Chapter 7 The Erythrocyte Physiology, Metabolism, and Biochemical Disorders A. Species Differences in Erythrocyte Shape A. Stem Cells and Progenitor Cells B. Hematopoietic Microenvironment C. Hematopoietic Growth Factors III. DEVELOPING ERYTHROID CELLS A. Morphological and Metabolic Changes E. Abnormalities in Erythroid Development E. Metabolism of Adenine Nucleotides K. Nature of Oxidants in Biology L. Metabolic Protection against Oxidants V. DETERMINANTS OF RBC SURVIVAL B. RBC Aging and Normal Life Spans VI. INHERITED DISORDERS OF RBCS A. Cytosolic Enzyme Deficiencies C. Miscellaneous Abnormalities Mammalian erythrocytes or red blood cells (RBCs) are anucleate cells that normally circulate for several months in blood despite limited synthetic capacities and repeated exposures to mechanical and metabolic insults. Their primary purpose is to carry hemoglobin (Hb), a heme-containing protein that accounts for more than 90% of the protein within RBCs (Quigley et al., 2004). The benefits of having Hb contained within cells, as opposed to free in plasma, include the much slower turnover in blood (free Hb has a half-life of only a few hours), the metabolic capability of RBCs to maintain iron in Hb in the functional ferrous state, and the ability to control Hb oxygen affinity by altering the concentrations of organic phosphates, especially 2,3-diphosphoglycerate (2,3DPG). In addition, the presence of free Hb in plasma in concentrations normally found in whole blood would exert an osmotic pressure several times greater than that normally exerted by plasma proteins, profoundly affecting the movement of fluid between the vascular system and tissues. Most RBCs in normal dogs, cats, horses, cattle, and sheep occur in the shape of biconcave disks (discocytes). The degree of biconcavity is most pronounced in dogs and less so in cats and horses. RBCs from goats generally have a flat surface with little surface depression; a variety of irregularly shaped RBCs (poikilocytes) may be present in clinically normal goats (Jain, 1986). The apparent benefit of the biconcave shape is that it gives RBCs high surface area-to-volume ratios and allows for deformations that must take place as they circulate. The RBC functions of oxygen transport, carbon dioxide transport, and hydrogen ion buffering are interrelated. Each Hb tetramer can bind four molecules of oxygen when fully saturated, forming oxyhemoglobin (OxyHb). Assuming a normal arterial pO2 of 100 mmHg and an Hb concentration of 150 g/l (15 g/dl) in blood, the presence of Hb-containing RBCs increases the oxygen carrying capacity of blood approximately 70 times that which could be transported dissolved in plasma (West, 1985). Approximately 10% of CO2 is transported dissolved in blood, 5% to 10% is transported bound to amine groups of blood proteins, and 80% to 85% is transported in the form of bicarbonate in normal individuals (Hsia, 1998; Jensen, 2004). Carbonic acid is formed when dissolved CO2 combines with water. This reaction occurs nonenzymatically but is accelerated by the presence of the carbonic anhydrase (CA), also called carbonate dehydratase, enzyme in RBCs. Bicarbonate is formed by the rapid spontaneous dissociation of carbonic acid as shown: Hb potentiates the formation of bicarbonate by buffering hydrogen ions and shifting the equilibrium of the reaction to the right. Carbamino groups are formed by the combination of CO2 with the terminal groups of proteins. The globin of Hb is the most important blood protein in this regard. The transportation of CO2 from the tissues to the lungs as carbamino groups is potentiated because deoxyhemoglobin (DeoxyHb) binds twice as much CO2 as OxyHb. The formation of carbamino groups can be represented as follows: Hb is the most important protein buffer in blood because it occurs in high concentration, has a relatively low molecular weight, and has a large number of histidine residues with pKa values close to 7.4, enabling them to function as effective buffers. It has about six times the buffering capacity of the plasma proteins. An additional factor of importance in contributing to the effectiveness of Hb as a blood buffer is the fact that DeoxyHb is a weaker acid than OxyHb. As a result, most of the H+ produced in the tissues under normal conditions is buffered as a direct result of the H+ uptake by DeoxyHb owing to an increase in effective pKa of Hb following release of oxygen to the tissues (West, 1985). Primitive hematopoietic stem cells (HSCs) appear to develop in the embryo from a common precursor cell for both endothelial and hematopoietic lineages. The first HSCs appear to develop as clusters of cells in the wall of the dorsal aorta, with subsequent development in the yolk sac, placenta, and fetal liver (Baron and Fraser, 2005). Beginning in midgestation and continuing throughout postnatal life, mammalian blood cells are produced continuously from HSCs within extravascular spaces of the bone marrow. HSCs are capable of proliferation, life-long self-renewal, and differentiation. HSCs replicate only once every 8 to 10 weeks (Abkowitz et al., 2002). The term hematopoietic progenitor cell (HPC) refers to cells that form colonies in bone marrow culture like HSCs but do not have long-term self-renewal capacities. HSCs and HPCs are mononuclear cells that cannot be distinguished morphologically from lymphocytes. The presence of a transmembrane glycoprotein termed cluster of differentiation antigen 34 (CD34) has been used to identify HSCs and early HPCs, but some HSCs (possibly inactive ones) lack CD34 (Gangenahalli et al., 2006). In addition, CD34 is also present on the surface of nonhematopoietic stem cells and vascular endothelial cells (Kucia et al., 2005; Wu et al., 2005). CD34 is believed to play a role in cell adhesion (Gangenahalli et al., 2006). The most primitive HSC has the capacity to differentiate into HPCs of all blood cell lineages and several cell types in tissue. The frequency of HSCs in the marrow is estimated to be <0.01% of nucleated marrow cells in adult mice and <0.0001% of nucleated marrow cells in adult cats (Abkowitz et al., 2002). HSCs produce HPCs that can give rise to one or more blood cell types. HPCs are much more numerous in marrow than are HSCs. Less than 2% of nucleated bone marrow cells in adult dogs are CD34+, but up to 18% CD34+ cells have been reported in neonatal pups (Faldyna et al., 2003; Suter et al., 2004). A common lymphoid progenitor cell is believed to give rise to B lymphocytes, T lymphocytes, lymphoid dendritic cells, and natural killer cells (Blom and Spits, 2006). A common myeloid progenitor cell is believed to give rise to all nonlymphoid blood cells, as well as macrophages, myeloid dendritic cells, osteoclasts, and mast cells (Kaushansky, 2006a). HPCs proliferate with higher frequency than do HSCs, but the self-renewal capabilities of HPCs decrease as progressive differentiation and cell lineage restrictions occur. When measured in an in vitro cell culture assay, HPCs are referred to as colony-forming units (CFUs). HPCs that rapidly proliferate, retain their ability to migrate, and form multiple subcolonies around a larger central colony in culture are called burst-forming units (BFUs). Colony-forming unit-granulocyte, RBC, macrophage, megakaryocyte (CFU-GEMM) is a tetrapotential HPC that has been studied extensively in vitro. The existence of a bipotential HPC (CFU-GM) that is the precursor of both neutrophils (and possibly other granulocytes) and monocytes is well established, and recent studies indicate the likelihood of a bipotential HPC for RBCs and megakaryocytes (Kaushansky, 2006a). Blood cell production occurs throughout life in the bone marrow of adult animals because of the unique microenvironment present there. The hematopoietic microenvironment is a complex meshwork composed of stromal (fibroblast-like) cells, endothelial cells, adipocytes, macrophages, subsets of lymphocytes, natural killer cells, and osteoblasts; extracellular matrix components; and glycoprotein growth factors that profoundly affect HSC and HPC engraftment, survival, proliferation, and differentiation (Abboud and Lichtman, 2006). Stromal cells and endothelial cells produce components of the extracellular matrix (ECM), including collagen fibers, basement membranes of vessels and vascular sinuses, proteoglycans, and glycoproteins. In addition to providing structural support, the ECM is important in the binding of hematopoietic cells and soluble growth factors to stromal cells and other cells in the microenvironment so that optimal proliferation and differentiation can occur by virtue of these cell-cell interactions. Collagen fibers produced by stromal cells may not have direct stimulatory effects on hematopoiesis but rather are permissive, promoting hematopoiesis by forming an inert scaffolding around which the other elements of the microenvironment are organized. Hematopoietic cells can adhere to collagen types I and VI. Adhesion molecules (most importantly β1-integrins) on the surface of hematopoietic cells bind to ECM glycoproteins such as vascular cell adhesion molecule-1 (VCAM-1), hemonectin, fibronectin, laminin, tenascin, vitronectin, and thrombospondin. The spectrum of expression of adhesion molecules on hematopoietic cells that will differentially bind to these ECM glycoproteins varies with the type, maturity, and activation state of the hematopoietic cells. In addition to anchoring cells to a given microenvironmental niche, binding of adhesion molecules on hematopoietic cells also plays a role in cell regulation by direct activation of signal pathways for cell growth, survival, and differentiation or by modulating responses to growth factors. Proteoglycans consist of a protein core with repeating carbohydrate glycosaminoglycans (GAGs) attached. Major proteoglycans in the marrow include heparan sulfate, chondroitin sulfate, hyaluronic acid, and dermatan sulfate. Proteoglycans enhance hematopoiesis by trapping soluble growth factors in the vicinity of hematopoietic cells and by strengthening the binding of hematopoietic cells to the stroma. Hematopoietic cells develop in microenvironmental niches within the marrow. HSCs are concentrated near trabecular bone where osteoblasts help regulate their numbers (Yin and Li, 2006). Erythroid cells develop around macrophages, megakaryocytes form adjacent to sinusoidal endothelial cells, and granulocyte development is associated with stromal cells located away from the vascular sinuses (Abboud and Lichtman, 2006; Kaushansky, 2006a). Proliferation of HSCs and HPCs cannot occur spontaneously but requires the presence of specific hematopoietic growth factors (HGFs) that may be produced locally in the bone marrow (paracrine or autocrine) or produced by peripheral tissues and transported to the marrow through the blood (endocrine). All cells in the hematopoietic microenvironment, including the hematopoietic cells themselves, produce HGFs or inhibitors of hematopoiesis. Some HGFs have been called poietins (erythropoietin and thrombopoietin). Other growth factors have been classified as colony-stimulating factors (CSFs) based on in vitro culture studies. Finally, some HGFs have been described as interleukins (ILs) (Kaushansky, 2006b). Hematopoietic cells coexpress receptors for more than one HGF on their surface. The number of each receptor type present depends on the stage of cell activation and differentiation. Binding of an HGF to its receptor results in a series of enzymatic reactions that generate signals that promote the synthesis of molecules that inhibit apoptosis, the formation of cell-cycle regulators (cyclins), and the synthesis of additional HGFs and their receptors (Kaushansky, 2006b). HGFs vary in the type(s) of HSCs or HPCs that they can stimulate to proliferate. Factors are often synergistic in their effects on hematopoietic cells. In some instances, an HGF may not directly stimulate the proliferation of a given cell type but may potentiate its proliferation by inducing the expression of membrane receptors for HGFs that do stimulate proliferation. Some glycoproteins such as IL-1 and tumor necrosis factor-α (TNF-α) can modulate hematopoiesis indirectly by stimulating marrow stromal cells, endothelial cells, and T cells to produce HGFs. Different combinations of HGFs regulate the growth of different types of HSCs or HPCs (Kaushansky, 2006a). Early-acting HGFs are involved with triggering dormant (GO) primitive HSCs to begin cycling. Stem cell factor (SCF), flt3 ligand (FL), and thrombopoietin are important early factors that act in combination with one or more other cytokines such as IL-3, IL-6, IL-11, and granulocyte-CSF (G-CSF). Intermediate-acting HGFs have broad specificity. IL-3 (multi-CSF), granulocyte-macrophage-CSF (GM-CSF), and IL-4 support proliferation of multipotent HPCs. These factors also interact with late-acting factors to stimulate proliferation of a wide variety of committed progenitor cells. Late-acting HGFs have restricted specificity. Macrophage-CSF (M-CSF), G-CSF, erythropoietin, thrombopoietin, and IL-5 are more restrictive in their actions. They have their most potent effects on committed progenitor cells and later stages of development when cell lines can be recognized morphologically (Kaushansky, 2006b). Primitive RBC production begins and predominates in the yolk-sac but also occurs later in the liver and bone marrow. Primitive RBCs are large (>400 fl in humans) generally nucleated cells with high nuclear-to-cytoplasmic ratios. Their nuclei have open (noncondensed) chromatin, and their cytoplasm contains predominantly embryonal Hb with high oxygen affinity (Segel and Palis, 2006; Tiedemann, 1977; Tsuji-Takayama et al., 2006). Like nonmammalian species, primitive RBCs enter blood as nucleated cells, but in contrast to nonmammalian species, enucleation can eventually occur in the circulation (Kingsley et al., 2004). Primitive RBCs appear to be generated in an erythropoietin (Epo)-independent manner, but their expansion and survival require Epo (Tsuji-Takayama et al., 2006). A switch to definitive erythropoiesis occurs during fetal development. Definitive erythropoiesis results in the production of smaller cells that generally extrude their nuclei before entering blood, produce fetal Hb (in some species) and adult Hb, and are highly dependent on Epo (Tsuji-Takayama et al., 2006). Oligopotent progenitor cells (including CFU-GEMM cells) are stimulated to proliferate and differentiate into BFU-E by SCF, IL-3, and GM-CSF in the presence of Epo. BFU-E proliferation and differentiation into CFU-E results from the presence of these same factors and may be further potentiated by additional growth factors. Epo is the primary growth factor involved in the proliferation and differentiation of CFU-E into rubriblasts, the first morphologically recognizable erythroid cells. CFU-E cells are more responsive to Epo than are BFU-E cells because CFU-E cells exhibit greater numbers of surface receptors for Epo (Sawada et al., 1990). Marrow macrophages are important components of the hematopoietic microenvironment involved with erythropoiesis. Both early and late stages of erythroid development occur with intimate membrane apposition to central macrophages in so-called blood islands. Several adhesion molecules are important in forming these blood islands (Chasis, 2006). These central macrophages may regulate basal RBC production by producing both positive growth factors, including Epo, and negative factors such as IL-1, TNF-α, transforming growth factor-β, and interferon-α, –β, and –γ (Chasis, 2006; Weiss and Goodnough, 2005; Zermati et al., 2000). The finding that Epo can also be produced by erythroid progenitors suggests that these cells may support erythropoiesis by autocrine stimulation (Stopka et al., 1998). Although some degree of basal regulation of erythropoiesis occurs within the marrow microenvironment, humoral regulation is important, with Epo production occurring primarily within peritubular interstitial cells of the kidney and various inhibitory cytokines being produced at sites of inflammation throughout the body. Epo is a 34 kDa glycoprotein hormone that exhibits a high degree of sequence homology among mammals (Wen et al., 1993). It is the principal HGF that promotes the viability, proliferation, and differentiation of erythroid progenitor cells expressing specific cell surface Epo receptors. The main mechanism used to achieve these effects is inhibition of apoptosis. The binding of Epo to its receptor results in autophosphorylation of the receptor and the activation of several kinases that initiate multiple signaling pathways (Eckardt and Kurtz, 2005). Early BFU-E cells do not express Epo receptors, but more mature BFU-E cells express Epo receptors and are responsive to Epo. Epo receptor copies on cell surfaces increase to maximum values in CFU-E cells and then decline in rubriblasts and continue to decrease in later stages of erythroid development (Porter and Goldberg, 1993; Prchal, 2006). Because of their Epo receptor density, CFU-E cells readily respond to Epo, promoting their proliferation, differentiation, and transformation into rubriblasts, the first morphologically recognizable erythroid cell type. High concentrations of Epo may accelerate rubriblast entry into the first mitotic division, shortening the marrow transit time and resulting in the early release of stress reticulocytes (Prchal, 2006). In the presence of Epo, other hormones including androgens, glucocorticoid hormones, growth hormone, insulin, and insulin-like growth factors (IGFs) can enhance the growth of erythroid progenitor cells in vitro (Leberbauer et al., 2005; Miyagawa et al., 2000). However, growth factors may have additional effects in vivo. For example, growth hormone and IGF-1 are reported to decrease Epo synthesis in rat kidneys (Sohmiya and Kato, 2005). Glucocorticoids promote the differentiation of embryonic stem cells to hematopoietic cells and prolong the proliferation of erythroid progenitor cells but reduce the rate of spontaneous differentiation and terminal maturation of erythroid cells (Leberbauer et al., 2005; Srivastava et al., 2006). Glucocorticoids appear to be important in stress erythropoiesis (e.g., following hemorrhage or increased RBC destruction) when a substantial increase in erythropoiesis is required (Bauer et al., 1999). The thyroid hormone 3,5,3’-triiodothyronine (T3) promotes the differentiation and maturation of erythroid cells toward enucleated RBCs (Leberbauer et al., 2005). Thyroid hormones may also promote the synthesis of Epo in the kidney (Ma et al., 2004). Epo production in adult mammals occurs primarily within peritubular interstitial cells that are located within the inner cortex and outer medulla of the kidney. The liver is an extrarenal source of Epo in adults and the major site of Epo production in the mammalian fetus (Jelkmann, 2007). Bone marrow macrophages and erythroid progenitor cells themselves have also been shown to produce Epo, suggesting the possibility of short-range regulation of erythropoiesis (Stopka et al., 1998; Vogt et al., 1989). The ability to deliver oxygen to the tissues depends on cardiovascular integrity, oxygen content in arterial blood, and Hb oxygen affinity. Low oxygen content in the blood can result from low partial pressure of oxygen (pO2) in arterial blood, as occurs with high altitudes or with congenital heart defects in which some of the blood flow bypasses the pulmonary circulation. Low oxygen content in blood can also be present when arterial pO2 is normal, as occurs with anemia and methemoglobinemia. An increased oxygen affinity of Hb within RBCs results in a decreased tendency to release oxygen to the tissues (McCully et al., 1999). Epo production is stimulated by tissue hypoxia, which is mediated by hypoxia-inducible factors (HIFs) that are heterodimers consisting of α and β subunits. An α subunit denoted 2α is most important in Epo production, at least for definitive erythropoiesis. Both α and β subunits are continuously translated, but α subunits are labile and regulated by tissue oxygen levels. At normal tissue oxygen levels in tissue (pO2 > 36 mmHg), α subunits are hydroxylated by prolyl hydroxylases, polyubiquitinated, and removed by proteasomal degradation. When tissue oxygen levels are low (pO2 < 36 mmHg), α subunits are no longer hydroxylated and degraded, allowing them to translocate into the nucleus and combine with β subunits to form heterodimeric transcription factors. These HIF heterodimers activate the transcription of the Epo gene, and many other target genes, by binding to the hypoxia responsive elements (HREs) in their promoter/enhancer regions. Binding to the Epo gene results in increased Epo synthesis when tissue hypoxia is present (Gruber et al., 2007; Jelkmann, 2007). Other tissues also exhibit Epo receptors, and Epo also stimulates nonhematopoietic actions including promoting proliferation and migration of endothelial cells, enhancing neovascularization, stimulating the production of modulators of vascular tone, and exerting cardioprotective and neuroprotective effects (Jelkmann, 2007). Rubriblasts are large cells (approximately 900 fl in humans) that are continuously generated from progenitor cells in the extravascular space of the bone marrow. The division of a rubriblast initiates a series of approximately 5 divisions over a period of 3 to 5 days to produce about 32 metarubricytes that are no longer capable of division (Prchal, 2006). These divisions are called maturational divisions because there is a progressive maturation of the nucleus and cytoplasm concomitant with the divisions. Each division yields a smaller cell with greater nuclear condensation and increased Hb synthesis. An immature RBC, termed a reticulocyte, is formed following extrusion of the nucleus (Harvey, 2001). Early precursors have intensely blue cytoplasm, when stained with Romanowsky-type bloodstains, owing to the presence of many basophilic ribosomes and polyribosomes that are actively synthesizing globin chains and smaller amounts of other proteins. As the cells are nonsecretory, rough endoplasmic reticulum is scant and limited to early erythroid precursors (Bessis, 1973). Hb progressively accumulates in these cells, imparting a red coloration to the cytoplasm. Cells with both red and blue coloration are described as having polychromatophilic cytoplasm (Harvey, 2001). Kinetics of erythroid cells and changes in biochemical and metabolic pathways are depicted in Figure 7-1; time intervals were determined for cattle (Rudolph and Kaneko, 1971). Erythroid precursors have iron requirements that far exceed the iron requirements of any other cell type because of the need for Hb synthesis. Developing erythroid cells generally extract about 75% of the iron circulating in plasma (Smith, 1997). Plasma iron is bound to apotransferrin, a beta globulin that can maximally bind two atoms of ferric iron per molecule. The proportion of apo-, mono-, and diferric forms of transferrin present in serum depends on the percentage saturation of transferrin with iron. Diferric transferrin is more efficient than monoferric transferrin in delivering iron to cells, because it binds with higher affinity to receptors and can deliver twice the iron per molecule of transferrin incorporated (Huebers et al., 1985). Transferrin molecules transport iron to erythroid cells and bind to transferrin receptor-1 (TfR1) on cell surfaces. Transferrin-TfR1 complexes localize to clathrin-coated pits, which invaginate to initiate endocytosis (Beutler, 2006). After the complexes are internalized as endosomes, a proton pump decreases the pH in the endosome, resulting in conformational changes in the proteins and subsequent release of Fe+3 ions from transferrin. Following reduction with NADPH-dependent ferrireductase, Fe+2 is exported from the endosome using divalent metal transporter-1 (Ohgami et al., 2005). The resultant apotransferrin-TfR1 complex is recycled to the cell membrane, where apotransferrin is released from the cell, and the receptor is again available for binding additional transferrin molecules. FIGURE 7-1 Summary of metabolic activities of the erythroid series. Maturation progresses from left to right. The time intervals indicated are for cattle. From Kaneko, 1980, with permission. A direct interorganelle transfer of iron occurs between endosomes and mitochondria (Sheftel et al., 2007). Most iron within mitochondria is incorporated into protoporphyrin IX to form heme, but some mitochondrial iron is used to synthesize iron-sulfur complexes that are important prosthetic groups for numerous proteins involved in electron transfer, metabolic, and regulatory processes (Lill et al., 2006). Some iron is presumably released from endosomes into a cytoplasmic labile iron pool (LIP). Various chaperone molecules have been proposed, but the nature of the iron in the LIP remains enigmatic (Beutler, 2006). Iron not required for iron-sulfur complex or heme synthesis is stored as ferritin within the cytoplasm. Each ferritin molecule is composed of a protein shell of 24 apoferritin subunits surrounding a central core containing as many as 4000 iron atoms as ferric hydroxide (Arosio and Levi, 2002). Individual ferritin molecules can be visualized by electron microscopy, and large aggregates of ferritin molecules can be visualized by light microscopy when stained for iron using the Prussian blue reaction. When membrane bound, ferritin aggregates have been called siderosomes (Bessis, 1973). Iron stored as ferritin is not available for Hb synthesis in erythroid cells (Ponka et al., 1998). Apoferritin, TfR1, and erythroid-specific 5-aminolevulinic acid synthase (eALAS) synthesis are regulated by the LIP concentration. An increase in the LIP stimulates apoferritin synthesis and inhibits TfR1 expression to minimize the potential of iron toxicity to the cell. A decrease in the cytoplasmic LIP results in decreased apoferritin synthesis and increased TfR1 expression on cell surfaces to maximize iron uptake and use for heme synthesis (Beutler, 2006). RBCs coordinate protoporphyrin IX formation with the availability of iron by increasing the synthesis of eALAS (rate limiting enzyme in porphyrin synthesis) when the LIP is high and decreasing eALAS synthesis when the LIP is low. Anucleated RBCs containing siderotic (iron-positive) inclusions are called siderocytes. Nucleated siderocytes have been called sideroblasts in human hematology, in which terminology used for RBC precursors is generally different from that conventionally used in veterinary hematology (Bottomley, 2004). Siderotic inclusions in erythroid cells may consist of cytoplasmic ferritin aggregates or iron-loaded mitochondria. Ferritin aggregates can occur normally in nucleated erythroid cells of humans (Cartwright and Deiss, 1975), dogs (Feldman et al., 1981), and pigs (Deiss et al., 1966), but the presence of iron-loaded mitochondria is a pathological finding (Cartwright and Deiss, 1975). Electron microscopy is used to definitively identify the nature of siderotic inclusions (Fresco, 1981; Hammond et al., 1969); however, the location of iron-positive inclusions in a ring around the nucleus of a nucleated siderocyte (termed ringed sideroblast in human hematology) strongly suggests the presence of iron-loaded mitochondria (Bottomley, 2004). Conditions resulting in the pathological iron accumulation in mitochondria may induce the synthesis of a novel mitochondrial ferritin (Torti and Torti, 2002). Except for iron deficiency, disorders in heme synthesis have the potential to cause excess iron accumulation in mitochondria (Beutler, 1995b; Fairbanks and Beutler, 1995). Experimental pyridoxine deficiency and experimental chronic copper deficiency have both resulted in mitochondrial iron overload in nucleated erythroid cells in bone marrow of deficient pigs (Hammond et al., 1969; Lee et al., 1968a). Drugs or chemicals reported to cause siderocytes or nucleated siderocytes in dogs include chloramphenicol (Harvey et al., 1985), lead, hydroxyzine, zinc (Harvey, 2001), and an oxazolidinone antibiotic (Lund and Brown, 1997). Siderotic inclusions in erythroid cells have been recognized in some dogs and cats with myeloproliferative disorders (Blue et al., 1988; Weiss and Lulich, 1999). Acquired dyserythropoiesis with siderocytes have been reported in dogs in which specific etiologies could not be determined, although some of these animals had inflammatory disorders (Canfield et al., 1987; Weiss, 2005). Congenital anemias with ringed nucleated siderocytes have been reported in humans (Bottomley, 2006). Persistent siderotic inclusions have been recognized in microcytic hypochromic erythrocytes from an English bulldog. Erythrocytes also contained Heinz bodies and rare hemoglobin crystals (Harvey et al., 2007). A congenital defect resulting in mitochondrial iron overload and secondary oxidant injury was suspected, but not identified. Refer to Chapter 9 for more information concerning iron metabolism. Hb is a tetrameric protein consisting of four polypeptide globin chains each of which contains a heme prosthetic group within a hydrophobic pocket. The molecule consists of two identical alpha and two nonalpha chains that are generally classified as beta chains in adults. Heme is a planar molecule composed of the tetrapyrrole protoporphyrin IX, containing a central ferrous molecule. The initial rate-controlling step in heme synthesis, the eALAS reaction, occurs within mitochondria (see Chapter 8). Glycine and the Krebs cycle intermediate succinyl-CoA are utilized as substrates, and vitamin B6, as pyridoxal phosphate, is required as a cofactor. The ALA formed is transported to the cytoplasm where a series of reactions results in the formation of coproporphyrinogen III, which must enter the mitochondria, presumably using an ATP-binding cassette transporter ABCB6 (Krishnamurthy et al., 2006) for the final steps in heme synthesis. The final reaction, catalyzed by heme synthetase, involves the insertion of ferrous iron into protoporphyrin IX. Following synthesis, heme must be transferred from mitochondria to the cytoplasm for combination with globin chains to complete the synthesis of Hb. The mitochondrial heme exporter has not been identified at this time. Free heme is poorly soluble in water and can bind to and damage cellular components (Kumar and Bandyopadhyay, 2005). It apparently is bound to cytosolic proteins for transport to sites of globin chain synthesis (Kumar and Bandyopadhyay, 2005; Taketani et al., 1998). Sometime after its synthesis, the iron moiety of heme is oxidized (presumably spontaneously) to the ferric state and is then more specifically called ferriheme (Schulman et al., 1974). Heme affects erythroid cell metabolism in different ways depending on the stage of maturation. It stimulates iron uptake and heme synthesis in early erythroid cells, but it inhibits iron uptake and heme synthesis in reticulocytes (Abraham, 1991; Battistini et al., 1991). A cellular heme exporter termed feline leukemia virus subgroup C cellular receptor (FLVCR) is up-regulated on colony-forming units-erythroid (CFU-E) progenitor cells. It may provide a safety mechanism to prevent the accumulation of toxic amounts of cytoplasmic heme before globin synthesis is initiated. The synthesis of polypeptide globin monomers occurs in association with ribosomes and polyribosomes in the cytoplasm. Evidence has been provided indicating that binding of a partially unstructured apo-β chain to a tightly folded holo-α chain to form a heme-deficient dimer is the initial step of Hb assembly. Such binding locks the β chain in a highly ordered conformation, which allows for an efficient heme acquisition by the β chain. (Griffith and Kaltashov, 2003). Free α globin chains are highly unstable and tend to aggregate within the cell and generate reactive oxygen species through chemical reactions catalyzed by their heme-bound iron molecules. Fortunately, free α globin chains are stabilized by binding to alpha Hb stabilizing protein (AHSP) until they combine with free β globin chains to form stable α–β dimers (Weiss et al., 2005). Two like α–β dimers spontaneously combine in a readily reversible manner to form Hb tetramers (Bunn, 1987). Newly synthesized Hb is in the form of methemoglobin (MetHb) (Schulman et al., 1974). Even though three different pathways are required for Hb synthesis in RBC precursors and reticulocytes, minimal intermediates (iron, globin chains, or heme) accumulate in the cytoplasm of these cells. Several positive and negative feedback mechanisms account for the balanced production of these Hb components. As already discussed, an increase in the LIP limits the uptake of additional iron by decreasing TfR1 synthesis. The availability of iron also limits and, thereby controls, heme synthesis. Free “uncommitted” heme inhibits iron uptake by erythroid cells and consequently heme synthesis. In addition, free heme is essential for the synthesis of globin chains at both the transcriptional and translational levels (Koury and Ponka, 2004). Consequently, globin synthesis does not occur in the absence of heme. Hb types are different in animal and human embryos than in fetuses or in adults. Embryonal Hbs are composed of either one or two pairs of peptide chains not found in adult Hbs (Kitchen and Brett, 1974). In higher primates, embryonal Hbs are replaced by fetal Hb composed of 2 α chains and 2 γ chains (HbF) in the fetus, followed by Hbs with 2 α chains and 2 β chains (HbA) or 2 α chains and 2 δ chains (HbA2) after birth. The γ globin gene was deleted during evolution in artiodactyls (even-toed ungulates). The γ globin gene functions as an embryonic gene in other nonartiodactyl mammals. Among nonprimate mammals, a specific fetal globin gene is expressed only in artiodactyls. In this lineage, a duplicated β globin gene (βF) is expressed in the fetus, rather than a γ globin gene. The entire β globin gene locus was duplicated in cattle and HbB sheep and triplicated in goats and HbA sheep during evolution (Gumucio et al., 1996). Most fetal Hb in cattle ( In addition to expressing fetal Hb ( FIGURE 7-2 Changes in proportion of β globin types in Hb from five newborn goats during the first 120 days of life. Fetal Hb contains βF. HbC contains βC. HbD differs from HbA by a single amino acid substitution in position 21 of their β chains, βD and βA, respectively. Modification of a figure by Huisman et al., 1969. The switch from production of fetal Hb to the production of adult Hb appears to result from an inherent programming of hematopoietic stem cells (Wood et al., 1985). In contrast, HbC synthesis appears to be mediated by Epo, which is low at birth and increases after birth as the hematocrit decreases (see Section V.C) (Barker et al., 1980; Huisman et al., 1969). Adult sheep RBCs contain little or no HbC, but adult goat RBCs may have as much as 10% HbC (Huisman et al., 1969). Sheep and goats that synthesize HbC during the neonatal period will also increase HbC synthesis in anemic adults and in nonanemic adults treated with Epo (Vestri et al., 1983; Yu et al., 2005). Most goats and sheep with HbA have 12 genes in the β-globin locus, including the βC gene. Cattle and sheep with HbB have 8 genes in the β-globin locus and lack the βC gene (Garner and Lingrel, 1988). HbC was not reported to occur in three breeds of Omani goats after birth even though they were reported to be either homozygous for HbA or heterozygous for HbA and HbB (Johnson et al., 2002). The composition of the β-globin locus was not investigated in these animals. The α-globin genes have been duplicated in humans and other mammals. Triplication of α-globin genes is relatively common in sheep and quadruplication of genes also occurs. There is an expression gradient of α-globin protein and α-globin mRNA levels produced by duplicated and triplicated α-globin genes, based on their location in the α-globin gene cluster. Gene expression progressively decreases from 5’ to 3’ (Vestri et al., 1994). Compared to sheep with duplicated α-globin genes, sheep with triplicated α-globin genes produce excess α-globin, resulting in an unbalanced alpha/beta ratio and larger RBCs with increased osmotic fragility (Pieragostini et al., 2003). Considerable heterogeneity of Hb types occurs in adult animals. Two or more Hb types are reported to occur in domestic animal species (Braend, 1988; Kitchen, 1974; Kohn et al., 1998, 1999; Pirastru et al., 2003; Rando and Masina, 1985). Most polymorphism of animal Hbs is determined genetically and is usually caused by multiple amino acid interchanges (Kitchen, 1974). Hb types were initially classified based on electrophoretic mobility in gels. Electrophoretic differences can result from differences in α or β chain structure. For example, HbA and HbB in sheep are attributable to differences in β chains (Garner and Lingrel, 1988), but HbA and HbB in goats are attributed to differences in α chains (Huisman and Kitchens, 1968; Pieragostini et al., 2005). Electrophoretic mobility in gels is not sensitive enough to identify many α and β chain variants, and distinctly different Hb tetramers can migrate with similar mobilities, resulting in misleading information. For example, HbB ( Nongenetic alterations in Hb structure can also contribute to apparent Hb heterogeneity. Examples include the N-acetylation of beta chains in cat HbB (Taketa et al., 1972) and glycosylation of Hb, a function of intracellular glucose concentration and RBC life span (Higgins et al., 1982; Rendell et al., 1985). Increased glycosylation of Hb has been reported in diabetic dogs and cats (Elliott et al., 1997, 1999). Similar to the glycosylation of Hb by glucose, cyanate combines spontaneously and irreversibly with Hb to form carbamylated Hb. Cyanate forms spontaneously from urea. Dogs with chronic renal failure and long-term increases in urea concentration have larger amounts of carbamylated Hb than dogs with acute renal failure (Heiene et al., 2001). Most reticulocytes are formed from metarubricytes within the extravascular space of the bone marrow by a process of nuclear extrusion that requires functional microtubules (Chasis et al., 1989) and is likened to mitosis (Bessis, 1973; Simpson and Kling, 1967). The erythroblast macrophage protein (Emp) associates with F-actin and is important in denucleation of metarubricytes, as well as blood island formation (Soni et al., 2006). Extruded nuclei quickly expose phosphatidylserine on their surfaces, which promotes their binding to, and phagocytosis by, blood island macrophages (Yoshida et al., 2005). Reticulocyte cytoplasm contains ribosomes, polyribosomes, and mitochondria necessary for the completion of Hb synthesis. Reticulocytes derive their name from a network or reticulum that appears when stained with basic dyes such as methylene blue and brilliant cresyl green. That network is not preexisting but is an artifact formed by the precipitation of ribosomal ribonucleic acids and proteins. As reticulocytes mature, the amount of ribosomal material decreases until only a few basophilic specks can be visualized with reticulocyte staining procedures. These mature reticulocytes have been referred to as type IV (Houwen, 1992) or punctate reticulocytes (Alsaker, 1977; Perkins et al., 1995). To reduce the chance that a staining artifact would result in misclassifying a mature RBC as a punctate reticulocyte using a reticulocyte stain, the cell being evaluated should have two or more discrete blue granules that are visible without requiring fine focus adjustment of the cell to be classified as a punctate reticulocyte. Reticulocyte metabolism and maturation have been reviewed by Rapoport (1986) and are summarized here. Immature reticulocytes continue to synthesize protein (primarily globin chains) with residual mRNA, tRNA, and rRNA formed before denucleation. About 30% of total Hb is synthesized after nuclear extrusion (Geminard et al., 2002). Synthesis of fatty acids is minimal, but phospholipids are synthesized from preformed fatty acids. Substrates for protein and lipid synthesis and for energy metabolism are provided from endogenous sources (breakdown of mitochondria and ribosomes) as well as from plasma. The reticulocyte can synthesize adenine and guanine nucleotides de novo (Rapoport, 1986). Most ATP is generated in reticulocytes by oxidative phosphorylation in mitochondria. Glucose is the major substrate, but amino acids and fatty acids can also be utilized for energy (Rapoport, 1986). Reticulocyte maturation into mature RBCs is a gradual process that requires a variable number of days depending on the species involved. Consequently, the morphological and physiological properties of reticulocytes vary with the stage of maturation. Early reticulocytes have polylobulated surfaces. The cell surface undergoes extensive remodeling with loss of membrane material and ultimately the formation of the biconcave shape of mature RBCs (Bessis, 1973). The loss of membrane protein and lipid components requires ATP and involves the formation of intracellular multivesicular endosomes that fuse with the plasma membrane releasing vesicles (exosomes) extracellularly (Geminard et al., 2002). This is a highly selective process in which some proteins (e.g., TfR1 and fibronectin receptor) are lost and cytoskeletal proteins (e.g., spectrin) and firmly bound transmembrane proteins (e.g., the anion transporter and glycophorin A) are retained and concentrated (Geminard et al., 2002; Ponka et al., 1998). The heat shock protein 70 (Hsp70) is concentrated in exosomes and may promote the formation of exosomes during reticulocyte maturation (Jeong et al., 2005). Some membrane proteins such as the nucleoside transporter, glucose transporter, Na,K-ATPase, insulin receptor, and adrenergic receptors decrease to variable degrees depending on the species involved (Chasis et al., 1989; Geminard et al., 2002). Examples of reticulocytes from a species that exhibit a complete or nearly complete loss of a protein that is retained in mature RBCs from other species include the adenosine transporter in sheep (Jarvis and Young, 1982), the glucose transporter in pigs (Zeidler and Kim, 1982), and Na,K-ATPase in dogs (Maede and Inaba, 1985). Although loss of membrane components accounts for much of the change in membrane protein composition during reticulocyte maturation, certain proteins such as protein 4.1 and glycophorin C increase because they are still being synthesized in reticulocytes (Chasis et al., 1989). These membrane alterations result in increased mechanical stability of blood reticulocyte and RBC membranes compared to marrow reticulocyte and nucleated erythroid cell membranes (Waugh et al., 2001). Mitochondria undergo degenerative changes in a programmed death phenomenon (mitoptosis) owing to 15-lipoxygenase attack and subsequent ATP-dependent proteolysis (Geminard et al., 2002). Degenerating mitochondria are either digested or extruded following entrapment in structures resembling autophagic vacuoles (Simpson and Kling, 1968). The polysomes separate into monosomes and decrease in number and disappear as reticulocytes mature into RBCs. The degradation of ribosomes appears to be energy dependent; it presumably involves proteases and RNAases (Rapoport, 1986). Reticulocyte maturation begins in the bone marrow and is completed in the peripheral blood and spleen in dogs, cats, and pigs. As reticulocytes mature, they lose the surface receptors needed to adhere to fibronectin and thrombospondin components of the extracellular matrix, presumably facilitating their release from the bone marrow (Telen, 2000). Residual adhesion molecule receptors on newly released reticulocytes may explain their tendency to concentrate in the reticular meshwork of the spleen (Patel et al., 1985). Reticulocytes become progressively more deformable as they mature, a characteristic that also facilitates their release from the marrow (Waugh et al., 2001). To exit the extravascular space of the marrow, reticulocytes press against the abluminal surfaces of endothelial cells that make up the sinus wall. Cytoplasm thins and small pores (0.5 to 2 μm) develop in endothelial cells, which allow reticulocytes to be pushed through by a small pressure gradient across the sinus wall (Lichtman and Santillo, 1986; Waugh, 1991). Pores apparently close after cell passage. Relatively immature aggregate-type reticulocytes are released from canine bone marrow; consequently, most of these cells appear polychromatophilic when viewed following routine blood film staining procedures (Laber et al., 1974). Absolute reticulocyte counts oscillate with a periodicity of approximately 14 days in some dogs, suggesting that canine erythropoiesis may have a homeostatically controlled physiological rhythm (Morley and Stohlman, 1969). Reticulocytes are normally not released from feline bone marrow until they mature to punctate-type reticulocytes; consequently, few or no aggregate reticulocytes (<0.4%), but up to 10% punctate reticulocytes, are found in blood from normal adult cats (Cramer and Lewis, 1972). The high percentage of punctate reticulocytes results from a long maturation time with delayed degradation of ribosomes (Fan et al., 1978). Reticulocytes are generally absent in peripheral blood of healthy adult cattle and goats, but a small number of punctate types (0.5%) may occur in adult sheep (Jain, 1986). Based on microscopic examination of blood films stained with new methylene blue, equine reticulocytes are absent from blood normally and rarely released in response to anemia. However, low numbers of reticulocytes have been reported in the blood of normal and anemic horses using an Advia 120 (Siemens Medical Solutions Diagnostics, Tarrytown, New York) automated analyzer (Cooper et al., 2005). Either the instrument is more sensitive than microscopic evaluation, or values reported in normal horses represent “noise” in the instrument. Except for horses, increased numbers of reticulocytes are released in response to anemia, with better responses to hemolytic anemia than to hemorrhage. When the degree of anemia is severe, basophilic macroreticulocytes, or so-called stress reticulocytes, may be released into blood. It is proposed that a generation in the maturation sequence is skipped and immature reticulocytes, about twice the normal size, are released (Rapoport, 1986). Increased Epo results in a diminution in the adventitial cell and endothelial cell barrier separating marrow hematopoietic cells from the sinus, thereby potentiating the premature release of stress reticulocytes from the marrow (Chamberlain et al., 1975). Although a portion of these macroreticulocytes apparently is rapidly removed from the circulation (Noble et al., 1990), it is clear from studies in cats that some can mature into macrocytic RBCs with relatively normal life spans (Weiser and Kociba, 1982). Ineffective erythropoiesis is a term used to describe the destruction of developing erythroid cells in marrow. Normally, few die within the marrow (Odartchenko et al., 1971), but ineffective erythropoiesis is prominent in disorders of nucleic acid, heme, or globin synthesis. Examples include folate deficiency, iron deficiency, vitamin B6 deficiency, lead poisoning, and thalassemia in humans (Jandl, 1987). Ineffective erythropoiesis also occurs in association with myeloproliferative and myelodysplastic disorders (Meyer and Harvey, 2004) and congenital dyserythropoiesis (Holland et al., 1991; Steffen et al., 1992). Folate is required for normal DNA synthesis. Folate deficiency impairs the activity of the folate-requiring enzyme thymidylate synthase (Jandl, 1987). Not only is deoxythymidylate triphosphate (dTTP) synthesis decreased, but deoxyuridylate triphosphate (dUTP) accumulates secondarily in the cell such that some becomes incorporated into DNA in place of dTTP. Cycles of excision and attempts to repair these copy errors, with limited thymidine available, result in chromosomal breaks and malformations and slowing of the S phase in the cell cycle. Consequently, erythroid precursors are often large with deranged-appearing nuclear chromatin; such cells are classified as megaloblastic cells. Folate deficiency in people causes macrocytic anemia because fewer divisions occur as a result of retarded nucleic acid synthesis in the presence of normal protein synthesis (Jandl, 1987). Possible causes of folate deficiency include dietary deficiency, impaired absorption, and drugs that interfere with folate metabolism. Macrocytic anemias resulting from folate deficiency are rarely reported in animals. A possible case was reported in a dog on anticonvulsant therapy (Lewis and Rebar, 1979), but serum folate was not measured. Megaloblastic precursors were present in bone marrow of cats with experimental dietary folate deficiency, but hematocrits and mean cell volumes (MCVs) remained normal (Thenen and Rasmussen, 1978). However, macrocytic anemia with dyserythropoiesis was reported in a cat that appeared to have both a folate-deficient diet and defective folate absorption (Myers et al., 1996). Macrocytic anemia has been reported in folate-deficient pigs (Bush et al., 1956), but not lambs (Stokstad, 1968). Vitamin B12 (cobalamin) deficiency in people causes hematological abnormalities similar to folate deficiency because vitamin B12 is necessary for normal folate metabolism in humans (Chanarin et al., 1985). In contrast, vitamin B12 deficiency does not cause macrocytic anemia in any animal species (Chanarin et al., 1985). Anemia has been reported in some experimental animal studies, but RBCs were of normal size (Stokstad, 1968; Underwood, 1977), although slight increases in MCV have been reported in B12-deficient goats fed diets deficient in cobalt (Mgongo et al., 1981). Cobalamin deficiency has been reported secondary to an inherited malabsorption of cobalamin in giant schnauzer dogs (Fyfe, 2000). Affected animals have normocytic, nonregenerative anemia with increased anisocytosis and poikilocytosis, neutropenia with hypersegmented neutrophils, and giant platelets. Megaloblastic changes in the bone marrow were particularly evident in the myeloid cell line. The malabsorption of cobalamin in these dogs apparently results from the absence of an intrinsic factor-cobalamin receptor in the apical brush border of the ileum. No blood or bone marrow abnormalities were recognized in kittens fed a B12-deficient diet for several months (Morris, 1977), but a normocytic nonregenerative anemia was present in a cobalamin-deficient cat that probably had an inherited disorder of cobalamin absorption (Vaden et al., 1992). A number of disorders exhibit macrocytic anemias with megaloblastic abnormalities in the marrow that mimic findings in human folate or cobalamin deficiency but have had normal serum levels of these vitamins when measured. Examples include cats infected with the feline leukemia virus (Dunn et al., 1984; Weiser and Kociba, 1983a), cattle with congenital dyserythropoiesis (Steffen et al., 1992), and myelodysplastic syndromes (Harvey, 2001). In addition, some miniature and toy poodles exhibit macrocytosis without anemia and variable megaloblastic abnormalities in the bone marrow with normal serum folate and cobalamin values (Canfield and Watson, 1989). Abnormalities in heme or globin synthesis can result in the formation of microcytic hypochromic RBCs. Cellular division is normal, but Hb synthesis is delayed; consequently, one or more extra divisions occur in RBC development, resulting in smaller cells than normal. Pyridoxine, vitamin B6, is required for the first step in heme synthesis. Although natural cases of pyridoxine deficiency have not been documented in domestic animals, microcytic anemias with high serum iron values have been produced experimentally in dogs (McKibbin et al., 1942), cats (Bai et al., 1989; Carvalho da Silva et al., 1959), and pigs (Deiss et al., 1966) with dietary pyridoxine deficiency. Erythroid cells with iron-loaded mitochondria, secondary to impaired heme synthesis, have been demonstrated in pigs fed a pyridoxine-deficient diet (Hammond et al., 1969). With the exception of young growing animals, iron deficiency in domestic animals usually results from blood loss. Milk contains little iron; consequently, nursing animals can easily deplete their body iron store as they grow (Furugouri, 1972; Harvey et al., 1987; Holter et al., 1991; Siimes et al., 1980). Microcytic RBCs are produced in response to iron deficiency (Holman and Drew, 1966; Holter et al., 1991; Reece et al., 1984), but a low MCV may not develop postnatally in species where the MCV is above adult values at birth (Weiser and Kociba, 1983b). The potential for development of severe iron deficiency in young animals appears to be less in species that begin to eat food at an early age. Chronic iron deficiency anemia with microcytic RBCs is common in adult dogs in areas where hookworm and flea infestations are severe (Harvey et al., 1982; Weiser and O’Grady, 1983). Severe iron deficiency appears to be rare in adult cats (French et al., 1987; Fulton et al., 1988) and horses (Smith et al., 1986), but it occurs frequently in ruminants that are heavily parasitized with blood-sucking parasites such as Haemonchus contortus. Much more information concerning iron deficiency is given in Chapter 9. Prolonged copper deficiency generally results in anemia in mammals (Auclair et al., 2006; Brewer, 1987; Lahey et al., 1952), although anemia was not a feature of experimental copper deficiency in the cat (Doong et al., 1983). The anemia is generally microcytic hypochromic; however, normocytic anemia has been reported in experimental studies in dogs, and normocytic or macrocytic anemias have been reported in cattle and adult sheep (Brewer, 1987). Copper deficiency results in impaired iron metabolism (Lee et al., 1968b). In experimental studies in pigs, serum iron concentration is low in early copper deficiency when iron stores are normal (Lahey et al., 1952; Lee et al., 1968b). Functional iron deficiency occurs because copper-containing proteins hephaestin and ceruloplasmin are required for normal iron transport (Lee et al., 1968a; Wessling-Resnick, 2006). If experimental copper deficiency is prolonged in pigs, hyperferremia occurs and nucleated erythroid cells with cytoplasmic siderotic (iron-positive) inclusions increase in bone marrow (Lee et al., 1968a). Reticulocyte mitochondria from copper-deficient pigs are unable to synthesize heme at the normal rate using Fe+3 (Williams et al., 1976). A deficiency in copper-containing cytochrome oxidase within mitochondria may slow the reduction of Fe+3 to Fe+2 within mitochondria. That would limit heme synthesis, which requires iron in the Fe+2 state (Porra and Jones, 1963). Hereditary deficiencies in synthesis of the globin α chain (α-thalassemia) and β chain (β-thalassemia) cause microcytic hypochromic anemias in humans with variable degrees of poikilocytosis (Weatherall, 2006). Both α– and β-thalassemia occur in mice, but thalassemias have not been reported in domestic animals. Aplastic anemia is generally used to describe anemias where granulocytic, megakaryocytic, and erythrocytic cell lines are markedly reduced in the bone marrow. When only the erythroid cell line is reduced or absent, terms such as pure red cell aplasia, selective erythroid aplasia, or selective erythroid hypoplasia are used. Many drugs have been incriminated in the production of aplastic anemia in humans (Shadduck, 1995). Drug-induced causes of aplastic anemia or generalized marrow hypoplasia in animals include estrogen toxicity in dogs, phenylbutazone toxicity in dogs and possibly a horse, trimethoprim-sulfadiazine administration in dogs, bracken fern poisoning in cattle and sheep, trichloroethylene-extracted soybean meal in cattle, albendazole toxicity in dogs and cats, griseofulvin toxicity in cats, various cancer chemotherapeutic agents, and radiation. Thiacetarsamide, meclofenamic acid, and quinidine have also been incriminated as potential causes of aplastic anemia in dogs. In addition to exogenous estrogen toxicity, high levels of endogenous estrogens, produced by estrogen secreting tumors and functional cystic ovaries in dogs and prolonged estrus in ferrets, can result in aplastic anemia (Harvey, 2001). Parvovirus infections can cause erythroid hypoplasia, as well as myeloid hypoplasia in canine pups (Potgieter et al., 1981; Robinson et al., 1980), but animals may not become anemic, because of the long life spans of RBCs. Although some degree of marrow hypoplasia or dysplasia often occurs in cats with feline leukemia virus (FeLV) infections (Cotter, 1979), true aplastic anemia is not a well-documented sequela (Rojko and Olsen, 1984). Hypocellular bone marrow has been reported in cats experimentally co-infected with FeLV and feline parvovirus (Lutz et al., 1995). Dogs with acute Ehrlichia canis infections may spontaneously recover or develop chronic disease that generally exhibits some degree of marrow hypoplasia. Although rare, aplastic anemia may develop in association with severe chronic ehrlichiosis in dogs (Neer, 1998). A retrospective review of cats with aplastic anemia identified 13 cases in which the clinical diagnoses included chronic renal failure (n = 5), FeLV infection (n = 2), hyperthyroidism treated with methimazole (n = 1), and idiopathic aplastic anemia (n = 5). The author suggested that starvation may have played a role in the development of marrow aplasia in some of these cats (Weiss, 2006). Idiopathic aplastic anemia has also been reported in dogs and horses (Harvey, 2001). CFU-E were not detected in bone marrow culture from a dog with an idiopathic aplastic anemia (Weiss and Christopher, 1985). Other potential causes of aplastic anemia include congenital defects and primary immune-mediated disorders. Most cases of aplastic anemia in adult humans are immune mediated, with activated type 1 cytotoxic T cells implicated in the pathogenesis (Young et al., 2006). The aberrant immune response may be triggered by environmental exposures, such as to chemicals and drugs or viral infections, and possibly by endogenous antigens generated by genetically altered bone marrow cells (Young, 2002). Selective erythroid aplasia (pure red cell aplasia) occurs as either a congenital or acquired disorder in people. Acquired erythroid aplasia is often associated with abnormalities of the immune system. Erythroid aplasia may also occur secondary to disorders such as B-19 parvovirus infection, lymphoid malignancies, and drug or chemical toxicities (Erslev and Soltan, 1996). Acquired erythroid hypoplasia or aplasia occurs in dogs (Stokol et al., 2000; Weiss, 1986). Some cases have immune-mediated etiologies based on positive responses to immunosuppressive therapy and the presence of antibodies that inhibit CFU-E development in marrow cultures (Weiss, 1986). Acquired, presumably immune-mediated, erythroid aplasia has also been reported in FeLV-negative cats (Stokol and Blue, 1999). Selective erythroid aplasia occurs in cats infected with FeLV subgroup C (FeLV-C), but not in cats infected only with subgroups A or B (Riedel et al., 1986). CFU-E numbers are markedly decreased but BFU-E numbers are normal in infected cats, indicating that FeLV-C inhibits differentiation of BFU-E into CFU-E (Abkowitz, 1991). The cell surface receptor for FeLV-C is called FLVCR. This receptor has recently been demonstrated to be a heme exporter (Quigley et al., 2004). Free heme is toxic to cells, and it is hypothesized that the binding of FeLV-C to FLVCR on CFU-E progenitor cells inhibits heme export from these cells, resulting in their destruction (Quigley et al., 2004). High doses of chloramphenicol cause reversible erythroid hypoplasia in some dogs (Watson, 1977) and erythroid aplasia in cats (Watson and Middleton, 1978). Marked erythroid hypoplasia has been reported in dogs, cats, and horses given recombinant human erythropoietin (Cowgill et al., 1998; Piercy et al., 1998; Woods et al., 1997). Antibodies made against this human recombinant glycoprotein apparently cross-react with the animals’ endogenous erythropoietin. Values for RBC glucose utilization, ion concentrations, and survival times in normal animals are given in Table 7-1. Enzyme activities are given in Tables 7-2 and 7.3, and chemical constituents in RBCs are given in Tables 7-4 and 7.5. These are not, however, comprehensive lists. Other values are provided by Friedemann and Rapoport (1974) and in various chapters of a reference book edited by Agar and Board (1983). Anemia induced by phlebotomy or by hemolytic drugs produces changes in many of the previously stated values owing to the influx of young RBCs into the circulation in response to the anemia (Agar and Board, 1983). Methods for enzyme assays vary considerably; consequently, each laboratory will need to establish its own reference intervals if enzyme studies are to be done. TABLE 7-1 Erythrocyte Glucose Utilization, Ion Concentrations, and Survival Times of Various Mammalsa a Mean values have been recalculated at times to permit direct comparisons between species. Standard deviation values are given where indicated. Abbreviation: RBC, red blood cell. Figures in parentheses are the references cited as follows: (1) Harvey and Kaneko, 1976a; (2) Magnani et al., 1980; (3) Leng and Annison, 1962; (4) Harkness et al., 1970; (5) Kim and McManus, 1971; (6) Laris, 1958; (7) Beutler, 1995a; (8) Coldman and Good, 1967; (9) Contreras et al., 1986; (10) Tucker and Ellory, 1971; (11) Vacha, 1983; (12) Miseta et al., 1993. The RBC membrane is composed of a hydrophobic lipid bilayer with a protein skeletal meshwork attached to its inner surface by binding to integral (transmembrane) proteins (Fig. 7-3). Membrane proteins from RBCs have been numbered by their migration location (Smith, 1987) on sodium dodecyl sulfate-polyacrylamide gel electrophoresis (SDS-PAGE); some have also been given one or more names. Electrophoretic patterns of membrane proteins on SDS-PAGE are species variable (Gillis and Anastassiadis, 1985; Kobylka et al., 1972; Smith et al., 1983a; Whitfield et al., 1983). TABLE 7-2 Erythrocyte Enzymes of Various Animal Speciesa a All enzyme units are U/g Hb, except ATPase given in μmoles phosphorus liberated/g Hb/h. Mean values have been recalculated at times to permit direct comparisons between species. Standard deviation values are given where indicated. Abbreviations: HK, hexokinase; GPI, glucose phosphate isomerase; PFK, phosphofructokinase; TPI, triosephosphate isomerase; GAPD, glyceraldehyde-3-phosphate dehydrogenase; PGK, phosphoglycerate kinase; MPGM, monophosphoglycerate mutase; PK, pyruvate kinase; LDH, lactate dehydrogenase; AST, aspartate aminotransferase; DPGM, diphosphoglycerate mutase; DPGP, diphosphoglycerate phosphatase; G6PD, glucose-6-phosphate dehydrogenase; 6PGD, 6-phosphogluconate dehydrogenase; FAD, flavin adenine dinucleotide; GR ( + FAD), glutathione reductase with FAD added to assay; GR ( + FAD), glutathione reductase without FAD added to assay; GPx, glutathione peroxidase; GST, glutathione S-transferase; SOD, superoxide dismutase; Cb5R, cytochrome-b5 reductase; NADPH-D, reduced nicotinamide adenine dinucleotide phosphate diaphorase; Na+-K+-ATPase, Na+-K+-adenosine triphosphatase. Temperatures above 25°C are included in reference citations. Figures in parentheses are the references cited as follows: (1) Beutler, 1984 (at 37°C, except Cb5R at 30°C); (2) Harvey et al., unpublished, 2007 (at 37°C, except Cb5R at 30°C); (3) Harvey and Kaneko, 1975a; (4) Maede and Inaba, 1987 (at 37°C); (5) Kurata et al., 1993; (6) Harkness et al., 1969; (7) Franken and Schotman, 1977; (8) Schechter et al., 1973; (9) Smith et al., 1972a; (10) Gupta et al., 1974 (at 44°C). The lipid bilayer and associated transmembrane proteins chemically isolate and regulate the cell interior. The bilayer consists of phospholipids arranged with hydrophobic hydrocarbon chains of fatty acids to the center of the bilayer and the polar ends of the molecules in contact with both intracellular and extracellular aqueous environments. Molecules of unesterified cholesterol are intercalated between fatty acid chains in molar concentrations approximately equal to the sum of the molar concentrations of phospholipids. Phospholipids are asymmetrically arranged, with anionic amino-containing phospholipids (phosphatidylserine and most of the phosphatidylethanolamine) located in the inner layer or leaflet of the bilaminar membrane. After uptake from plasma, these phospholipids are shuttled (flipped) from the outer leaflet to the inner leaflet by an ATP-dependent aminophospholipid-specific translocase or flippase (Zwaal et al., 1993). Most of the cationic choline-containing phospholipids, phosphatidylcholine (lecithin) and sphingomyelin, are located in the outer layer (Delaunay, 2007). These choline-containing phospholipids are readily exchangeable with plasma phospholipids, whereas the aminophospholipids are not (Reed, 1968). RBCs also contain a Ca+2-dependent phospholipid scramblase that is activated by a protein kinase C catalyzed phosphorylation. This scramblase accelerates the bidirectional transbilayer movement of phospholipids. The inhibition of the flippase or the activation of the scramblase can result in increased phosphatidylserine in the outer layer, which promotes coagulation and erythrophagocytosis (Delaunay, 2007; Mandal et al., 2005). TABLE 7-3 Erythrocyte Enzymes of Various Animal Speciesa a All enzyme units are U/g Hb, except ATPase given in μmoles phosphorus liberated/g Hb/h. Mean values have been recalculated at times to permit direct comparisons between species. Standard deviation values are given where indicated. Abbreviations: HK, hexokinase; GPI, glucose phosphate isomerase; PFK, phosphofructokinase; TPI, triosephosphate isomerase; GAPD, glyceraldehyde-3-phosphate dehydrogenase; PGK, phosphoglycerate kinase; MPGM, monophosphoglycerate mutase; PK, pyruvate kinase; LDH, lactate dehydrogenase; AST, aspartate aminotransferase; DPGM, diphosphoglycerate mutase; DPGP, diphosphoglycerate phosphatase; G6PD, glucose-6-phosphate dehydrogenase; 6PGD, 6-phosphogluconate dehydrogenase; FAD, flavin adenine dinucleotide; GR, glutathione reductase; GPx, glutathione peroxidase; GST, glutathione S-transferase; SOD, superoxide dismutase; Cb5R, cytochrome-b5 reductase; Na+-K+-ATPase, Na+-K+-adenosine triphosphatase. Temperatures above 25°C are included in reference citations. Figures in parentheses are the references cited as follows: (1) Zinkl and Kaneko, 1973b; (2) Smith et al., 1972b; (3) Agar and Smith, 1973; (4) Agar and Smith, 1974; (5) McManus, 1967; (6) Harkness et al., 1969; (7) Agar et al., 1974a; (8) Kurata et al., 1993; (9) Del Boccio et al., 1986; (10) Maral et al., 1977; (11) Gupta et al., 1974 (at 44°C); (12) Suzuki and Agar, 1983; (13) Board and Agar, 1983. Species vary in RBC membrane phospholipid compositions (Garnier et al., 1984; Nelson, 1967; Wessels and Veerkamp, 1973). Cattle, sheep, and goat RBCs are unique in that their membranes contain little or no phosphatidylcholine and high sphingomyelin levels (Nouri-Sorkhabi et al., 1996). Another anomaly recognized in bovine RBCs is an extreme asymmetry of phosphatidylethanolamine, with only 2% of the total present in the outer leaflet (Florin-Christensen et al., 2001). Although RBC cholesterol/phospholipid ratios remain relatively constant, differences occur in membrane phospholipid composition between neonate and adult RBCs (Marin et al., 1990). TABLE 7-4 Erythrocyte Chemical Constituents of Various Speciesa a Given in nmole/ml RBC, except lactate and pyruvate, which are given in nmole/ml whole blood. Mean values have been recalculated at times to permit direct comparisons between species. Standard deviation values are given where indicated. Abbreviations: G6P, glucose 6-phosphate; F6P, fructose 6-phosphate; FDP, fructose 1,6-diphosphate; DHAP, dihydroxyacetone phosphate; 3PG, 3-phosphoglycerate; 2PG, 2-phosphoglycerate; PEP, phosphoenolpyruvate; AMP, adenosine monophosphate; ADP, adenosine diphosphate; ATP, adenosine triphosphate; 2,3-DPG, 2,3-diphosphoglycerate; Pi, inorganic phosphate; GSH, reduced glutathione; GSSG, oxidized glutathione. Figures in parentheses are the references cited as follows: (1) Beutler, 1984; (2) Harvey et al., unpublished, 2007 (using methods of Beutler, 1984); (3) Harvey et al., 1992b; (4) Smith and Agar, 1976; (5) Harkness et al., 1969; (6) Maede and Inaba, 1987; (7) Maede et al., 1982; (8) Snow and Martin, 1990. A small amount of glycolipid is also located in the outer layer. Species differ in the dominant glycolipids of RBCs (Eberlein and Gercken, 1971; Yamakawa, 1983). Based on studies of human blood group antigens, it is assumed that many animal blood group antigens are also glycolipids and that specificity resides in the carbohydrate moieties. Integral membrane proteins penetrate the lipid bilayer. These glycoproteins express carbohydrate residues on the outside surface of the cell. They contribute negative charge to the cell surface, function as receptors or transport proteins, and carry RBC antigens (Chasis and Mohandas, 1992; Mohandas and Chasis, 1993; Schrier, 1985). Band 3 (anion exchanger 1) is the major integral protein. It accounts for approximately one-fourth of the total membrane protein, with about 106 copies/RBC (Delaunay, 2007; Schrier, 1985). It is important as an anion transporter and provides a site for binding of the cytoskeleton internally. Additional transmembrane glycoproteins called glycophorins also help anchor and stabilize the cytoskeleton (Chasis and Mohandas, 1992). The membrane skeleton appears as a dense sweater-like meshwork bound to the inner surface of the lipid bilayer. It is a major determining factor of membrane shape, deformability, and durability (Mohandas and Chasis, 1993). A simplified cross section of the RBC membrane is shown in Figure 7-3. Spectrin is a heterodimer of long, flexible alpha and beta chains twisted around one another. Heterodimers are bound together by self-association at their head ends to form tetramers. Multiple spectrin tail ends (an average of six) are joined together by binding to common short filaments of actin and a variety of other proteins, including tropomyosin and adducin, to form an anastomosed meshwork of polygons (Fig. 7-4). These junctional complexes with actin are stabilized by other proteins, including protein 4.1 and protein 55. Protein 4.1 binds the meshwork to one or more integral membrane glycophorins (Delaunay, 2007). The meshwork is also bound to transmembrane band 3 by ankyrin in regions of spectrin self-association, and protein 4.2 may strengthen the ankyrin to band 3 linkage (Mohandas and Chasis, 1993; Rybicki et al., 1996). Band 4.2 has not been demonstrated in some species including horses (Guerra-Shinohara and Barretto, 1999). TABLE 7-5 Erythrocyte Chemical Constituents of Various Speciesa a Given in nmole/ml RBC, except lactate and pyruvate, which are given in nmole/ml whole blood. Mean values have been recalculated at times to permit direct comparisons between species. Standard deviation values are given where indicated. Abbreviations: G6P, glucose 6-phosphate; F6P, fructose 6-phosphate; FDP, fructose 1,6-diphosphate; DHAP, dihydroxyacetone phosphate; 3PG, 3-phosphoglycerate; 2PG, 2-phosphoglycerate; PEP, phosphoenolpyruvate; AMP, adenosine monophosphate; ADP, adenosine diphosphate; ATP, adenosine triphosphate; 2,3-DPG, 2,3-diphosphoglycerate; Pi, inorganic phosphate; GSH, reduced glutathione; GSSG, oxidized glutathione. Figures in parentheses are the references cited as follows: (1) Zinkl and Kaneko, 1973b; (2) Noble et al., 1983; (3) Agar and Smith, 1974; (4) Magnani et al., 1983; (5) Agar et al., 1983; (6) Harkness et al., 1969; (7) Agar et al., 1974a; (8) Travis et al., 1985; (9) Battaglia et al., 1970; (10) Srivastava and Beutler, 1969. FIGURE 7-3 Schematic model of the organization of the RBC membrane skeleton. The cytoskeletal meshwork assumes a condensed configuration in nondeformed discocytes, with spectrin tetramer fibers existing in folded or coiled states (Fig. 7-4
I. INTRODUCTION
A. Species Differences in Erythrocyte Shape
B. Functions of RBCs
II. HEMATOPOIESIS
A. Stem Cells and Progenitor Cells
B. Hematopoietic Microenvironment
C. Hematopoietic Growth Factors
D. Erythropoiesis
1. Primitive Erythropoiesis
2. Definitive Erythropoiesis
3. Erythropoietin
III. DEVELOPING ERYTHROID CELLS
A. Morphological and Metabolic Changes
B. Iron Metabolism
1. Transferrin and Transferrin Receptors
2. Intracellular Iron Transport
3. Ferritin
4. Iron Regulation of Transferrin Receptor and Ferritin Expression
5. Siderotic Inclusions in Erythroid Cells
C. Hb Synthesis
1. Heme Synthesis and Metabolism
2. Globin Synthesis
3. Control of Hb Synthesis
4. Hb Types in Animals
) is replaced by adult Hb types during the first 3 weeks after birth (Lee et al., 1971). Embryonal Hbs are replaced by adult Hb types during the fetal period in cats, dogs, horses, and pigs. Consequently, Hb types present in fetuses are identical to those found in adults in these species (Bunn and Kitchen, 1973; Kitchen and Brett, 1974).
) in the fetus, most goats and some sheep express a juvenile Hb (
) called HbC during the neonatal period that is subsequently replaced with adult Hb types. Sheep that produce adult HbA (
) express variable amounts (generally less than 10%) of HbC during the neonatal period, but sheep that produce adult HbB (
) do not express HbC in the neonatal period (Huisman et al., 1969; Yu et al., 2005). Fetal Hb in the newborn goat is replaced almost entirely by HbC during the first 40 days of life (Figure 7-2), and then HbC is gradually replaced by adult Hbs (Huisman et al., 1969).
) and HbD (
) in goats have almost the same mobility at alkaline pH (Pieragostini et al., 2005).
D. Reticulocytes
1. Formation
2. Metabolism
3. Maturation into RBCs
4. Species Differences in Marrow Release
5. “Stress” Reticulocytes
E. Abnormalities in Erythroid Development
1. Ineffective Erythropoiesis
2. Vitamin and Mineral Deficiencies
3. Deficiencies in Globin Synthesis
4. Aplastic Anemia
5. Selective Erythroid Aplasia
IV. MATURE RBC
A. Membrane Structure
1. Lipids
2. Integral Membrane Proteins
3. Membrane Skeletal Proteins
Stay updated, free articles. Join our Telegram channel
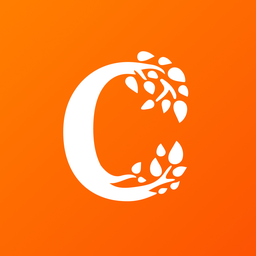
Full access? Get Clinical Tree
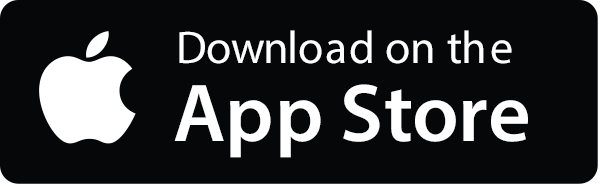
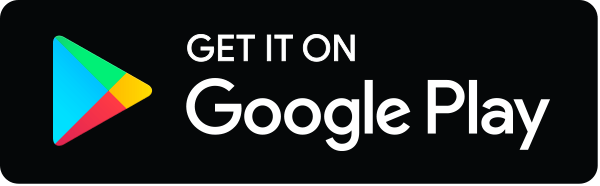