CHAPTER 3 The athletic capacity of horses results from physiologic properties (see also Chapters 1 and 2), attributed in particular to: 1. High maximal aerobic capacity ( 2. Large intramuscular stores of energy, particularly glycogen 3. High respiratory capacity of skeletal muscle 4. Splenic contraction, which results in the oxygen-carrying capacity of blood increasing by up to 50% soon after the onset of exercise 5. Highly efficient and adaptable gait(s) Vertebrate locomotion requires the controlled integration of numerous physiologic and metabolic pathways, which then impact on the musculoskeletal system, providing the organism with mobility. Perhaps the most important pathways are those concerned with the production of energy, for without energy muscles cannot contract and mobility is not achieved. Muscular movement requires the transformation of chemical energy stored in metabolic substrates or fuels to the kinetic energy of muscular contraction. All pathways integral to energy supply are concerned with the ultimate production of adenosine triphosphate (ATP), the final carrier of energy “packages” utilized by muscles for contraction. For muscles to contract, there is a coupling of thin actin and thick myosin filaments to form cross-bridges, and then these filaments slide relative to each other by a change in the orientation of the cross-bridges (Guyton, 1986; see Chapter 12). This process is repeated millions of times during muscular contraction. Energy is necessary for the change in orientation of the cross-bridges to occur, and the energy comes from the cleavage or hydrolysis of ATP at the head of each myosin filament. The cleavage of a high-energy phosphate bond from ATP is catalyzed by the enzyme myosin adenosine triphosphatase (ATPase) and produces adenosine diphosphate (ADP), free phosphate, a proton, and the release of energy (Figure 3-1). The energy released is utilized by the working muscle for contraction (Cain and Davies, 1962). In addition, ATP is the source of energy required to restore the contracted muscle to a relaxed or resting state via the distribution of calcium ions (Åstrand and Rodahl, 1986). Under normal conditions, there is a finite store of ATP within muscle, sufficient to maintain muscular activity for only a few seconds (Åstrand and Rodahl, 1986; Lindholm, 1979). Therefore, for continuous muscular exertion, it is necessary to resynthesize ATP, and this is done by the pathways of aerobic (oxidative) and anaerobic phosphorylation (Figure 3-2). Oxidative or aerobic phosphorylation production of ATP via aerobic pathways occurs within the inner membrane of mitochondria in a series of single oxidation reactions known as the electron transport or respiratory chain. Oxidation is the donation or loss of electrons (often in the form of hydrogen) from an atom or molecule, whereas reduction is the acceptance of electrons (hydrogen) by an atom or molecule. When electrons are donated, considerable chemical energy is liberated, and a portion of this energy is captured for the rephosphorylation of ADP to ATP, with the remainder being lost as heat energy (Guyton, 1986). Nicotinamide adenine dinucleotide (NAD) and flavin adenine dinucleotide (FAD) act as hydrogen carriers (acceptors) during glycolysis, beta (β)–oxidation, and the tricarboxylic acid (TCA) cycle and, therefore, are reduced to NADH and FADH2. These coenzymes are essential for aerobic and anaerobic phosphorylation, but their concentrations within the muscle are low. Therefore, NADH and FADH2 must be reoxidized to NAD+ and FAD via the electron transport chain. Specific mitochondrial enzymes incorporated in the electron transport chain catalyze the oxidation through a process of dehydrogenation. The donated hydrogen atoms provide the electrons that are transported from one enzyme complex to another by electron carriers, for example, cytochrome c. The importance of oxygen (O2) in this whole process is that it acts as the final hydrogen acceptor to form water. The energy released by the step-by-step transfer of electrons from NADH or FADH2 to O2 via the electron carriers is used to pump protons from the inner matrix of the mitochondrion into the outer chamber between the inner and outer mitochondrial membranes. This creates a strong transmembrane electric potential. Energy for the phosphorylation of ADP to ATP is obtained as the protons flow back through an inner membrane enzyme complex called ATP synthetase (Guyton, 1986; Stryer, 1988). The two major electron donor substrates for aerobic phosphorylation are carbohydrates (CHO) and fatty acids. Glucose is the main CHO, and if it is not used for immediate energy production, it is stored as glycogen, mostly in skeletal muscle and to a lesser extent in the liver (Hodgson et al., 1983, 1984; Lindholm, 1979). Adipose tissue constitutes the largest store of fatty acids (FA) (Robb et al., 1972; Stryer, 1988). Adipocytes store fat within their cytoplasm as triglycerides. Triglyceride storage also occurs to a much lesser extent in muscle (Lindholm, 1979). The importance of CHO as a substrate for energy production increases as exercise intensity increases (Hodgson et al., 1985; Lawrence, 1990). Glucose diffuses into the muscle cell cytoplasm from the circulation and is phosphorylated to glucose-6-phosphate (G-6-P) in a reaction catalyzed by the enzyme hexokinase (HK) and requiring one ATP molecule. G-6-P is then transferred into the glycolytic pathway for immediate energy production or reversibly converted to glucose-1-phosphate (G-1-P) and then glycogen for storage. Glycogen stores provide most of the glucose required for energy production during exercise. In the glycolytic pathway, G-6-P is phosphorylated to fructose-6-phosphate (F-6-P), which is then phosphorylated to fructose-1,6-bisphosphate (F-1,6-BP) in a reaction catalyzed by phosphofructokinase (PFK) and at the expense of one ATP molecule. F-1,6-BP is subsequently split into the triose phosphate isomers, glyceraldehyde-3-phosphate (Gl-3-P) and dihydroxyacetone-phosphate (DiH-P). DiH-P is readily converted into Gl-3-P by triose phosphate isomerase (TPI). Therefore, one molecule of glucose or glycogen gives two molecules of Gl-3-P. Gl-3-P then proceeds through a series of reactions, the end result being the production of pyruvate, ATP, NADH, water, and hydrogen ions. The net reaction for the glycolytic pathway utilizing glucose is: Under aerobic conditions, the hydrogen atoms are transferred to the electron transport chain, and pyruvate is transported into the mitochondrial matrix as a substrate for acetyl coenzyme A (acetyl CoA). Acetyl CoA then enters the TCA cycle by combining with oxaloacetate to form citrate. The normal function of the TCA cycle requires three NAD+ and one FAD to accept hydrogen atoms during the oxidative conversion of citrate back to oxaloacetate. When O2 is available, the NADH and FADH2 are then reoxidized back to NAD+ and FAD in the electron transport chain, thus replenishing the adenine dinucleotide stores and producing ATP (see Figure 3-2). The complete aerobic utilization of one mole of glucose generates 36 to 38 ATP. When O2 is not available, pyruvate is converted to lactate as described later. Following lipolysis, nonesterified fatty acids (NEFAs) are released into the circulation and are subsequently available as hydrogen donors for energy production in skeletal muscle. NEFAs likely diffuse into muscle cells down a concentration gradient as well as being actively transported across the cell membrane. At the cytoplasmic surface of the outer mitochondrial membrane, the NEFAs are esterified (activated) enzymatically forming long-chain acyl CoA molecules. The acyl CoA molecules are then linked to carnitine and shuttled across to the matrix side of the inner mitochondrial membrane. In the mitochondria, the acyl CoA molecules undergo a series of four reactions known as β-oxidation. With each cycle of β-oxidation two-carbon (C2) units are sequentially removed from the acyl CoA molecule and acetyl CoA, NADH, and FADH2 are produced (see Figure 3-2). NADH and FADH2 subsequently donate their electrons in the electron transport chain, generating ATP and being reoxidized to NAD+ and FAD in the process. Acetyl CoA is utilized in the TCA cycle as previously described. The splitting of C2 units from the parent acyl CoA molecule is repeated until the whole chain has been cleaved into the acetyl CoA molecules. The number of carbon atoms in the parent FA chain will determine the net energy yield from β-oxidation. Complete oxidation of one palmitic acid molecule produces 129 molecules of ATP. The pathways of anaerobic phosphorylation occur solely in the muscle cell cytoplasm, with no reactions in the mitochondria as there are for aerobic phosphorylation. With the initiation of exercise, there is a lag period before oxidative energy production becomes an important source of ATP. During this time, rapid supplies of ATP must still be available if muscular contraction is to continue. Stores of ATP in skeletal muscle are limited (4–6 millimoles per kilogram [mmol/kg] wet muscle) and contribute little to the total energy supply (Lindholm and Piehl, 1974; McMiken, 1983). Until aerobic phosphorylation makes a substantial contribution to energy supply, rapid regeneration of ATP must occur in the absence of O2. The anaerobic phosphorylation of ADP is achieved by three pathways: (1) the phosphocreatine reaction, (2) the myokinase reaction, and (3) anaerobic glycolysis. The former two pathways may be described as anaerobic alactic reactions because no lactate is produced as it is in the latter process (Clayton, 1991). In the gluteus medius muscle of Standardbreds, the size of the CP pool is estimated to be 15 to 20 mmol/kg wet muscle (Lindholm and Piehl, 1974; Lindholm, 1979). This source of ATP replenishment would support maximum intensity exercise for no more than a few seconds (Åstrand and Rodahl, 1986; Clayton, 1991). At rest, this reaction proceeds at an approximately equal rate in both directions with little net ATP being produced. In working muscle, AMP-deaminase reduces AMP concentration by converting it to ionosine monophosphate (IMP) and ammonia. This provides the driving force for the myokinase reaction toward the production of ATP (McMiken, 1983). Again, this pathway only has the capabilities of providing small amounts of ATP. The anaerobic production of two molecules of pyruvate from one molecule of glucose or glycogen is identical to that described for aerobic glycolysis. In the absence of available O2, pyruvate accepts hydrogen atoms from NADH and is converted to lactate, rather than being converted to acetyl CoA and entering the TCA cycle. The reaction is catalyzed by lactate dehydrogenase, and the regeneration of NAD+ during the reduction of pyruvate to lactate sustains glycolysis under anaerobic conditions (see Figure 3-2). The net result of anaerobic glycolysis is the production of three molecules of ATP from one molecule of glycogen or two molecules of ATP from one molecule of glucose. This form of energy production is relatively rapid compared with aerobic glycolysis but yields a significantly lower amount of ATP, and substrates are limited. At the initiation of exercise, the immediate source of energy is locally available ATP. As previously noted, this supply of ATP is limited and rapidly depleted; therefore, for exercise to continue, ATP must be replenished by other processes. Creatine phosphate and the phosphocreatine pathway provide the next rapidly available ATP source; however, this energy supply is also of limited capacity (McMiken, 1983). The myokinase reaction provides a further means of regenerating ATP, but it is restricted to certain muscle fiber types and is used in anaerobic exercise only when these fibers are recruited (Meyer et al., 1980). The myokinase reaction is believed to have a minor role in energy production overall. The glycolytic pathway with the production of pyruvate and lactate provides the main ongoing ATP source and reaches peak metabolism within about 30 seconds of the onset of exercise. The delay in maximal glycolytic output is possibly caused by the multiple and complex reactions required (McMiken, 1983). The large stores of glycogen in equine muscle (Hodgson et al., 1984; Lindholm, 1979; Lindholm and Piehl, 1974; Lindholm et al., 1974; Nimmo and Snow, 1983) allow this pathway to provide an early consistent source of energy, but still there is a finite limit to this substrate. Aerobic mechanisms for ATP replenishment represent the most efficient means of substrate production. However, it is the slowest pathway to respond to exercise demands, owing to the cardiovascular lag in supplying O2 to the cells and the intricacy of the reactions. Oxidative processes are in full production within 1 minute after the onset of exercise, and then muscular energy is more likely to be dependent on the rate of O2 transport to the cells rather than substrate availability (McMiken, 1983). In fit racehorses, the time to peak energy production via oxidative processes can be as short as 20 seconds, especially following warm-up (Tyler et al., 1996). At rest and during low-intensity exercise (walking and trotting), aerobic pathways provide most energy requirements after the initial lag period. At this exercise intensity, the ratio of ATP to ADP will be high; PFK will, therefore, be inhibited and β-oxidation of fatty acids will provide the main method for ATP regeneration (Hodgson et al., 1985; Lawrence, 1990). Such is the case during endurance rides, in which case, it is well recorded that blood concentrations of nonesterified fatty acids (NEFA) increase and the glycogen utilization rate is low (Hodgson et al., 1983; Hodgson et al., 1985; Lucke and Hall, 1980; Snow et al., 1982) (Table 3-1 and Table 3-2). TABLE 3–1 Rates of Glycogen Utilization in Horses Performing Various Types of Athletic Activities *Millimoles glucose units per kilogram muscle per minute (dry weight). †Millimoles glucose units per kg muscle per min (wet weight). Approximate figures for dry weight values are presented in parentheses (Adapted from Hodgson, 1985.) TABLE 3–2 Mean Plasma Nonesterified Fatty Acid Concentration in Horses Performing Various Athletic Activities (Adapted from Hodgson, 1985.) As exercise intensity increases, ADP accumulates, and this stimulates anaerobic glycolytic energy production and a dramatic increase in the use of CHO substrates (see Table 3-1). Galloping and bursts of intense exercise such as during polo games and jumping rely heavily on anaerobic energy supply. The self-limiting nature of anaerobic power output (substrate exhaustion) means the horse can only maintain maximal speed for about 600 to 800 m. After this distance, energy supply falls back to the slower aerobic pathways, necessitating a reduction in speed of exercise (Hodgson et al., 1985; McMiken, 1983). Scientific data on the relationship between nutrition and equine performance has occupied the attention of many researchers in recent years; however, it is difficult to design controlled experiments that only isolate nutritional influences on performance. Subtle, yet important effects of nutritional alterations may go undetected, partly because the power of statistical studies is limited by the small numbers of horses often used in experiments (Hintz, 1994). The relationship between energy and exercise is complex and inseparable. The amount of energy required depends on the type and duration of activity and the horse’s body weight. Maintenance digestible energy (DE) requirements are linearly related to body weight (Pagan and Hintz, 1986a). During submaximal exercise energy expenditure is exponentially related to speed and proportional to the body weight of the riderless horse or the combined weight of the horse plus rider (Pagan and Hintz, 1986b). The method used by Pagan and Hintz (1986b) for calculating energy requirements was based only on the amount of work performed and may not account for any follow-on demands for energy in recovery that the work bout stimulates (Lawrence, 1990). The stores of major fuels in the horse for muscular contraction are outlined in Table 3-3 as calculated by McMiken (1983). It is clear that “fast” energy stores (i.e., ATP, creatine phosphate, and glycogen) are limited despite the high capacity for glycogen storage in equine muscle. The primary dietary sources of energy stores for the horse are soluble and fiber derived CHOs and fats. Protein is considered to play a minor role as an energy source. Absorbed CHO is immediately available as an energy source in the form of blood glucose. Muscle and liver are the reservoirs where excess CHO is stored as glycogen. Numerous studies have documented the depletion of muscle glycogen stores that occurs with exercise in the horse. The rate and percentage of depletion that results is a function of the intensity and duration of exercise. Muscle glycogen utilization per minute is greatest at faster speeds over shorter distances (Hodgson et al., 1984; Nimmo and Snow, 1983) (see Table 3-1), but the total percentage of glycogen depletion increases with increasing duration of exercise (Hodgson et al., 1983; Snow et al., 1981; 1982). Liver glycogen stores are also depleted significantly during exercise (Lindholm et al., 1974; Lindholm, 1979). Assimilated fats are stored as triglycerides (uncharged esters of glycerol) in adipose tissue and muscle. Quantitatively, adipose tissue constitutes the largest energy store in the body (see Table 3-3). Triglyceride concentrations in muscle are considerably less than in fat (Lindholm, 1979). The triglycerides are highly concentrated stores of energy because they are reduced and anhydrous (Stryer, 1988). The initial event in the utilization of triglycerides as an energy substrate is their hydrolysis by lipases to glycerol and free fatty acids (FFA). Lipolysis is stimulated by epinephrine, norepinephrine, glucagon, and adrenocorticotrophic hormone and inhibited by insulin. Glycerol is converted in a number of steps to Gl-3-P, which is an intermediate in both the glycolytic and gluconeogenic pathways. The FFAs undergo β-oxidation and enter the TCA cycle as previously described. Oleic, palmitic, and linoleic acids represent the major FAs in the horse (Robb et al., 1972). Fat has been shown to be the major energy substrate during low-intensity exercise. This is best evidenced by a decrease in the respiratory exchange ratio (R) (McMiken, 1983; Pagan et al., 1987; Rose et al., 1991) and an increase in plasma NEFA concentrations (Essen-Gustavsson et al., 1991; Lindholm, 1979; Rose et al., 1980) that occurs with prolonged submaximal exercise. R is calculated by dividing the volume of carbon dioxide (CO2) expired by the volume of O2 consumed during exercise. R values around 0.7 indicate fat utilization, whereas for CHO utilization, the value is 1.0. Values within this range reflect various mixtures of FA and CHO metabolism. When anaerobic metabolism predominates, R values will exceed 1.0 because lactate production is high, thereby adding to the CO2 load to be eliminated. Digested protein is absorbed from the small intestine as amino acids and small peptides. When amino acids are available in excess of the animal’s requirements, they may be broken down to provide energy. Degradation by deamination or transamination reactions occurs mostly in the liver, with the final product being acetyl CoA for utilization in the TCA cycle. Leucine, a branched-chain amino acid, may undergo oxidation directly in muscle (Lawrence, 1990). The contribution of amino acids to energy production during exercise is minor compared with that of CHO and FA (Åstrand and Rodahl, 1986), perhaps in the range of 1% to 15% (Lawrence, 1990). High-protein diets (up to 16%) were once thought necessary to sustain the performance of mature equine athletes, but now it is considered that approximately 10% protein in the diet is adequate (see Chapter 4). Many published reports have described the effect that altering components of the normal diet has on substrate utilization and performance in the horse. The consumption of large amounts of digestible CHO within a few hours of strenuous activity may depress the performance of that exercise (Åstrand and Rodahl, 1986). This is possible because insulin-stimulated uptake of blood glucose results in hypoglycemia, a greater dependence on muscle glycogen, and, therefore, earlier onset of fatigue. Free FA mobilization is also inhibited by insulin (Frape, 1988). The effects of consumption of CHO or fat before or during exercise on metabolism are summarized in Table 3-4. However, a lack of available CHO during submaximal exercise can also limit performance; there is strong evidence supporting the use of high CHO diets in humans for endurance exercise. In humans, muscle glycogen loading was achieved by performing intense exercise and then consuming a CHO-rich diet (Lindholm, 1979). Current practice is to combine a program of decreased activity with increased CHO consumption a few days before competition to achieve a glycogen load. Glycogen loading in horses has been accomplished, but no obvious improvement in work performance has been demonstrated (Frape, 1988; Lawrence, 1990; Snow, 1994; Topliff et al., 1983; 1985). Intravenous, but not oral, glucose supplementation has increased glycogen repletion rates after exercise (Davie et al., 1994; 1995). Although glycogen loading is not recommended in the horse, adequate CHO intake must still be ensured (Hintz, 1994). A low CHO diet and regular exercise lead to glycogen depletion and decreased performance in horses (Topliff et al., 1983; 1985). In a study in which fit Standardbreds were exercised strenuously for 3 consecutive days to achieve a 55% depletion of the muscle glycogen store, anaerobic, but not aerobic, capacity was seen to be impaired (Lacombe et al., 1999). However, the association between glycogen depletion and impaired anaerobic metabolism is not conclusive as confounding effects of other exercise-induced changes on performance could not be eliminated (Lacombe et al., 1999). When muscle glycogen was depleted by 22%, there was no significant effect on the performance of Thoroughbreds exercising at high intensities (Davie et al., 1996). A CHO supplement taken an hour or two before exercise does not seem to benefit endurance performance, but intake of glucose during exercise may supplement waning plasma concentrations and delay the onset of fatigue (Lawrence, 1990). The beneficial effects of feeding high-fat diets to horses on substrate utilization and performance remain controversial, although benefits for horses with problems such as recurrent tying up and gastric ulceration are unequivocal. Differences in the condition of the horses, type of exercise, the length of the adaptation period to the diets, the type of fat used as the supplement, and the level of fat supplemented, particularly in relation to CHO, make comparing the published results difficult. Many variations in study designs influence the results. Feeding an increased level of fat is suggested to cause metabolic adaptations that permit horses to preferentially utilize fat and spare glycogen during exercise, but the evidence to support such a proposal is inconclusive (Hintz, 1994; Lawrence, 1990; Snow, 1994). Interest in amino acid requirements of the performance horse is growing, and there is a suggestion that improvements in oxidative capacity can be brought about by certain amino acid supplements (Hintz, 1994; Lawrence, 1990). Higher-than-necessary protein diets are often fed to performance horses, but studies to demonstrate that this practice enhances exercise capabilities are lacking (Lawrence, 1990). In the case of CHO-rich and fat-rich diets, plasma concentrations of glucose, ammonia, lactate, alanine, and the muscle concentrations of G-6-P and lactate were higher at the end of exercise, compared with normal diets (Essén-Gustavsson et al., 1991). Higher pre-exercise muscle glycogen concentrations and FFA concentrations were present when horses were fed a CHO-rich diet compared with the fat-rich and normal-diet fed periods. No significant difference in performance during trotting at submaximal intensity on a horizontal treadmill was detected between the three diets (Essén-Gustavsson et al., 1991) with the average time to fatigue being 51 to 56 minutes. Whether or not the diets would alter performance in shorter or longer exercise periods remains unanswered. The effects on protein metabolism need to be further investigated as both the CHO-rich and the fat-rich diets, compared with normal diet, were associated with significant increases in branched-chain amino acids in plasma during and at the end of exercise (Essén-Gustavsson et al., 1991). The resting plasma concentration of the branched-chain amino acids was increased by 26% with the fat-rich diet but only by 8% with the CHO-rich diet. A 9% (control) or 18.5% (high) crude-protein diet had no effect on hepatic or muscular glycogen utilization and did not affect exercise performance in Quarterhorses exercising at submaximal intensities (Miller-Graber et al., 1991). Performance of Arabian endurance horses was not augmented by excessive protein in the diet (Hintz, 1983). In contrast, Standardbreds fed a high-protein diet (20%) or high-fat diet (15% soybean oil) showed greater muscle and liver glycogen utilization during prolonged exercise than when fed a control diet of 12% crude protein (Pagan et al., 1987). During higher-intensity, shorter-duration exercise, glycogen utilization was less when horses were fed high-protein or high-fat diets. The timing of feeding and what to feed before exercise have considerable influence on the metabolic and physiologic responses to exercise (Harris and Graham-Thiers, 1999; Lawrence et al., 1995). In one study, it was concluded that feeding only hay shortly before exercise would not adversely affect performance but feeding grain would and that grain therefore should be withheld (Pagan and Harris, 1999). Of course, many other nutritional components not discussed in this chapter may play roles in equine performance. These include water, electrolytes, acid–base balance, minerals, and vitamins. For more information see Chapter 4.
Energetic considerations of exercise
O2max)
Production of energy
Aerobic glycolysis
Fatty acid utilization
Anaerobic phosphorylation
Phosphocreatine reaction
Myokinase reaction
Anaerobic glycolysis
Energy pathway contributions in the exercising horse
Energy substrates
Carbohydrates
Fat
Protein
Effects of dietary alterations on energy substrate utilization
Stay updated, free articles. Join our Telegram channel
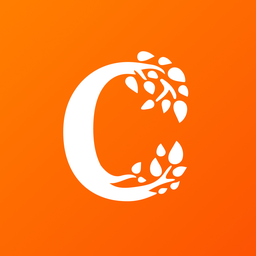
Full access? Get Clinical Tree
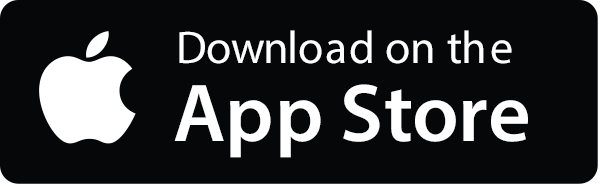
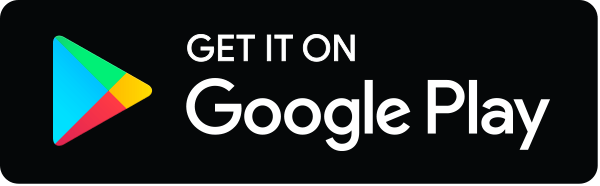