothermic process. Thus, evaporation of water at the surface of the body results in heat loss. The exact amount of energy involved in this process is dependent on the temperature and vapor pressure of the surrounding air. The latent heat of vaporization of 1 g of water is 598 calories (cal) (2501 joules [J]) at 0°C and 575 cal (2406 J) at 40°C. Evaporation of 1 liter (L) of sweat in a human being can remove 580 kcal (2428 kilojoules [kJ]) from the body (1 cal = 4.186 J) (Åstrand and Rodahl, 1979). During panting, inspired air is almost fully saturated with water at a temperature similar to the deep body temperature as it passes over the wet surfaces of the upper respiratory tract. The associated heat loss is governed by the ambient air humidity and respiratory ventilation rate. Although horses do not pant routinely, there is evidence of considerable evaporative heat loss from the respiratory tract during exercise in response to normal ventilation (Hodgson et al, 1993). Insensible perspiration involves the passage of water through the skin by processes other than sweating. The skin is not impermeable to water, and thus, water can diffuse out as a result of the skin–ambient vapor pressure gradient. In humans, approximately 10 milliliter per square meter per hour (mL/m2/hr) of water passes through skin in ambient conditions, increasing to 30 mL/m2/hr in a warm environment. The average human has a body surface area of 1.5 to 2.0 m2 (Åstrand and Rodahl, 1979). Thermoregulatory mechanisms maintain the body temperature of homeotherms within a narrow range by regulating heat production and heat loss. A complex neurophysiologic process regulates internal body temperature. The principal neuronal elements of the thermoregulatory system are peripheral thermoreceptors, the spinal cord, and the hypothalamus. Peripheral thermoreceptor organs are located in a number of locations, including skin, the buccal cavity, skeletal muscle, the abdomen, regions of the spinal cord, the medulla oblongata, and preoptic–anterior hypothalamic region of the midbrain. These temperature-sensitive structures are responsible for detection of a disturbance and produce proportional nerve impulses. For information from thermosensitive receptors in several parts of the body to be translated into appropriate instructions to the effector organs, there must be convergence of the neural pathways from these temperature sensors and transmission of this information to an interpretive center. A coordinating center in the central nervous system receives afferent nerve impulses and produces efferent impulses, initiating a correction that is transmitted to the effector organs. There is considerable evidence from experiments in a variety of animal species that the hypothalamus may represent this interpretive center. Thermal stimulation of peripheral temperature sensors, the spinal cord, and the hypothalamus results in appropriate thermoregulatory effector activity. However, destruction of the hypothalamus downregulates and even turns off thermoregulatory responses to local heating of all these structures. This may be interpreted to suggest that the integrity of particular hypothalamic areas is necessary for normal thermoregulation and that most of the regulation of internal temperature occurs as a function of the hypothalamus. Once the hypothalamus signals the effector organs, these end organs are responsible for correction of the initial disturbance, which, in turn, results in reduction of stimulation by the sensory organs as thermoregulation is effected (Rowell, 1986). The thermoregulatory system utilizes various mechanisms of heat flow to effect heat loss from the body. Highly effective mechanisms for dissipating heat include sweating and panting (or elevated respiratory volume), which exploit the significant heat loss associated with the evaporation of water, in addition to convective heat loss. The cardiovascular system has a critical role in thermoregulation, with blood flow being used as a means for heat transfer from sites of heat production within the body core to areas where dissipation of heat can occur, primarily skin and the respiratory tract (Hodgson et al., 1993). The principal physiologic thermoregulatory mechanisms that utilize the vaporization of water from the body surface are panting or increased respiratory volume and sweating. Hominoidae, including humans, and Equidae (horses, mules, donkeys, etc.) are the only species that depend on sweating as the primary mechanism for thermoregulation, whereas sheep, dog, and pig rely much more on respiratory heat loss (McLean, 1973). Panting or increased respiratory volume has advantages over sweating in that its efficiency is not limited by the hair coat, which can insulate the animal against both radiant heat and the effects of cold, as well as reducing fluid and electrolyte losses in sweat. However, disadvantages of panting exist, particularly during exercise, when there is competition between the need for appropriate gaseous exchange and optimum evaporative heat loss (Ingram, 1975). Sweating is the most important means of heat loss in humans, with respiratory heat loss accounting for only 11% of total heat loss in a thermoneutral environment. This percentage increases with rising core temperature (Åstrand and Rodahl, 1979). Similar to humans, horses rely primarily on sweating for heat loss, with the respiratory tract contributing to heat loss, particularly during exercise. Respiratory frequency has been reported to be dependent on environmental temperature during rest and exercise, increasing 1.9 breaths/min for every 1°C increase in ambient temperature. Investigations of the exact proportion of heat dissipated by the respiratory tract have reported different values depending on the type of horse, the ambient temperature, and the exercise intensity. Ponies at rest in ambient conditions (21 to 23°C) lose 14% to 22% of total heat production by pulmonary ventilation, whereas horses in a cooler environment of 16°C have respiratory heat loss of 38% at rest and 17% during maximal exercise. Although horses do not normally pant, if evaporation of sweat is limited by high humidity or anhidrosis, they may experience heat-induced tachypnea. Pulse–respiration inversion (respiratory rate in excess of heart rate), frequently shown by endurance horses during recovery from exercise in hot humid environments, is likely a form of panting in horses (Geor and McCutcheon, 1996). In horses, rectal temperature is reported to be significantly higher (∼0.5°C greater) in hot environments than in thermoneutral environments. Such temperature variations may reflect an adaptation to desert environments, similar to that seen in camels. To limit the need for sweating, some species inhabiting hot, arid environments have relatively labile body temperatures allowing storage of heat. Body temperatures of the camel may rise to 41°C during the heat of the day and decrease to as low as 34°C during the night. Such thermolability obviates the need for evaporative cooling, thus resulting in a mechanism of conserving water (Bligh, 1973). Heat exchange occurs within the large surface area of the upper respiratory tract. Inspired air is heated to body temperature and saturated with water vapor by the time it reaches the alveoli. During expiration, some heat passes back to the mucosa, with resultant condensation of water. The difference between initial heat transfer to the inspired air and subsequent transfer back to the mucosa is the mechanism of respiratory heat loss. The quantity of heat loss depends on the environmental temperature and humidity; the warmer and more humid the air, the smaller is the heat loss. In some animals, panting increases ventilation rate, resulting in greater heat loss from the respiratory tract (Bligh, 1973). The glands of the general body surface of humans have a primary heat-regulatory function, whereas the glands on the palms and soles are concerned with emotional responses and are insensitive to heat. The sweat glands of the horse resemble the sweat glands of humans in their heat-regulatory function (Carlson, 1983). The ionic concentration of sweat in humans varies markedly between individuals and is strongly affected by the sweating rate and the state of heat acclimatization of the subject. Human thermogenic sweat is hypotonic relative to plasma. Sodium chloride is the main constituent, with the chloride concentration being 30 to 50 millimolars (mM) and sodium concentration 20 to 60 mM (Table 8-1). The concentration of sodium chloride (NaC1) increases as sweat rate increases initially. In fact, increased short-term high-intensity activity results in increases in the concentrations of these electrolytes in serum and sweat. Thus, during short-term strenuous exercise, the NaC1 concentration may increase by up to 50% above initial values. In contrast, prolonged exercise tends to result in decreases in the concentration of electrolytes in sweat (Amatruda and Welt, 1953). Potassium concentration in sweat is only slightly higher than that of plasma at low sweat rates (10 to 35 mM) and decreases to 5 mM as sweat rate increases. Potassium secretion is not affected by acclimatization or dietary intake. Sweat calcium concentration similarly decreases with increasing sweat rate from 3 to 10 mM to 1 to 2 mM. Bicarbonate concentration is 2 to 10 mM and tends to increase with sweat rate. Traces of magnesium, iodide, phosphorus, sulfate, iron, zinc, copper, cobalt, lead, manganese, molybdenum, tin, and mercury are also present, as well as negligible amounts of vitamins (Robinson and Robinson, 1954). In humans, a highly sophisticated mechanism for electrolyte reabsorption is present in sweat glands. An ultrafiltrate of plasma-like isotonic precursor fluid (Na+ = 150 mM, Cl– = 124 mM) is secreted by the secretory coil of the sweat gland. As the precursor fluid flows through the duct, much of the sodium chloride is reabsorbed in excess of water, resulting in sweat that is hyposmotic. The absorption of NaC1 by the duct is due to active transport of the Na+ by a sodium–potassium (Na+–K+)-sensitive adenotriphosphatase (ATPase). Na+ diffuses passively from the lumen to the cell interior and is then actively pumped out from the cell to the interstitium at the peritubular cell membrane in exchange for K+, with Cl− passively following Na+ (Gordon and Cage, 1966). The composition of horse sweat during exercise, heat stress, and epinephrine infusion has been measured by a number of investigators. The concentration of electrolytes reported in these studies is presented in Table 8-1. A number of methods of sweat collection have been utilized in these studies, including directly scraping sweat off the horse, collecting the drops that run off the horse, and collecting the sweat onto absorbent pads. Residual electrolytes from previous sweating and artificial elevation due to evaporation also will alter the sweat composition. These methodologic problems may explain the significantly higher electrolyte concentration reported by the early investigators when compared with later studies (McCutcheon and Geor, 1998; Smith, 1890). Equine sweat, unlike that of humans, is hypertonic relative to plasma, with a Na+ concentration similar or slightly higher than plasma, Cl– significantly higher, and K+ 10 to 20 times greater than serum concentrations. High-intensity exercise in horses produces more dilute sweat than low-intensity prolonged exercise, a process that may be caused by increased epinephrine concentrations that result during high-intensity exercise. Epinephrine infusion results in production of more dilute sweat than that occurring during exercise. Epinephrine concentrations are elevated during exercise in horses, and sweat in this species is produced in response to both sympathetic nervous activity and circulating epinephrine (McCutcheon and Geor, 1998). Sweat produced by the horse has an unusually high concentration of a protein relative to many other species. This protein is referred to as latherin and is responsible for producing the lather seen on horses after exercise. Latherin has a surfactant-like action, promoting spreading and evaporation of sweat and possibly aiding evaporation and cooling (Eckersall et al., 1982). The primary sudomotor mechanism in humans is cholinergic, whereas in the majority of other species this mechanism is essentially adrenergic-sympathetic. Sweating appears to be under sympathetic nervous control in the cow, sheep, goat, pig, donkey, and horse. This innervation involves α-receptors in the cow, sheep, and goat, α- and β-receptors in the dog, and β-receptors in the horse (Evans, 1955). The exact action of the nervous system on sweat glands has not been elucidated. The final stimulus to the glands must be humoral, either bloodborne or released from adjacent nerve endings. For example, sweat glands in humans and horses and the footpads of dogs and cats have a nerve supply closely associated with them. However, in the majority of other species, there must be a nonneural peripheral component in the sudomotor control mechanism. The sweat glands of humans, dogs, and horses respond to adrenergic and cholinergic drugs, whereas those of sheep and goats, donkeys, and pigs respond to adrenergic but not cholinergic substances (Snow, 1977). Experimentally, sweating in horses can be stimulated by intravenous and local injection of epinephrine. However, norepinephrine, the usual adrenergic postganglionic neurotransmitter, results in a minimal sweating response. Certainly, during exercise, the circulating epinephrine concentration is sufficient to cause sweating (Snow, 1977). The principal means for the role of the cardiovascular system as a thermoregulatory effector include (1) increasing the cardiac output (Q°) and (2) redistributing the cardiac output, particularly blood flow to the skin (Rowell, 1986). On exposure to heat, there is a resultant increase in blood flow to skin, inducing conduction of heat to the surface of the body and, thus, increasing skin temperature and facilitating convective heat loss from the body to the environment. In more severe heat stress, blood flow to skin also provides the latent heat for vaporization of sweat and supplies fluid for sweat production. In cold environments, reduced blood flow to skin decreases skin temperature, thereby limiting heat loss from the body (Rowell, 1986). The anatomic arrangement of the skin vasculature is designed to facilitate heat transfer. Three plexuses of vessels are present in skin such that a large volume of blood can be redistributed to skin to maximize heat loss. Specialized vessels, arteriovenous anastamoses (AVAs), are present in skin and contribute to this process. AVAs are short vessels that connect arteries and veins, and opening of these vessels results in bypass of capillary beds, allowing a greatly increased blood flow through skin. Control of skin blood flow is primarily mediated by the sympathetic nervous system. Response to heat involves vasodilation of arterioles and AVAs, whereas vasoconstriction in response to cold involves both arterioles and veins (Rowell, 1986). In response to heating, cardiac output (Q°) rises to maintain central blood pressure in the face of increased skin blood flow. In humans, cardiac output commonly increases 50% to 70% in response to heat stress and may more than double if the increase in core temperature exceeds 2°C. The increase in sympathetic nervous activity associated with heat stress results in a rise in heart rate and an increase in myocardial contractile force and stroke volume (Rowell, 1986). The alteration in Q° in the horse during heat stress is not well understood. Redistribution of Q° will differ among species, depending on the relative importance of the two major heat loss mechanisms: sweating and panting. In panting animals such as sheep and dogs, heat stress causes major increases in blood flow to respiratory muscles and the nasobuccal regions. However, in humans and horses, which rely on sweating for heat loss, there is an increased blood flow to the skin (Hales, 1973; Hodgson et al, 1993; Rowell, 1986). Redistribution of Q° in response to heat stress has been studied in ponies. Given the similarities between the thermoregulatory systems of horses and humans, similar responses to heat stress appear to occur. Exact measurements of redistribution of the Q° are not possible in humans. However, all available estimates indicate that the entire increase in Q° that occurs in heat stress is directed to the skin. In all the major organs in which flow has been measured (splanchnic, renal, and skeletal muscle), decreases in blood flow occur. The decrease in renal and splanchnic blood flows are in the order of 25% to 40% during significant heat stress. This matches the situation that occurs in ponies with the degree of reduction in cardiac output to the viscera directly relevant to the degree of heat stress, exercise intensity, and duration of exercise (McConaghy et al., 1996). In the 1930s, Nielsen demonstrated that a stable elevation of internal body temperature occurs during exercise in humans with this change proportional to the intensity of exercise and relatively independent of environmental changes. In 1966, Saltin and Hermansen further investigated this relationship in humans and discovered that body temperature elevation during exercise is related to the relative work load rather than the absolute work load. A similar relationship is reported to exist in horses. In 1949, Robinson first reported the linear relationship between sweating rate and internal temperature in humans and observed that the elevated body core temperature during exercise triggers a heat-dissipation response that is related to the magnitude of the elevation. These observations have been instrumental in the attempt to define temperature regulation during exercise (Nielsen, 1939; Saltin and Hermansen, 1966). Under normal circumstances, during exercise, a metabolic heat load is produced that is proportional to the intensity of the exercise bout. This heat load is transferred from the working muscles to the body core, resulting in elevation of core temperature. Sweating is initiated at a certain core temperature, dictated also by skin temperature, and sweating continues at a rate proportional to the increase in core temperature. At some point, determined by environmental conditions, the evaporative rate will match the rate of energy production. At this point, heat production will equal heat loss, and the body temperature will become stable and constant at a higher than resting core temperature (Rowell, 1986). Heat transfer in the horse during exercise is depicted in Figure 8-1. At the onset of exercise, the rate of heat production in muscle greatly exceeds the rate of heat dissipation, resulting in a rapid elevation of muscle temperature. Muscle temperature can increase at a rate of 1°C per minute at the beginning of strenuous exercise, and muscle temperatures of 45°C have been reported in exercising horses. Heat flows down the temperature gradient from the muscle to the surrounding tissue primarily via convection. An additional small amount of heat is transferred by direct conduction. The convective transfer occurs as a result of blood flow through the muscle. Muscle blood flow is greatly increased during exercise, which both increases oxygen supply and enhances removal of metabolic wastes and heat. Increases in blood flow to the working muscles of the pony of more than 10-fold from resting levels have been recorded (Hodgson et al., 1993; McConaghy, 1996; McConaghy et al., 1996). During the early minutes of exercise, core blood is heated with heat storage exceeding heat dissipation, and core temperature rises in proportion to the exercise intensity. The rise in core temperature during exercise has a number of advantages. It allows storage of heat, which reduces the amount of heat that must be dissipated. In addition, a moderate elevation of muscle temperature results in an improvement of muscular performance, facilitates oxygen release from the RBCs, and augments an increase in maximal heart rate. Metabolic reactions are accelerated, and enzyme activity is enhanced by moderate increases in temperature; thus, energy production is faster when core temperature is elevated (Åstrand and Rodahl, 1979). The core temperature rises slowly when compared with the temperature of muscle and blood in the central circulating pool, reflecting the large amount of energy that can be stored within the entire body mass. As the rate of heat dissipation rises to balance the rate of heat production, the core temperature reaches a plateau and remains relatively stable for the duration of exercise. The rise in the core temperature stimulates centrally located thermoreceptors, causing an increase in blood flow to skin and the initiation of sweating. The increase in skin blood flow transfers heat to skin for dissipation. The net transfer of heat from muscles to skin for dissipation depends on the core–to–skin temperature gradient. Skin temperature is initially lower than the core temperature. At the onset of exercise, skin temperature falls slightly due to increased convection resulting from the motion of the subject and then gradually rises. When skin temperature is already high, such as occurs in a warm environment or due to radiant gain from the sun, heat transfer to the skin will be compromised (Rowell, 1986). Heat is transferred from skin to the environment by convection, radiation, sweat evaporation, and respiratory losses. Environmental conditions, mainly temperature, govern which of these modes is most effective. Loss of heat by convection and radiation depends on a temperature difference between skin and the environment. When environmental temperature is low (∼10°C), the mean skin temperature will be approximately 25°C to 28°C, resulting in a temperature difference of 15°C to 18°C. Under these conditions, convection and radiation alone would be sufficient to dissipate the entire heat load imposed by mild-to-moderate exercise. As the ambient temperature increases, the skin–environment temperature gradient falls, becoming negligible at about 36°C and actually reversing at higher environmental temperatures. Thus, heat loss via convection and radiation becomes ineffective, and the body must rely on sweat evaporation for heat loss (Åstrand and Rodahl, 1979). In moderate ambient temperatures (say, 25°C), approximately 50% of the metabolic heat load is dissipated by radiation and convection and the other 50% by evaporation. However, when skin temperature and ambient temperature are equal, evaporative cooling becomes the only avenue for dissipation of heat. The rate of heat loss by evaporation of sweat depends mainly on the water vapor pressure gradient between skin and the environment and the fraction of the body surface area that is covered with sweat. High environmental humidity will decrease the water vapor pressure gradient and limit the ability of the body to lose heat via sweating. The environmental conditions of high ambient temperature and humidity present a serious threat to the body’s mechanisms of heat loss and can result in dangerous elevations of body temperature if exercise continues. This was one of the challenges faced when conducting the equestrian events for the Olympic Games in Atlanta (Adams et al., 1975; Jeffcott and Kohn, 1999; Rowell, 1986). where Horses are capable of very high work intensities, and the rate of heat production may exceed basal levels by 40- to 60-fold during racing speeds. The basal metabolic rate of a resting horse has been recorded at 2.2 to 4.2 mL/kg/min. This value is similar to that calculated from the metabolic body size (a function of the body weight to the 3/4 power, 3 kcal/kg3/4/hr). The metabolic body size of a 450-kg horse is 97.7 kg, the basal metabolic rate is 285 kcal/hr, equivalent to an oxygen uptake of 58.6 L/hr or 2.2 mL/kg/min. A Thoroughbred racehorse exercising at race speeds runs at approximately 16 to 17 meters per second (m/s), an intensity requiring its maximal oxygen uptake (85 to 90 L O2/min). This exercise level would be associated with a heat production of 450 kcal/min (90 L/min × 5 kcal/L). If this metabolic heat load was not dissipated, an elevation of body temperature of 1°C for every minute of exercise could occur (i.e., 60°C/hr), assuming the heat capacity of the horse is the same as that of humans (0.83 kcal/kg/°C) (Hodgson et al., 1993). Despite the lower rate of heat production during endurance exercise, heat dissipation is more important than during racing because of the prolonged duration of the exercise. A Thoroughbred racehorse typically races at maximal speed for 1 to 3 minutes. This would he associated with heat production of 1350 to 2250 kcal (450 kcal/min). The body is able to store a large amount of heat (0.83 kcal/kg/°C), 415 kcal/min for a 500-kg horse. If all the heat produced during a race was stored, the body temperature would rise 3.25°C to 5.42°C. Measurements of exact heat production during this type of exercise have been made, but estimates using treadmill exercise provide useful insights. At the trot and canter, heat production was 78 and 131 kcal/min, respectively. These levels of heat production would result in body temperature elevations of 0.13°C/min at the trot and 0.23°C/min at the canter. Recorded rectal temperature rises of only 0.02°C/min and 0.035°C/min occurred, indicating storage of approximately 15% of the heat produced (Hodgson et al., 1993). Heat that is stored during exercise is dissipated after cessation of exercise. The core temperature continues to rise within the first few minutes of recovery from maximal exercise as heat is redistributed from muscles.
Thermoregulation
Mechanisms of heat transfer
Radiation
Convection
Conduction
Evaporation
Regulation of internal body temperature
Physiologic thermoregulatory mechanisms for heat loss
Mechanisms of evaporative heat loss
Humans versus horses
Evaporative heat loss from the respiratory tract
Evaporative heat loss from sweating
Chemical composition of sweat: Humans
Formation of sweat in humans
Equine sweat composition
Innervation of the sweat glands
Circulatory adjustments for thermoregulation
Skin blood flow
Cardiac output
Redistribution of cardiac output
Thermoregulation during exercise
Energy exchanges during exercise
Estimations of heat production during exercise
O2 = oxygen consumption and k = amount of heat liberated per liter of oxygen consumed (k = 4.7 to 5.1 kcal, depending on the substrates used). Assuming a 20% metabolic efficiency, approximately 1 kcal/L of O2 is available for muscular work (Åstrand and Rodahl, 1979; Mitchell, 1977).
Stay updated, free articles. Join our Telegram channel
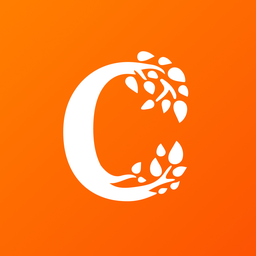
Full access? Get Clinical Tree
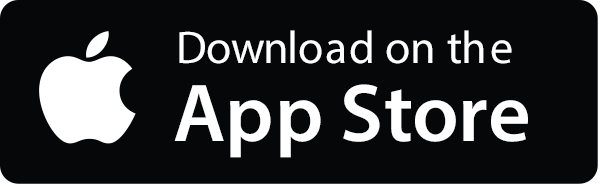
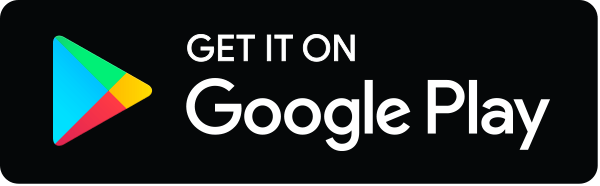