Fig. 1.
Illustration of the location, connections and composition of the PPTg. (a) Sagittal view on a rat brain section (taken from the atlas of Paxinos and Watson (94)). Schematic representation of the principal afferent and efferent connections of the PPTg with forebrain and brainstem nuclei. Black arrows represent cholinergic connections, dotted arrows GABAergic and dashed arrows glutamatergic connections. Grey arrows represent unclassified connections. (b) Schematic graded distributions of glutamatergic, cholinergic and GABAergic neurons within the PPTg. The gradient represents the rostro-caudal distribution of each neuron type based on the cell identification of Wang and Morales (2) and Mena-Segovia et al. (95). GPe globus pallidus externa, GPi globus pallidus interna, LC locus coeruleus, LH lateral hypothalamus, LDTg laterodorsal tegmental nucleus, SC superior colliculus, SN substantia nigra, STn subthalamic nucleus, VTA ventral tegmental area.
2 How to Study the Pedunculopontine
In order to study the behavioural (and inferentially, psychological) functions of the PPTg, scientists have typically applied the classic methods of behavioural neuroscience to problems of movement disorder. For the most part, data have generalized across species, but this is not always the case. It is widely accepted that the structure and connectivity of the PPTg is similar across species, up to and including humans. Imaging of human brain has not often touched on the brainstem, but at least some studies have produced data that are entirely compatible with conclusions based on rodent experiments (47). What has been harder to accommodate with the rodent literature are studies of movement in primates.
Perhaps the most difficult aspect of dealing with the PPTg – alluded to already in the section on DBS – is direct intervention through stereotaxic surgery. In humans there is still uncertainty about the precise location of PPTg. In experimental animals, stereotaxic surgery presents a number of problems (1) if one wishes to lesion the whole structure, multiple small infusions of neurotoxin are required because PPTg is a relatively long thin structure; (2) exposure of the surface of the brain prior to penetration within a device exposes a large superficial blood vessel – the superior sagittal sinus – that can be damaged while drilling skull, or which can require moving aside in order to access brain below; (3) we have found that the introduction of excitotoxic agents into the PPTg in both hemispheres simultaneously is often fatal to rats and as such we do not use this approach. Rather, we make lesions in one hemisphere then return 7 days later to make lesions in the contralateral one.
Targeting the PPTg by stereotaxic surgery is a challenge. In rats, when the skull is in a level position during surgery, the PPTg sits right underneath the superior sagittal sinus. By elevating the incisor bar on the stereotaxic frame, problems with the sinus can be avoided, and the vessel not touched by inserted pipettes, cannulae or electrodes. The angle to move the incisor bar to is calculated by multiplying the distance between the interaural line and the back of the incisor bar by the sine of 8°29′ (0.147), as described by Whishaw et al. (48). The cholinergic neurons of the PPTg are intimately associated with those of the adjacent LDTg (much as the DA-containing neurons of SNc are continuous with those of the VTA). Stereotaxic access to the LDTg is even more difficult because the bulk of it is embedded within the central gray, close to the cerebral aqueduct and access to it is also obstructed by superficial blood vessels. By raising the incisor bar as described above helps avoid the vessels; refining the stereotaxic coordinates by means of pilot studies, using fine tipped glass pipettes, as well as measuring the dorsoventral distance to the LDTg from dura rather than skull has helped our lab improve the accuracy and reliability of infusions into the LDTg. Other labs have used angled approaches to the LDTg, though this can be difficult because it adds an extra potential for stereotaxic error.
Stereotaxic surgery of human PPTg has proven to be even more difficult. One major point of discussion, as mentioned above, is the correct localization of the nucleus. Neurosurgeons describe the PPTg as “unfamiliar territory” pointing out the difficulty of defining the borders of the PPTg correctly because of its small size and reticular nature. Individual differences between subjects are also more of an issue than one faces working with laboratory animals of a standard age and size. Preoperative neuroimaging should be of benefit here, but the fact that the PPTg is not clearly visible in T1-weighted MR images makes this difficult (49, 50). Common approaches to refining localization during functional neurosurgery are microelectrode recording and measuring local field potentials, which remain only an approximation because the specific physiological characteristics of human PPTg neurons are as yet undefined (49). However, by recording cell activity and plotting the corresponding neurons in the coordinate system of a human brainstem atlas, these approaches can provide useful information for electrode implantation (51). Post-operative structural magnetic resonance imaging should be obtained, both in order to match these with intra-operational microelectrode recording and to create evidence for functional characteristics of PPTg neurons of PD patients (23). Localization by direct proton density MR imaging is also a technique described as being useful during surgery in order to address these problems (49).
2.1 Lesions
2.1.1 Excitotoxic Lesions
Discrete lesions of the whole PPTg in our lab are successfully made by the neurotoxin ibotenic acid. Infusions of ibotenate are made up as 0.12 M solution in phosphate buffer with a final pH adjusted to pH 7.0 using 2 M NaOH. This technique spares fibres of passage but it damages all neuronal types (52). In order to avoid creating lesions of adjacent structures but still targeting the complete PPTg, multiple placements of low volumes are necessary. When delivered in a volume of 200 nl in two injection sites per hemisphere the whole structure can be affected. The toxin is infused using a 0.5–1 μl syringe or by pressure ejection from a drawn glass micropipette (tip diameter, 30–40 μm) for better control and left in situ for 5 min after infusion. Bilateral lesions are performed in two separate unilateral surgeries separated by 7 days, as experience immediately proved that bilateral ibotenate infusions made in this region in only one surgery resulted in high mortality rates. During the immediate post-operative recovery period convulsive activity and barrel-rolling are to be expected with any excitotoxin. Rats need to be monitored closely during this period until this activity disappears. It is rarely acknowledged, but important to note that different excitotoxins have different diffusion characteristics which need to be examined in pilot experiments; see (39) for an elaboration of this point.
What are the behavioural consequences of such lesions? As outlined above, excitotoxic lesions of the rodent PPTg do not have an effect on either spontaneous or amphetamine-induced locomotion (induced by either systemic administration or microinjection directly into the nucleus accumbens) (40). Other basic behavioural patterns such as feeding, drinking or grooming are not affected either (53). It can, however, be shown that the PPTg is involved in complex associative processing. Lesions of the PPTg affect responding for conditioned reinforcement. PPTg lesioned rats show an increased rate of response on non-reinforced levers, as opposed to control rats that learn to discriminate between the levers and focus almost exclusively on the reinforced lever. This indiscriminate lever pressing reflects a disruption in the process of reward-related behaviour but not motor deficit per se (40). The same inability to learn the association between a conditioned stimulus and a reward has been shown in experiments using different experimental paradigms (54) after bilateral excitotoxic lesions of the whole PPTg. Complex cognitive processing as required to complete the delayed spatial win-shift radial maze task has also shown to be affected by whole bilateral PPTg lesions in the absence of any locomotor deficits (55), an effect that cannot be attributed to disrupted motivation (55, 56). Here it made no difference whether or not the rats received the lesions before or after being trained on the task. They made significantly more errors than sham-operated rats and showed therefore impairment in making the appropriate choice of the arm to enter in the maze (55).
Given the strong connections to midbrain DA neurons and their importance for establishing associations between action and reinforcement (57), an involvement of the PPTg in drug reward was expected. Responding for intravenous administration of amphetamine (58) and heroin (59) is affected by lesions of the whole PPTg; self-administration of nicotine was investigated with separate lesions of the anterior PPTg (aPPTg) and posterior PPTg (pPPTg) because of the different relationships these have with midbrain DA neurons – pPPTg preferentially innervates the VTA and aPPTg the SNc. Posterior but not anterior lesions affected nicotine administration. On the other hand, lesions of aPPTg cause significantly reduced baseline levels of locomotor activity which is not observed after pPPTg lesions (60). In respect to drug self-administration studies, PPTg lesions appear to abolish the ability to learn a specific contingency between an action and its outcome, unless the association between action (lever pressing) and reward (outcome) has been made prior to the lesion. However, when the requirements for obtaining a reward change rats with PPTg lesions that previously performed well show impairments (58, 61). These data strongly support the contention that the PPTg is involved in learning about the selection of appropriate actions rather than movement per se.
2.1.2 Transient Lesions
Another method of studying the behavioural functions of the PPTg is inactivation of the structure by direct pharmacological manipulations rather than by permanently lesioning it. This can be achieved, for example, by infusion of the GABA agonist muscimol into the PPTg (62). For this purpose, guide cannulae are placed above the PPTg and by means of injectors, muscimol can be administered prior to the testing session. In our lab, a dose of 50 ng/hemisphere has shown to produce an effect in the PPTg, however other researchers observed effects only after higher doses (62). A much smaller amount is necessary to inactivate, for example, the VTA (63). The use of muscimol is especially interesting in the study of the PPTg’s role in learning because it allows re-tests of rats after inactivation, and the use of within subject controls. Furthermore, long interruptions between training and testing due to surgeries can be avoided.
In line with data obtained from ibotenic acid-lesioned rats, inactivation of the pPPTg by means of muscimol in our own lab (D. Wilson and D. MacLaren, unpublished data) caused rats to significantly overconsume 20% sucrose solution compared to rats with sham inactivation (Fig. 2). This validates that the technique has similar effects to ibotenic acid lesions and allows for further studies in behavioural paradigms suited to inactivations rather than lesions.
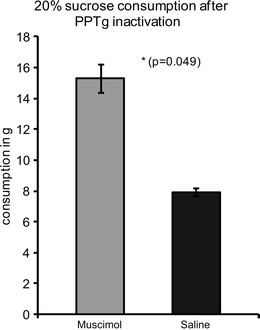
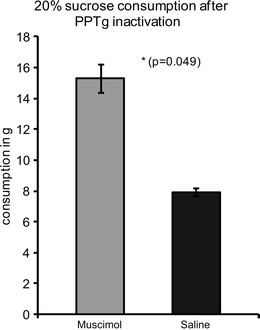
Fig. 2.
Mean (±SE) consumption of 20% sucrose solution (in grams solution) over 90 min following posterior PPTg inactivation by muscimol microinjection or sham inactivation by saline microinjection. (Muscimol: 0.05 μg/0.3 μl per hemisphere infused at 0.3 μl/min via 30-gauge stainless steel cannulae inserted via permanent indwelling guides. Sham inactivation involved an identical procedure but infusion of the vehicle solution [0.9% saline] only).
A change in firing pattern from tonic to burst firing in midbrain DA neurons is implicated in reward-related learning (64). Maskos (46) explains how the cholinergic neurons of the mesopontine tegmentum, including the PPTg, may be essential for this change: restoration of the high-affinity nicotinic–cholinergic receptor subunit β2 in the VTA restores burst firing, which is completely absent in the mouse knockout of the same receptor subunit (65). An inactivation study offers support for this hypothesis: inactivation of the PPTg by means of 1% lidocaine hydrochloride silences conditioned responses of midbrain DA neurons which were present before the inactivation (66). Using muscimol for temporary inactivations Corrigall et al. have shown that the inactivation of the whole PPTg lead to reduced nicotine self administration in rats which had learnt to lever press for nicotine prior to inactivation (67).
2.1.3 Lesions Selective for Cholinergic Neurons
Because the PPTg is a heterogeneous structure with different interdigitated neurons, isolating the functions of the different neuron types is of considerable interest. This cannot be achieved with neurotoxins such as ibotenic acid. The first neurotoxin that was thought to be selective for cholinergic neurons was ethylcholine mustard aziridinium ion (AF64A) which operates through choline transport mechanisms and causes a decrease in cellular choline acetyltransferase activity (68, 69). It has been used in the PPTg (70) but it has been shown that even low doses of AF64A produce a local non-specific lesion in the injection site (71). AF64A has not been a success in PPTg or elsewhere in brain.
The best-known fusion toxin selective for cholinergic neurons is 192 IgG saporin, which has been invaluable in study of basal forebrain cholinergic neurons. It is ineffective on PPTg neurons because they lack the necessary 192 IgG nerve growth factor receptor (72). However, the fact that only cholinergic neurons in the mesopontine – the Ch5 group in the PPTg and the Ch6 group in the LDTg – express the urotensin II receptor has allowed for the development of a selective fusion toxin (DTx/UII). The conjugation of the diphtheria toxin (DTx) catalytic portion and an active UII-targeting domain created a toxin that selectively binds to cholinergic PPTg neurons causing cell death over a period of 21 days (73). The only published report of behavioural effects of this toxin has to be interpreted with great caution as the behavioural effects in terms of changes in gait and posture are the result of the bilateral injection of DTx/UII in a concentration (20–30%) that causes non-selective lesions rather than selective cholinergic lesions, as the authors report themselves (74). In recent work in this lab (75), selective lesions of cholinergic neurons in the posterior PPTg made with DTx/UII that produced significant loss of cholinergic neurons (measured by choline acetyltransferase immunohistochemistry) but not non-cholinergic neurons (measured by NeuN immunohistochemistry) caused only minor behavioural effects. This raises intriguing questions about the substrate for the robust behavioural effects seen after excitotoxic lesions.
2.2 Stimulation
In the past, the stimulation of the PPTg has been achieved by electrical stimulation or neurochemical stimulation using the muscarinic cholinergic antagonist scopolamine or agonist carbachol, the GABA receptor antagonist bicuculline, N-methyl-d-aspartate (NMDA) or l-trans-pyrrolidine-2,4-dicarboxylic acid (PDC), which blocks l-glutamate uptake and presumably prolongs the activity of endogenous glutamate. Electrical and neurochemical stimulation of the PPTg has been shown to activate DA neurons in SNc and VTA (76, 77) and to increase burst firing without affecting population activity (78). Furthermore, PPTg stimulation causes changes to phasic DA release in the nucleus accumbens (79). In a more detailed analysis, stimulation of the LDTg showed that its excitatory input causes DA midbrain neurons to release DA in the ipsilateral striatum with a triphasic pattern of an initial rapid release, a short reduction in release and a sustained increase (80); it is thought likely that the same pattern follows PPTg stimulation.
In order to examine the influence of the PPTg on neuronal activity of the thalamus, Fos expression was measured after PDC infusion into the PPTg – increased expression of Fos was seen in the centrolateral, ventrolateral and reticular nuclei (81). This stimulation caused no change in locomotion of the rats, so the increase in thalamic activity can be attributed to the PPTg stimulation itself rather than being an artefact of the injection procedures; care needs always to be taken in measuring Fos activity in freely moving animals. The use of a glutamate reuptake blocker is particularly helpful: the PPTg has substantial glutamatergic inputs. Direct injection of exogenous glutamate has little effects because it is rapidly removed by high affinity uptake processes. Structural analogues of glutamate can be problematic also, because of their capacity to induce excitotoxic lesions; this indeed is what excitotoxins are. Blockade of reuptake effectively prolongs the activity of endogenous glutamate – levels of glutamate might therefore achieve levels that are at the maximum of what might normally be physiologically present, but there is the virtue of achieving stimulation targeted through normal synaptic processes.
2.3 Electrophysiological Recordings
Electrophysiological recordings of PPTg neuronal activity in experimental animals are not numerous – the difficulties of access to the PPTg are in part the cause of this – but they nevertheless allow examination of its physiological characteristics and are particularly interesting because they show a range of different activities in response to locomotion and voluntary movement. Garcia-Rill identified three types of neurons in the area of the PPTg that display rhythmic activity in decerebrate rats in response to locomotion (82). Non-bursting, possibly cholinergic Type II neurons relay feedback of sensory information from the spinal cord and provide the main inputs back into the thalamus and the SNc. They can be divided into ON and OFF cells. The ON cells show a tonic firing pattern during locomotion which ceases or decreases when locomotion stops. The OFF cells also exhibit a tonic firing pattern which increases before the cessation of locomotion and decreases when the locomotion frequency increases. Those neurons might be important for the maintenance of gait. Bursting non-cholinergic type I neurons show a bursting pattern of firing during locomotion which are primarily innervated by GABAergic GPi and possibly SNr which provide the main PPTg outputs to the spinal cord. These neurons might be important for the initiation of programmed movements, determining therefore the initiation and frequency of stepping (for review see (15)).
Electrophysiological recording studies show that the PPTg receives polymodal sensory information and is involved in reward-related learning. In a conditioned lever-pressing task, cats were trained to hold down a lever until an auditory signal indicated that releasing the lever would lead to a food reward. PPTg neurons responded very early to the stimulus when the conditioned movement had to be executed as quickly as possible, which could be interpreted as a signal triggering the previously learned action. PPTg neurons furthermore reacted with a sustained response when the cat was expecting the reward and when the food reward was given (83). This resembles DA activity in SNc and ventral striatum in relation to reward and expectation of reward (57). To investigate further the involvement of PPTg neurons in reward-related responding PPTg neuron activity was recorded while monkeys were executing a visual saccade task. First of all PPTg neurons showed burst firing not only during saccades but also during target fixation, suggesting a role in relaying the signal indicating the necessary performance of goal-directed behaviour (84). The existence of abundant excitatory projections to midbrain DA neurons from the PPTg is consistent with Kobayashi’s hypothesis that the PPTg is involved in reward prediction error computing (85). Recording studies showed that during rewarded trials of a visual saccade task, the PPTg activity increased gradually after the onset of the fixation target and remained elevated until reward delivery, whereas the activity decreased during trials when no reward was delivered. According to the authors, this shows that a high level of prediction of an upcoming reward was associated with enhanced activity of PPTg neurons. This, together with an abrupt increase in firing in response to completely unpredicted rewards supports the claim of an implication of the PPTg in prediction error signalling (85). Subsequent studies identified neurons in PPTg that responded in a graded manner to stimuli predicting reward and a separate population of neurons that responded to the reward itself (86).
In addition to these studies, the PPTg has been investigated for its role in sleep states, and in particular, transitions between REM sleep, slow wave sleep and the waking state. There is no doubt that PPTg neurons are active during the waking state and REM sleep, but quiet during slow wave sleep. As noted above, the PPTg has a profound control over thalamic activity – and hence cortical activation and information processing – that has been the object of study of sleep labs worldwide (see for example (4)). As is the case with locomotion, however, while the PPTg is most clearly involved with sleep regulation, this is only one among many functions and does not identify a unique function or the nucleus.
2.4 Imaging
Other methods of measuring neuronal activity are quantification of Fos-immunoreactivity, 2-deoxyglucose autoradiography, and in situ hybridization for the first subunit of cytochrome oxidase messenger RNA. Increased activity in the PPTg in 6-hydroxydopamine lesioned animal models for PD is shown by 2-deoxyglucose autoradiography (12) and measurement of cytochrome oxidase (10) provides support for a role of the PPTg in the physiopathology of PD.
Given its proposed role in the regulation of nicotine reinforcement (87) Lanca et al. investigated the extent of nicotinic activation in the LTDg and PPTg (70) by measuring Fos expression after systemic administration of nicotine. Nicotine-induced Fos activity was found to be above baseline levels in both the PPTg and LDTg, and double labelling of Fos and nicotinamide adenine dinucleotide phosphate diaphorase (NADPH-d – this is a nitric oxide synthase that is, in this region of brain, selectively expressed by cholinergic neurons) showed that it is mainly non-cholinergic neurons that show nicotine-induced activity. These results are interesting especially in the light of later findings that show involvement of the PPTg in nicotine self-administration (88). Moreover, these animal data are consistent with human imaging studies that show elevated activity in the PPTg in abstinent nicotine addicts exposed to stimuli associated with the drug (47).
2.5 New Horizons: Optogenetics
Optogenetics is a new technique that allows for reversible activation of specific neuronal populations (see Plotkin et al., Volume I, chapter 10). Optogenetic techniques for activating or disabling neurons (depending on the opsin used) transiently have enormous potential for functionally dissecting the properties for targeted groups of neurons from within a heterogeneous population (89). Though not done yet, it is undoubtedly feasible to produce transgenic rat or mouse lines in which cholinergic neurons express Cre. Cre (Cre recombinase) is an enzyme that can be used to delete a segment of DNA flanked by LoxP sites (that is, “floxed”). The deletion effectively produces a mutation linked to specific cells. Deploying a CRE-inducible adeno-associated virus vector carrying the gene encoding the light-activated cation channel channelrhodopsin-2 (fused with yellow fluorescent protein; ChR2-EYFP) could allow optogenetic modulation of cholinergic neurons in the PPTg in a temporally accurate and reversible manner. Mouse models are available for this (90); for our purposes, the production of a rat model would have considerably more power given the more extensive behavioural repertoire of the lab rat compared to mouse.
3 What Have We Learned?
In this review, we have outlined a number of the ways in which it is currently possible – and might in the immediate future be possible – to manipulate or monitor the PPTg. Numerous labs around the world have engaged with experimental studies of PPTg functions using a raft of different techniques – lesioning it in whole or part to determine what the consequence of its absence are; stimulating it, recording from it or imaging it. What is clear from the literature is that, while once there might have been reluctance to engage experimentally with such a deep brain structure, the work of many labs – and using a range of lab animal species – has shown that it is (with care) perfectly possible to engage with the PPTg just as it is with forebrain structures where such techniques are used routinely. What is also of interest is that, en masse, the studies point not just to involvement of the PPTg with locomotion and sleep – the traditional functions – but to a much wider engagement with processes that might be called “cognitive”. These include processing relating to reward and reinforcement, learning, attention and the selection of appropriate actions. Anyone about to start examining the PPTg needs to take cognizance of this breadth of functional properties.
Interest in the PPTg in regard to movement disorders has been greatly increased by the possibility that low frequency DBS here might improve gait and locomotion in Parkinsonian patients. There were two reasons for looking at the PPTg in regard to Parkinsonism (1) it is clearly altered in the disease, both through neuronal loss and through changed activity, most likely the result of changed input from the basal ganglia; and (2) it remains the case that the PPTg is considered still as a “motor structure” whose primary function is the production of coordinated movement. In this review, we have tried to shift the focus from the PPTg as a brainstem nucleus responsible for locomotion towards the idea that it has an even more complex function. This discussion resembles the debate about the “mysterious motor functions” of the basal ganglia in the 1980s (91).
Since the late seventeenth century, the basal ganglia were thought to be the “seat of motor power”, a concept that held well into the twentieth century (92). The basal ganglia are undoubtedly involved in movement disorders but new knowledge about the anatomical and physiological properties of the basal ganglia made it impossible to maintain this idea of the basal ganglia as a structure of “pure” motor control. Therefore, the understanding of what a movement disorder actually is and the concept of motor control had to be re-assessed. In the 1982, Robert Wartenberg Lecture David Marsden analyzed in detail those “mysterious motor functions” (91). Electrophysiological studies had revealed that the basal ganglia nuclei did not show activity in relation to specific movements but rather to more complex stimuli. Further, discrete lesions of the basal ganglia caused deficits similar of frontal lobe syndrome (a comparison we have also suggested for deficits observed after PPTg lesions (53)). The basal ganglia were seen to be involved in what could be thought of in some sense as non-motor functions. Marsden summarized: “the striatum takes in information from virtually all areas of the nervous system to undertake some unknown transformation into instructions to the output zones of the GPi and SNr whose own output is coded in relation to motor behavior” (p. 518) and that the basal ganglia were responsible for the “automatic execution of learned motor plans” (p. 534). This shifted basal ganglia function towards a function that critically involved learning. Disorders such as PD are very much understood in these terms – not as muscular disorders per se but involving problems with the selection and execution of actions.
< div class='tao-gold-member'>
Only gold members can continue reading. Log In or Register a > to continue
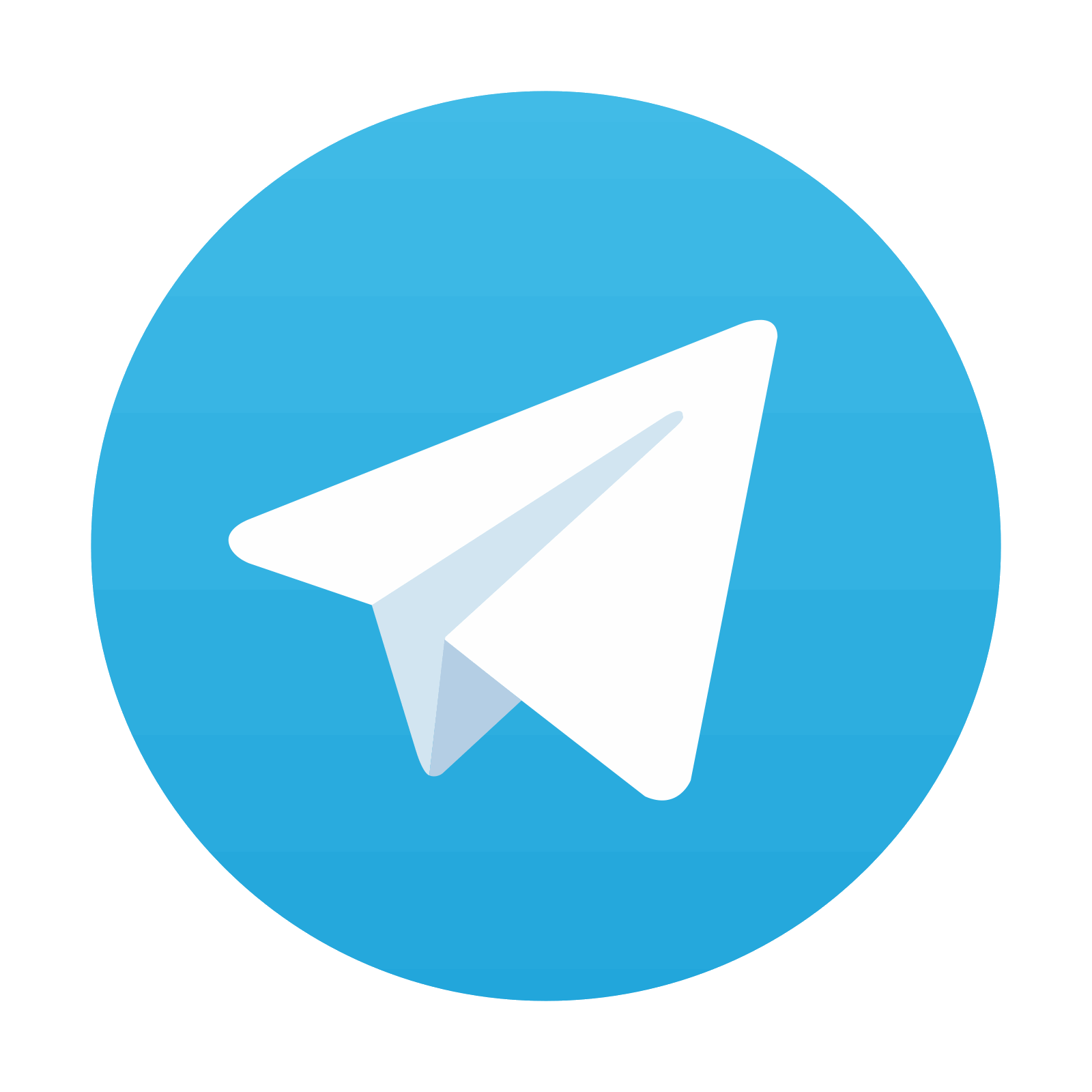
Stay updated, free articles. Join our Telegram channel
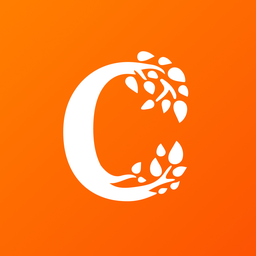
Full access? Get Clinical Tree
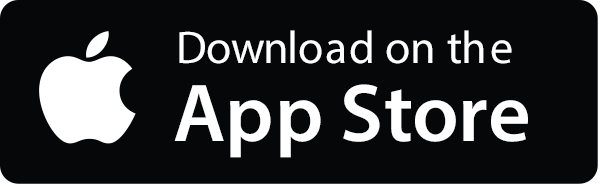
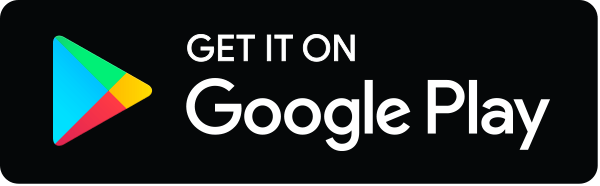