CHAPTER 13 Tendons, ligaments, bone, and cartilage are connective tissues in which the activities of various cellular populations are responsible for synthesis and maintenance of large amounts of extracellular matrix that should, theoretically, be dynamically optimized to respond to mechanical demands. One of the major reasons postulated for training horses is to increase musculoskeletal strength that would result in increased resistance to injury. However, many injuries are preceded by failure of the tissue to adapt to exercise, an imbalance between subclinical damage (microdamage) and cellular reparative activity, or both. Our knowledge of how various physical stimuli influence adaptation or damage of equine connective tissues prior to clinical injury is surprisingly limited, even in the case of bone that is more easily assessed than cartilage, tendon, or ligament tissue. It has become increasingly evident that a significantly greater understanding of the responses of cellular populations to various exercise regimens is required, including how various types, frequencies, magnitudes, rates, and durations of mechanical loading may be transduced into coordinated cellular control of matrix synthesis, degradation, and turnover. In tendon, in particular, the cell types and their connection into functional networks are very poorly defined. Ultimately, all of the macroscopic, microscopic, ultrastructural, or biochemical changes occurring in response to exercise are determined by cellular activity. Finally, in reviewing the various equine training studies that have been performed, it is important to recognize the difficulties involved in their direct comparisons. Quantification of the exercise performed has not been standardized, including calculation of loading cycles and magnitudes of forces applied, and in many, the intensity of training has been altered to allow for individual horses’ “fitness.” A cumulative work index, including distances worked at various speeds in various periods, has been proposed, but that requires refinement in terms of application to different locations; stages of training; and individual horse factors, including age and different environments (Firth and Rogers, 2005a). In some studies, researchers have effectively immobilized horses in control or “exercise” groups; in those studies where control animals were not confined, quantifying the amount of exercise during periods of natural activity has usually not been attempted. There is very limited information on “normal” frequencies, durations, and magnitudes of various activities for horses of various ages, breeds, and functions, in the enormous diversity of situations in which they either are kept or live in the wild. Studies using the global positioning system (GPS) technology are few. The “optimal” mechanical environment, in terms of appropriate growth, development, and adaptation of the musculoskeletal system during training and competitive athletic activity, remains unknown. This, together with the background of enormous individual variation, means that resistance to injury has not yet been maximized. Horses can attain high speeds largely because specific tendons and ligaments store and return elastic strain energy as the foot is set down and then lifted. This, effectively, halves muscular work during galloping. The tendons act as “biologic springs,” and horses are one of the species where tendons have reached their most extreme development. As a result, these are also clinically the most important tendons because of their susceptibility to injury; most current research information relates to superficial digital flexor tendon (SDFT) injury, one of the most common causes of lameness in Thoroughbreds in both racing and training and in sport horses. The mid-metacarpal region of the forelimb SDFT is most frequently injured, with rupture often initially located in the tendon core. In the suspensory ligament (SL), lesions may occur in the proximal one third (proximal suspensory desmitis), middle third (body lesions), or branches (branch lesions) (Dyson et al., 1995). It has been estimated that 10% to 30% of racehorses and horses in training experience SDFT or SL injury, with long periods out of work (up to 18 months) (Avella et al., 2009; Goodship et al., 1994; Pinchbeck et al., 2004; Williams et al., 2001). In the long term, in many horses, the SDFT or other components of the suspensory apparatus is reinjured. In one Japanese study, 70% of horses that had sustained tendon injuries failed to return to their previous level of performance (Jorgensen and Genovese, 2003; Jorgensen and Genovese, 2003; Oikawa and Kasashima, 2002). However, in a more recent study of Thoroughbred racehorses in the United Kingdom, SDFT injury did not cause a significant reduction in Racing Post Rating (published by the Racing Post newspaper) when these horses returned to racing (O’Meara et al., 2010). In the same study, it was concluded that the assessment of the outcome of an SDFT injury requires a minimum completion of five races on return to competition. Tendon injuries seem to occur more often in horses used for steeplechasing, and other types of high-level competition, including elite eventing and showjumping (Murray et al., 2006; Singer et al., 2008; Takahashi et al., 2004; Williams et al., 2001). Increased age was a significant risk factor in several epidemiologic studies (Avella et al., 2009; Perkins et al., 2005; Williams et al., 2001), which can be related to the pathophysiology of exercise-induced tendon injury. In trotters, there appears to be a high prevalence of SL injury, which is thought to reflect differences in load distribution between the flexor structures at the gallop and at the extended trot (Goodship et al., 1994). Anatomy of tendons and ligaments of the distal limb The suspensory apparatus of the fetlock (metacarpophalangeal and metatarsophalangeal joints) comprise the SDFT, the deep digital flexor tendon (DDFT), accessory ligaments of the flexor tendons, the SL, proximal sesamoid bones, intersesamoidean ligaments, and distal sesamoidean ligaments (Figure 13-1, A and B). The digital flexor muscles are thought to act largely to dampen the high-frequency and potentially damaging oscillations occurring in the limb during high-speed locomotion (Wilson et al., 2001). However, results of a recent study of muscle force and fascicle length in horses walking and cantering on a treadmill indicate that the muscle of the DDFT does play a role in supplementing mechanical work, in contrast to that of the SDFT (Butcher et al., 2009). Cross-sectional areas (CSAs) vary markedly along the lengths of digital tendons, with significant differences existing between individual horses; these differences are not based on height, weight or mid-metacarpal circumference (Smith et al., 1994). There are also breed differences, with ponies and purebred Spanish horses having smaller CSAs in the metacarpal region compared with Thoroughbreds (Agut et al., 2008; Smith et al., 1994). In the forelimb, the accessory ligament of the SDFT (radial or superior check ligament) originates on the caudomedial aspect of the distal radius and joins the tendon immediately proximal to the carpus. The SDFT measures approximately 45 cm in length, extending through the carpal canal, where it has a round shape, and down the palmar surface of the third metacarpal bone (Mc3), where it is crescent-shaped (Denoix, 1994). The SDFT widens and flattens considerably in the fetlock region, forming a fibrous ring (manica flexoria) proximal to the proximal sesamoid bones, through which the DDFT passes. The SDFT branches at the distal end of the proximal phalangeal bone (P1), with each branch inserting on the scutum medium, a fibrocartilaginous structure attached to the proximopalmar aspect of the middle phalangeal bone (P2) (Denoix, 1994). The DDFT also has an accessory ligament (carpal or tarsal check ligament, or inferior check ligament), which, in the forelimb, originates from the palmar carpal ligament, joining the tendon in the mid-metacarpal region (Shively, 1983); the accessory ligament of the DDFT in the hindlimb originates from the joint capsule and plantar ligaments of the tarsus but is poorly developed or absent in horses. The accessory ligaments are thought to prevent overstrain of the digital flexor muscles, functioning as part of the passive “stay” apparatus. The DDFT is round in cross-section in the metacarpal–metatarsal region, becoming oval as it passes through the manica flexoria and then widening at the palmar aspect of the fetlock joint. It passes between the SDFT insertions and over the palmar aspect of the navicular (distal sesamoid) bone to insert on the distal phalangeal bone (P3). The SL, underlying the DDFT, is a highly modified interosseous muscle that still contains large numbers of skeletal myofibers. It ranges from 20 to 25 cm long in the forelimb and 25 to 30 cm in the hindlimb (Denoix, 1994). Muscle tissue located within the structure of the SL is also thought to have a “damping” effect (Wilson et al., 1991). In the forelimb, the SL originates from the palmar carpal ligament and proximal palmar aspect of the third metacarpal bone and descends between the second and fourth metacarpal bones; in the hindlimb, the SL originates largely from the plantaroproximal aspect of the third metatarsal bone. The SL divides in the distal metacarpal–metatarsal region (the exact site varies) into two branches, each of which inserts on the abaxial surface of the corresponding proximal sesamoid bone, with thin extensor branches passing forward to join the common digital extensor tendon (CDET). The extensor branches may limit P1–P2 joint flexion and have been suggested to assist in orienting the foot just prior to impact (Jansen et al., 1993; Wilson et al., 1991). Distally, the suspensory apparatus continues as the sesamoidean ligaments (straight, oblique, cruciate, short), which are highly modified in horses in terms of number, arrangement, strength, and area of insertion, this being correlated in an evolutionary sense with development of the single-toed foot and the passive spring apparatus (Camp and Smith, 1942). In some sites, tendons are surrounded by a synovial sheath; a carpal synovial sheath surrounds the SDFT and DDFT in the carpal canal, with a mesotenon between them. The carpal synovial sheath extends 7 to 10 cm proximal to the antebrachiocarpal joint to the distal extent of the proximal third of the metacarpus (Denoix, 1994). An incomplete digital synovial sheath begins 4 to 7 cm proximal to the proximal sesamoid bones and extends to the middle of P2. When not within a synovial sheath, the tendons are surrounded by the paratenon, a layer of connective tissue that overlies loose connective and vascular tissue comprising the epitenon. The blood supply of tendons arises from the musculotendonous junction and the osseous insertion, between which are longitudinal intratendonous vessels and extratendonous vessels. The extratendonous vessels are located in (1) the paratenon, in extrasynovial locations or (2) the mesotenon that is variably present as remnants, termed vinculae, and pass from the wall of the tendon sheath to the tendon proper (Smith and Webbon, 1996). Two major blood vessels extend longitudinally in the lateral and medial borders of the mid-metacarpal SDFT, with extensively anastomosing vessels between them. Where tendons are surrounded by synovial sheaths, diffusion of nutrients is also important; injuries are less frequent in such regions in the horse (Smith and Webbon, 1996). Tendon tissue where it inserts into the bone may be fibrous or fibrocartilaginous, the latter with a transitional zone of hyaline cartilage. The microanatomy of tendons and ligaments is poorly understood in relation to other connective tissues. Despite their bland gross appearance, they have a complex uniaxial hierarchical structure (Figure 13-2), which has not yet been completely defined in terms of mechanical or biologic properties. There is disagreement in the literature on the nomenclature and nature of various hierarchical levels and subunits with certain terms, including collagen “fiber,” having many different definitions. Some of this confusion may relate to the initial studies of Kastelic et al. (1977), which were based on rat tail tendons, the structure of which cannot necessarily be directly extrapolated to locomotor tendons or to the specialized structures found in the equine distal limb. This model is the most commonly cited, but there is variation in its interpretation. The discussion below refers to data obtained from examination of equine tendons, wherever possible, and avoids the use of “fiber” as a term. Fascicles: Fascicles are the largest tendon subunits, measuring approximately 1 mm in diameter in the equine SDFT, with a variable polygonal shape in cross-section (see Figure 13-2) and an age-related reduction in CSAs (Gillis et al., 1997). They are separated by small amounts of loose connective tissue, termed the endotenon, which contains the vasculature. The inner surface of the epitenon, a fine connective tissue sheath underlying the paratenon that surrounds the whole tendon, is continuous with the endotenon. Fascicles appear to move independently, on the basis of in vitro cyclical loading studies of both equine and human tendon specimens, with small or negligible lateral force transmission (Goodship et al., 1994; Haraldsson et al., 2008). In addition to low interaction between fascicles, results from loading studies using human Achilles tendon specimens suggest that the overall mechanical responses of tendons may be determined by a subset of the “strongest” fascicles (Komolafe and Doehring, 2010). In contrast to rat tail tendon, it is not an easy matter to dissect out individual fascicles because of branching and spiraling along the longitudinal axis. Fascicles comprise collagen fibrils, with the extracellular matrix between them, and tenocytes. Blood vessels do not penetrate into the fascicle under normal circumstances. In the SL, skeletal muscle tissue comprises 2% to 11% of the total area (Dyson et al., 1995), arranged in bundles that vary in size and arrangement, often in the form of two longitudinally oriented bundles within the proximal part and body of the ligament that are less organized distally and differ in shape between the forelimb and the hindlimb (Wilson et al., 1991). Muscle tissue is associated with longitudinal sheets of adipose tissue. Standardbreds have 40% more muscle in the SL compared with Thoroughbreds, and higher muscle content in the hindlimb SL than in the forelimb SL (Wilson et al., 1991). Collagenous matrix: Approximately 70% of the tendon matrix consists of water. Collagen comprises approximately 75% to 80% of the dry weight of tendon tissue. Elastic fibers are also present but are a minor component in most tendons and ligaments. Collagen fibrils are the units of tensile strength in tendons and ligaments, in which they are submicroscopic cylindrical structures arranged in parallel in the longitudinal axis of the fascicle (see Figure 13-2); in ligaments, there tends to be greater variation in orientation. Although equine digital tendons show significant variation in CSAs along their lengths, the CSA is inversely proportional to total collagen content, dry weight of collagen, and percentage occupied by collagen fibrils (Birch et al., 2002; Riemersma and de Bruyn, 1986). This implies that (1) the injury-prone mid-metacarpal SDFT site with the smallest CSA would not be expected to be the weakest point, and (2) variation in CSAs in these tendons is largely due to the noncollagenous components of the matrix. In adult tissue, collagen fibrils consist of predominantly type I collagen (95%). Type III collagen occurs in small amounts within fascicles and is also found in the endotenon; small amounts of other collagen types, including III, IV, V, VI, XII and II, have also been identified. Type II collagen, normally associated with cartilage, is present in the fibrocartilaginous tendon regions that are subjected to compressive forces as they “wrap around” bony prominences. Collagen fibrils, like other matrix components, are synthesized by tenocytes (tendon fibroblasts) that are arranged in longitudinal rows between them. Tenocytes extend cytoplasmic processes to each other between and within their rows. It has been proposed that these cytoplasmic processes are sheetlike, enveloping bundles of collagen fibrils (McNeilly et al., 1996; Ralphs et al., 2002). Collagen fibrils and fibril bundles are thought to be assembled within complex and overlapping extracellular spaces formed by the tenocyte cytoplasmic membrane, which begin with the fusion of intracellular collagen secretory vacuoles with the surface (Birk and Trelstad, 1986). More recently, plasma membrane protrusions with lumens, termed “fibropositors,” have been identified in embryonic tendon projecting into parallel channels between cells, and the tips of these structures are the sites of fibril deposition in the extracellular matrix (Canty et al., 2004). The collagen fibrils follow a planar zigzag waveform, termed the “crimp,” which is visible histologically under polarized light as black-and-white banding and may be generated during embryonic development by tenocyte contraction (Herchenhan et al., 2012) (see Figure 13-2 and Figure 13-3). The crimp is thought to act as a mechanical safety buffer; straightening occurs under load (in the toe region of the stress–strain curve) before the fibril is itself stretched. A fibril with a low crimp angle will straighten and fail before a fibril with a relatively high crimp angle (Patterson-Kane et al., 1997a; Wilmink et al., 1991) (Figure 13-4). Collagen fibrils are thought to comprise subfibrils (as defined during in vitro collagen fibrillogenesis studies), which are, in turn, proposed to be formed by microfibrillar units. Each microfibril is a grouping of five tropocollagen molecules, which are arranged in a parallel, quarter-stagger array responsible for the repeating bands of fibrils visible in longitudinal sections under the electron microscope (see Figure 13-2). Each molecule comprises three polypeptide α-chains (in type I collagen two α-1 and one α-2), each of which is coiled in a left-handed helix (see Figure 13-2) with approximately 100 amino acids. Almost two thirds of the collagen molecule is composed of three amino acids: (1) glycine (33%), (2) proline (15%), and (3) hydroxyproline (15%). Tenocytes synthesize larger precursor (procollagen) molecules that are secreted extracellularly, followed by enzymatic cleavage of aminopropeptides and carboxyl propeptides. It is also possible for collagen synthesis and fibril formation to occur intracellularly (Canty et al., 2004). Cross-links are formed within and between the three helices of a molecule but also form between molecules, this being essential for the strength of the collagen fibril. Lysyl oxidase converts lysine and hydroxylysine residues to aldehydes, which can form reducible or nonreducible cross-links (e.g., lysylpyridinoline and hydroxylysylpyridinoline); levels of reducible cross-links decrease with age. In neonatal foals, the fibril diameter distribution in tendons and ligaments is unimodal (i.e., the histogram has a single peak), becoming bimodal during maturation (Parry et al., 1978a) (Figure 13-5). In theory, large fibrils are stronger because of their lower surface area per unit volume and consequent higher intrafibrillar density of collagen cross-links. Smaller fibrils, although weaker, may have greater interaction with the surrounding noncollagenous matrix, reducing interfibrillar slippage and contributing to elasticity (Parry et al., 1978a). Type III collagen has been associated with smaller fibril diameters (Birk and Mayne, 1997) and is found in greater amounts in (weaker) immature and healing tendons and in ligaments; as the fibril distribution becomes bimodal in tendons during maturation, the collagen type changes to a clear predominance of type I. As small numbers of large-diameter fibrils can make a large contribution to tendon strength, the diameter distribution is often expressed as the mass-average diameter (MAD). The MAD is effectively the mean of the fibril diameter-area distribution as derived from the diameter-frequency distribution of a given collagen fibril population and correlates with tendon tensile strength (Parry and Craig, 1988). There are significant differences in collagen fibril diameter distributions between tendons with different functions (see Figure 13-5). In the SDFT, with conflicting requirements for strength and elasticity, both large and small diameter fibrils are prominent. Similarly, the SL contains large numbers of small diameter fibrils, but in the DDFT, most are of large diameter (Patterson-Kane et al., 1998a). Mean mass-average diameters of approximately 132 nm, 202 nm, 114 nm, and 271 nm have been reported for the SDFT core, DDFT, SL, and CDET, respectively, in young adult Thoroughbreds (Edwards et al., 2005; Patterson-Kane et al., 1997d; 1998a). MADs in the SDFT and DDFT peak at 12 to 18 months and 2 years, respectively. Crimp angle and period length in the digital flexor tendons reduce from birth until approximately age 2 years, and “mature” (hydroxylysylpyridinoline) collagen cross-links increase to almost maximal levels by age 12 to 18 months (Patterson-Kane et al., 1997b). The digital flexor tendons are, therefore, considered mature by the time horses begin to train and race. Noncollagenous matrix: Proteoglycans (PG) comprise up to 20% of solids in the matrix and are molecules that play significant roles in structural integrity and regulation of the matrix. Each PG molecule has a protein core, to which one or more sulfated polysaccharide side chains, termed glycosaminoglycans (GAGs), are attached. Small PGs have one to two GAGs, with decorin and fibromodulin being the most abundant in equine tendon (Smith and Webbon, 1996). Small PGs are thought to play an important role in the regulation of collagen fibril diameter, and interfibrillar PG bridges have been visualized in tendons and other connective tissue (Scott and Tomlinson, 1998; Watanabe et al., 2005). It has been proposed that these PGs also contribute to matrix strength and elasticity through their collagen fibril surface interactions and that they may bind various growth factors; however, the results of one recent study of human patellar tendon, some fascicles in which were treated with chondroitinase ABC to digest GAGs, suggested that proteoglycan-associated GAGs have no effect on tensile force transmission (Svensson et al., 2011). Small PGs, in general, are more abundant in regions of tendons subject largely to tensile loading (e.g., the metacarpal region of the SDFT), with greater prominence of large PGs, including aggrecan and versican, in fibrocartilaginous wrap-around regions, including the dorsal aspects of tendons traversing the palmar surface of the fetlock and plantar surface of the hock (tarsus) region. Another abundant noncollagenous molecule that has received significant research attention is cartilage oligomeric matrix protein (COMP), a five-armed disulfide-bonded calcium-binding glycoprotein with globular C-terminal domains, which is found in load-resistant tissues. C-terminal domains bind to fibrillar collagen (I, II) and collagen type IX in a zinc-dependent matter. COMP, which was first identified in cartilage, has been proposed to assist in collagen molecule alignment and fibrillogenesis in addition to providing structural integrity; in the SDFT, a positive correlation was seen between high COMP immunolabeling and the percentage of small fibrils (<60 nm) (Södersten et al., 2005). Levels of COMP in the SDFT are influenced by weightbearing; levels in the tensile region are low in neonates, reach a peak at approximately 2 years (skeletal maturity), and then rapidly decline (Smith et al., 2002b). This decline does not occur in the fetlock region of the SDFT. Highest levels of COMP are found in tendons experiencing the highest tensile loads; levels are lower in the DDFT and the CDET (Smith et al., 1997; 2002b). There is significant regional variation in the biochemical composition of tendons, in particular, between tensional and wrap-around regions. Glycosaminoglycan (GAG) concentrations are higher in the sesamoid region than the metacarpal region of the SDFT. In the adult bovine DDFT, there is higher expression of messenger ribonucleic acid (mRNA) for collagen types I and II, decorin, biglycan, and aggregan in the wrap-around region (Perez-Castro and Vogel, 1999). The equine SDFT is not homogeneous (i.e., not “biochemically blank”) at birth, which has been suggested to be caused by fetal movement in utero; however, there are significant and differential changes in the metacarpal and sesamoid regions during postnatal growth and development associated with changes in the mechanical environment (Lin et al., 2005). This essentially fibrocartilaginous differentiation in wrap-around regions has been reduced in rabbit flexor tendons by surgical translocation to eliminate compression; that is, even in adult tendons, load-modulated remodeling can occur within a short time (Gillard et al., 1979). Tendons transmit muscular forces to bones, often crossing one or more joints and enabling muscles to (1) move skeletal parts distant from their own positions and (2) coordinate contractile forces of agonist and antagonist (groups of) muscles for precision of movement. Ligaments by definition act passively to link bones and resist their distraction, limiting the motion of some joints and contributing to antigravity mechanisms (Hildebrand and Goslow, 2001). The equine digital flexor tendons and the SL deviate from these traditional definitions, as part of a unique passive apparatus. Structural and material properties: It is important to understand the meaning of terms used to describe the mechanical properties of tendons, ligaments, and other connective tissues. Tendons and ligaments stretch and narrow when subjected to tensile loads. In vitro, a tendon loaded at a constant rate elongates until rupture occurs; the resulting load-elongation curve has a characteristic sigmoidal shape, and properties of the tendon or ligament as a whole structure can be calculated from it. In the initial “toe” region, there is easy stretching in response to low loads, which has been proposed to be caused by straightening of the collagen fibril crimp, the interfibrillar sliding, or both (Nordin et al., 2001). As loading continues, the straightened fibrils are stretched, and there is a “linear” region, with progressively greater force required to produce equivalent amounts of elongation. The slope of this region is the structural stiffness of the tendon. At increasing loads, the curve becomes nonlinear, with the point at which this occurs being termed the “yield point” (although in practice this point is not always easily determined), at which time stretching and slipping of collagen fibrils and molecules begin to occur. Deformations below the yield point are fully recoverable, with the tendon returning to its original shape (elastic deformation); if loading continues, there is increasing collagen deformation with defibrillation and rupture. The ultimate load is the force at complete failure. Because tendons differ in size between individuals and anatomic sites, the load can be normalized as stress, that is, the internal transmission of the load. This is the force per unit CSA, usually expressed in kilograms per centimeters squared (kg/cm2) or megapascals (1 MPa = 10.2 kg/cm2). The resultant deformation is normalized as strain, which is a dimensionless term, defined as the percentage change in the length relative to the original “resting” length. The stress–strain curve is also sigmoidal (Figure 13-6, A), but properties calculated from it refer to the material that the tendon or ligament is composed of and not the structure as a whole. The slope of the curve in the linear region is a measure of the intrinsic stiffness of the material and is termed the “modulus of elasticity” (Young’s modulus, E). It is important not to confuse E with (1) elasticity, that is, the ability of the tendon or ligament to return completely to its original shape after the load is removed, and (2) structural stiffness (as described above). The ultimate stress and strain values, at which the tendon breaks, are independent of the size and shape of the tendon. Measurements made in vitro do not completely predict the behavior of tendons and ligaments in vivo, as these structures are not linearly elastic, that is, stress and strain do not alter in phase. They are defined as “viscoelastic,” which means that they exhibit time-dependent behavior under loading, reflecting the complex interactions between collagen fibrils, the noncollagenous matrix, and water. This can be modeled as a combination of the response of a viscous fluid and an elastic solid (spring). When strain rates are increased, the linear region of the stress–strain curve becomes steeper, that is, the tissue becomes stiffer, and the curve shifts slightly to the left. During repeated cyclical loading, however, the stress–strain curve moves to the right along the strain axis (i.e., the tendon stiffness reduces) until it reaches a steady state. This has been suggested to be caused by packing of collagen fibrils and redistribution or elimination of water from the matrix. In the creep test, under a static load below the linear region of the stress–strain curve, there is an initial rapid deformation followed by slower deformation until equilibrium is reached. Whether dynamic or static loading is applied, this is termed “preconditioning” and is relatively short-lived and eventually recoverable, with no effect on the eventual maximal load. Some energy input is lost, mostly as heat, due to internal resistance processes, and this is termed “hysteresis,” or energy dissipation. Under cyclical loading conditions, the unloading curve will be below the loading curve, with the area between the two representing the energy lost by viscous damping (see Figure 13-6, B); energy dissipation in equine tendons and ligaments is approximately 5%. Mathematical modeling predicted that the temperature of the core of the SDFT would plateau at 11°C above that of the surface at a sustained gallop due to insufficiency of the blood supply in dissipating energy lost as heat. Subsequently, peak intratendonous temperatures of up to 45°C were recorded (Wilson and Goodship, 1994); it is possible that core temperatures might be even greater under some circumstances, including extended overground exercise. Under static loading conditions in vitro, the toe region for the SDFT extends to approximately 3% to 4% strain, with the linear region from 4% to 11%, yield strains of 5% to 13%, yield stresses of 48 to 52 MPa, ultimate tensile strains between 10% and 27%, and ultimate tensile stresses of 65 to 120 MPa (Birch, 2007; Crevier et al., 1996; Dowling et al., 2002; Wilson, 1991). The ultimate tensile strain varies along the length of the SDFT, with highest values in the metacarpal region (Crevier et al., 1996). Values for the elastic modulus for the forelimb SDFT have varied from 1097 MPa to almost 2500 MPa (Batson et al., 2003; Birch, 2007; Crevier et al., 1996; Denoix, 1994). For the DDFT, an ultimate tensile stress of 89 MPa has been reported, with elastic modulus values of 613 to 1585 MPa (Denoix, 1994; Smith, 2006). For the SL, ultimate tensile strains of 10% to 12% and an ultimate tensile stress of 78 MPa have been measured, with an elastic modulus of 510 to 1100 MPa (SL body) (Birch 2007; Denoix, 1994; Smith 2006). The significant individual variation in structural and material properties of each structure suggests that certain horses are more prone to injury. For example, large differences are noted between individuals in both SDFT CSA and ultimate tensile force, with up to twofold differences in the latter (9.5–20 kN [kilonewton]) (Birch 2007; Goodship et al., 1994; Smith et al., 1994; Wilson, 1991). There are also significant differences between horses in the ultimate tensile stress (i.e., the material properties vary between animals); horses with weak tendon tissue tend to have weak bone tissue, raising the possibility that type I collagen quality may vary as in humans (Draper et al., 2004). Alternatively, however, a weaker tendon might exert less force on the attached bone either directly or by signaling earlier “switch-off” of muscular contractile force, resulting in a lesser cortical thickness, lower bone size, or even lower bone density parameters. Strains occasionally have been measured in vivo, with weightbearing values in the SDFT of ponies and horses of 2% to 4% at a walk and 4% to 10% at a trot (the latter value with a rider), and peak loads of 0.8 kN to one third of bodyweight (Lochner et al., 1980; Meershoek and Lanovaz, 2001; Platt et al., 1994; Riemersma et al., 1996; Silver et al., 1983; Stephens et al., 1989, ). Strain measurements of 11.5% and 16.6% were obtained from the SDFTs of two galloping horses (Stephens et al., 1989). Strain rates of up to 200% (i.e., the rate at which deformation occurs) have been predicted and recorded in the SDFT during the early weightbearing phase (Lochner et al., 1980; Stephens et al., 1989; Wilson, 1991). Maximum stresses of 40 to 50 MPa have been calculated to act in the SDFT, the DDFT, and other forelimb and hindlimb tendons at speeds up to 7.4 meters per second (m/s) (Biewener, 1998); Thoroughbreds gallop at speeds up to 19 m/s. Although inherent experimental errors preclude direct comparison, the in vitro and in vivo measurements of SDFT strains support the concept of a narrow mechanical safety margin, thought to be typical of energy-storing tendons (Riemersma and Schamhardt, 1985; Wilson, 1991). Additionally, it is important to consider differences in the relative loading of the flexor structures during weightbearing and interacting external factors, including the weight of the rider, surface conditions, and hoof angle and conformation. The SDFT and the SL are loaded with greater strain rates and are maximally loaded earlier in the stride compared with the DDFT at the walk and the trot (Platt et al., 1994). Strains of 3.4% to 5.9% at the walk, 5.6% at the trot, and 6.3% at the slow canter have been calculated or measured for the SL with smaller maximal stresses of 18 to 25 MPa for speeds up to 7.4 m/s than those for the flexor tendons (Biewener, 1998; Jansen et al., 1993; Keegan et al., 1992). However, it has been suggested that at the extended trot, significant loads are redistributed to the SL. Lowering of toes with respect to heels or raising of heels with respect to toes reduces loading of the DDFT and increases loading of the SDFT and the SL (Denoix, 1994; Stephens et al., 1989). Some computerized analysis of conformation has been performed, showing an increased risk of SDFT injury in racehorses that have increased metacarpophalangeal joint angle or carpal valgus conformation (Weller et al., 2006). Energy-storing versus positional tendons: Tendons such as the CDET, which position the digit prior to weightbearing (see Figure 13-1, A) and function only to transmit muscular force, are defined as “positional tendons.” Certain specialized tendons, including the SDFT of horses, also have a significant function in storing elastic energy, resulting in significant savings in muscular work during high-speed gaits, with important biomechanical and injury-risk implications central to equine evolution and survival. This concept was first proven in kangaroos hopping at high speeds, their gastrocnemius and plantaris tendons being obvious examples of such “biologic springs”; it was calculated that almost 40% of the positive and negative work performed during hopping was accounted for by tendon stretching and subsequent elastic recoil (Alexander and Vernon, 1975). As the horse bears weight, extension of the metacarpophalangeal joint stretches the SDFT, the DDFT, and the SL, including the accessory ligaments of the SDFT and the DDFT, storing kinetic and potential energy as elastic energy to be released during the subsequent propulsion phase. Proximal limb joints, including the elbow and the shoulder, may contribute to this elastic energy storage and release (Clayton et al., 1998). The percentage recovery of mechanical work by tendon elastic energy savings at a gallop has been estimated at 36% (7.4 m/s) (Biewener, 1998). Because of their dissimilar functions, the SDFT and CDET experience different mechanical environments; the CDET is not a load-bearing tendon and is thought to experience maximum strains of approximately 2.5% (Birch et al., 2008b). The SDFT must be strong enough to deal with the high locomotor and gravitational forces it experiences, but must be able to stretch significantly to store sufficient amounts of energy. Although some researchers have claimed that tendons with different functions do not differ in their mechanical properties, there is clear evidence from in vitro studies of significant differences between the energy-storing SDFT and the positional CDET of horses. Although as a structure the SDFT has a larger CSA and, therefore, fails at higher loads, as a material, it is able to stretch more to store elastic energy (Batson et al., 2003). In two studies of tendons from adult horses, the SDFT had a significantly higher CSA, structural stiffness, and failure load compared with the CDET which had no difference in ultimate stress (Batson et al., 2003; Birch, 2007). However, the failure strains were higher and the mean elastic moduli (1086 ± 261 MPa, per Batson et al., 2003; 970.8 ± 60.4 MPa, per Birch, 2007) lower in the SDFT compared with the CDET (1586 ± 279 MPa, per Batson et al., 2003; 1236.3 ± 209.6 MPa, per Birch, 2007) in both studies. Greater material stiffness is an advantage for the CDET as it functions only to transfer muscle force for accurate limb movement and positioning. These results were supported by measurements of the SL, which is also thought to store elastic strain energy during locomotion. The SL from the same horses in the more recent study had an elastic modulus (643 ± 130 MPa) significantly lower than that of either the SDFT or CDET (Birch, 2007; Smith 2006). Tenocytes are fibroblastic cells within fascicles responsible for the synthesis, maintenance, and degradation of the matrix. Surprisingly, most research on tenocytes has been conducted only in the last 20 years; prior to that most tendon research focused on the matrix. As a result, tenocytes are still a poorly defined population with no generally agreed specific markers. A very small number of genes, including tenomodulin, are expressed in tendons and ligaments but not in other musculoskeletal tissues of rats (Archambault et al., 2006), and scleraxis is a specific marker for tendon and ligament during embryogenesis. Tenocytes were traditionally thought to be relatively inert, but recent studies in human athletes have demonstrated by using tendon biopsies rapid upregulation of collagen synthesis following single bouts of exercise (Magnusson et al., 2007). They are arranged in interlinked networks with connection of their cytoplasmic processes by both gap junctions (GJ) and adherens junctions (AJ) (McNeilly et al., 1996; Ralphs et al., 2002). Tenocytes are immediately surrounded by small amounts of specialized (pericellular) matrix containing type VI collagen, versican, and fibrillin-2 (Ritty et al., 2003). Tenocytes comprise a very small proportion of the tissue, with cellularity in the young adult SDFT (2–7 years of age) of only 400 cells/mm2, dropping to less than 300 cells/mm2 in older horses (Stanley et al., 2007). They are capable of oxidative energy metabolism, suggesting that they may be susceptible to hypoxia (Birch et al., 1997a). In addition to synthesizing matrix components, tenocytes also produce enzymes involved in matrix degradation, much of which is thought to occur extracellularly. These include MMPs, ADAM (A distegrin and metalloproteinases) and ADAMTS (A distegrin and metalloproteinases with thrombospondin motifs). Actions of the proteinases are inhibited by tissue inhibitors of metalloproteinases (TIMPs). There are also fibroblastic cells in the paratenon, epitenon, and endotenon, the roles of which are not certain. It is known that transforming growth factor-β (TGF-β1) is found in only the endotenon in the adult SDFT (Cauvin et al., 1998). The existence of stem cells in tendon tissue was first suggested by isolation of cell lines with pluripotential behavior, with subsequent direct demonstration within mouse and human tendon tissue of a minor population of cells exhibiting universal stem cell characteristics that are now termed tendon stem or progenitor cells (TSPCs) or tendon-derived stem cells (TDSCs) (Bi et al., 2007; Lui and Chan, 2011; Salingcarnboriboon et al., 2003). It has been suggested, but not demonstrated, that TDSCs have a perivascular location within the endotenon (Lui and Chan, 2011). However, other authors, on the basis of studies of mouse tendon tissue, claim that the TDSCs are within the extracellular matrix of fascicles and that the stem cell “niche” is determined by the nature of that matrix (Bi et al., 2007). Adult equine tendon-derived cells have some degree of multipotential differentiation capability, but it is less than that of bone marrow–derived mesenchymal stem cells (Strassburg et al., 2006). Identification of TDSCs in situ in equine tendon has not yet been achieved. Tenocyte types: Histologically, three tenocyte “types” have been defined in equine tendon on the basis of their nuclear morphology. It is not known if these are true functional cell types and if they are the equivalents of osteocytes, osteoblasts, and osteoclasts in bone. Type 1 tenocytes have thin spindle-shaped nuclei, whereas those of type 2 cells are more rounded and cigar-shaped nuclei (Figure 13-7, A). In the SL, there are larger numbers of cells than in the SDFT, with predominance of type 2 nuclei (Smith and Webbon, 1996) (see Figure 13-7, A and B). Three dimensionally, type 1 and 2 tenocyte nuclei are slightly flattened ovoids; the range of nuclear widths is greater for the type 2 cells (Doube, 2001, Stanley, 2005). Type 3 tenocytes are found in wrap-around regions and have a more chondrogenic phenotype (see Figure 13-7, B). Type 3 tenocytes express significantly less gap junction proteins or none at all (Ralphs et al., 1998). In other species, tendon cells equivalent to type 2 and type 1 cells in horses have been referred to as “tenoblasts” and “tenocytes,” respectively (Chuen et al., 2004; Ippolito et al., 1980; Kannus, 2000). It has been proposed that as they age, the tenoblasts become elongated and transformed into tenocytes, although there is only indirect evidence for this (Ippolito et al., 1980; Nakagawa et al., 1994). Age-related increases in the proportions of type 1 tenocytes and in nuclear lengths have been measured in the equine SDFT and CDET, along with reductions in type 2 cells and overall cellularity (Stanley, 2005). Type 2 tenocytes (tenoblasts) do appear to be more synthetically active compared with type 1 cells; in nonequine tendons the type 2 tenocytes have been shown to contain more extensive synthetic organelles, express higher levels of certain proteins including type I procollagen and matrix metalloproteinase 1 (MMP1), and have higher proliferative and apoptotic indices (Chuen et al., 2004; Ippolito et al., 1980). Tenocyte network: The ability of tenocytes to sense and respond to load is central to maintenance of the matrix. Detection of load signals is thought to occur through deformation of the cytoplasmic membrane, the cytoskeleton, or both, and growth factors may be required for this process (Banes et al., 1995; Wang, 2006). Tenocytes are attached to extracellular matrix proteins, including collagen and fibronectin by integrins, these being transmembrane mechanoreceptors with α- and β-subunits (Wang et al., 1993). Adhesion to collagen via α1β1 integrins, which preferentially bind to nonfibrillar collagen types, promote cell growth, and inhibit collagen synthesis. Adhesion via α2β1 integrins, which preferentially bind to fibrillar collagens (including type I), inhibit the cell cycle, and promote collagen synthesis and degradation. Tenocytes attach more strongly together in response to mechanical loading, with upregulation of n-cadherin (an AJ component) and vinculin, an actin-binding protein that links both integrin molecules and AJ components to the cytoskeleton, and assemblage of more of their actin fibers into stress fibers with tropomyosin (Ralphs et al., 2002). Tenocytes have been suggested to establish an internal cytoskeletal tension through their extracellular interactions, resulting in a mechanostat set point that determines whether cells will respond in an anabolic manner or a catabolic manner to mechanical loading (Lavagnino and Arnoczky, 2005). The nucleus itself may be deformed, as demonstrated in tendons in vitro by using confocal laser scanning microscopy, and may also play a significant role in the signal transduction pathway (Arnoczky et al., 2002). Additionally, tenocytes are now known to express a single primary cilium, this being a microtubule-based sensory organelle that projects into the extracellular matrix parallel to the longitudinal axis of the tendon; the deflection of cilia in response to tensile loading that has been demonstrated experimentally supports the concept that these structures are involved in mechanotransduction (Donnelly et al., 2010, Lavagnino et al., 2011). Tenocytes are also linked into coordinated networks by GJ, that allow metabolites, ions, and small molecules <1 kilodalton (kDa) to pass directly between the cytoplasm of neighboring cells. Each GJ comprises two annular hemichannels that are embedded in the cytoplasmic membranes of apposing cells and dock to form a tightly sealed channel. Each hemichannel comprises connexins, these being transmembrane proteins of which 21 genes are known in the human genome. Connexins 43 and 32 (Cx43, Cx32) have been identified in equine and nonequine tendons (McNeilly et al., 1996; Stanley et al., 2007; Figure 13-8). Both isoforms may be found in the GJ linking tenocytes in the same row, but only Cx43 forms the GJ channels responsible for lateral links (McNeilly et al., 1996). Calcium wave propagation is blocked in avian tendon cells if they are cultured in the presence of GJ chemical blockers or connexin antibodies (Boitano et al., 1992). GJ blockade also prevents upregulation of collagen synthesis by tendon cells in response to cyclic loading (Banes et al., 1995, 1999). There is some evidence from a study of avian tenocytes treated with antisense oligodeoxynucleotides that Cx43 and Cx32 may differentially modulate collagen synthesis, with Cx43 being inhibitory and Cx32 stimulatory (Waggett et al., 2006). In wrap-around regions of tendons, where type 3 tenocytes predominate and the expression of GJ is low to nonexistent, coordination of tenocyte behavior must occur by other means (e.g., via indirect cytokine and growth factor signaling) (Ralphs et al., 1998). FIGURE 13-8 Longitudinal cryosections from the superficial digital flexor tendon and common digital extensor tendon of a fetus, a foal, and an old horse, immunolabeled for connexin 43 (green plaques). Tenocyte nuclei are counterstained with propidium iodide (red). Immature tendons were significantly more cellular, and greater expression of the gap junction protein per cell was measured in the fetal structures. Bar = 80 μm. (From Stanley RL, et al.: Gap junction protein expression and cellularity: comparison of immature and adult equine digital tendons, J Anat 211:325, 2007, with permission). Tendon-specific and age-specific differences in tenocyte networks: Significant changes in cellularity, tenocyte morphology, and tenocyte activity with both maturation and aging have been documented in equine digital tendons, with marked differences between energy-storing tendons and positional tendons. In the adult horse, the SDFT has a significantly higher cellularity than the CDET (Stanley, 2005; Stanley et al., 2007; Young et al., 2009). However, there is increasing evidence that these more numerous SDFT tenocytes are less active. In the SDFT of adult Thoroughbreds, age-related increases in collagen-linked fluorescence were documented, but these did not occur in the CDET; this suggested, but did not directly prove, that the turnover of collagen reduces with age in the SDFT (Batson et al., 2003). Subsequent analysis of aspartic acid racemization of collagen in horses 4 to 30 years of age indicated mean collagen half-life values of 198 years and 34 years for the SDFT and the CDET, respectively (Thorpe et al., 2010). Analysis of tissue showed lower relative levels of mRNA for type I and III collagen and for MMPs per cell in the SDFT compared with the CDET (Birch et al., 2008b). There were high levels of the cross-linked carboxyterminal telopeptide of type I collagen (ICTP) (a marker of collagen synthesis) and lower levels of tissue fluorescence in the CDET; that is, this was further support for the hypothesis that tenocytes are less active in the SDFT than in the CDET of the adult horse, with reduction or inhibition of collagen turnover. In immature horses, however, the SDFT tenocytes are significantly more numerous and appear to be more active. This is not surprising, as increases in total bodyweight are very rapid in foals, particularly within the first 90 days of birth (Hintz et al., 1979). The SDFT cross-sectional area increases more than twofold between 50 and 365 days, necessitating significant synthetic cellular activity (Kasashima et al., 2002). In histomorphometric and immunofluorescent labeling studies, the density of tenocytes was greatest in tendons of late-term equine fetuses and foals, with higher expression of GJ proteins, and type I and III collagens in immature horses than in adults for the SDFT (Stanley et al., 2007; Young et al., 2009) (see Figure 13-8). In the CDET, there was actually an increase in Cx43 and collagen expression with maturation, exceeding levels in the more cellular SDFT (Young et al., 2009). Cultured immature SDFT-derived cells produced more collagen and COMP compared with adult cells in another study, with no such maturational changes in CDET cells (Goodman et al., 2004). When gap junction permeability was studied in cell culture, there was a reduction with maturation in the SDFT but not in the CDET (Young et al, 2009). Additionally, significantly higher levels of TGF-β (a potent stimulator of type I collagen synthesis) were measured in young equine tendons compared with adult structures (Cauvin et al., 1998). It has been suggested that despite the theoretically higher demand for matrix renewal compared with positional structures, energy-storing tendons, including the SDFT, specifically “switch off” synthetic cellular machinery once maturity is reached to maintain the matrix within narrow optimal limits of elasticity and stiffness (Smith et al., 2002a). This has also been measured in the bovine DDFT, with no expression of mRNA for collagen or PG in the tensional region of the adult structure (Perez-Castro and Vogel, 1999). The mechanisms by which this cellular switchoff occurs are not understood; however, it is a key consideration in the response of the SDFT to imposition of increased exercise through training, racing, or other athletic activity. There is very little information on the effects of exercise on tendons in any species, and much of it is inconsistent, as opposed to the well-documented changes that occur in skeletal muscle tissue and bone. To some extent, this can be attributed to the lack of a suitable animal model in which tendons can be isolated and differentially loaded, e.g., as for the functionally isolated avian ulna model used for bone research (Rubin and Lanyon, 1984b). Additionally, it is important to consider whether the changes that have been documented have any adaptive significance. For example, changes in tendon stiffness will not necessarily maximize elastic energy storage if forces applied to the tendon are not also substantially altered (Buchanan and Marsh, 2002). Moreover, increases in strength or stiffness may not necessarily increase resistance to fatigue damage. A number of controlled exercise studies using foals or young adult Thoroughbreds have been performed in an attempt to determine effects of specific training regimens on tendon structure and composition (Table 13-1 and Table 13-2); these studies will be discussed below. Tenocytes constantly repair small amounts of damage to the matrix under normal circumstances; otherwise, all tendons would weaken and rupture (Ker, 2002). It has been suggested that damage is actually the trigger for tenocytes to produce “better” material to resist fatigue (Ker et al., 2000). However, excessive and repetitive loading may cause levels and types of subclinical matrix damage (microdamage) that cannot be repaired by the tenocytes for various reasons, including insufficient rest periods between episodes and simple overwhelming of cellular capacity due to a high frequency or nonuniformity of overstrain. Tenocytes could potentially be damaged themselves, either dying or responding with inappropriate synthetic or degradative activity. Prolonged cyclical loading of equine SDFT explants at 5% strain (1hertz [Hz] for 24 hours) resulted in significantly reduced matrix strength, a process that required viable cells and MMP activity (Dudhia et al., 2007). Experimental work with cultured human tenocytes has suggested that upregulation of MMP expression is associated with high levels of strain (Yang et al., 2005). Some researchers have hypothesized the opposite, that is, the occurrence of isolated fibril rupture near the end of the linear region of the stress–strain curve, which results in mechanobiologic understimulation of tenocytes in the region of microdamage with resultant upregulation of expression of various MMPs (Arnoczky et al., 2007). Degradation of the pericellular matrix would further compromise cell–matrix interactions and signaling. Mechanical stress–deprivation has also been shown to upregulate tenocyte apoptosis in tendon explants (Egerbacher et al., 2008). Temperatures of up to 45°C as documented in the SDFT core of galloping horses could be an important factor. Although high survival of tendon-derived cells after 10 minutes at that temperature was documented in one study (91% ± 4%) (Birch et al., 1997b), those cells were in suspension, a situation in which cells are known to have greater heat resistance and are not linked by the GJ. Subsequent work using SDFT-derived cellular monolayers indicated significant cell loss following a 10-minute period, during which the temperature was increased to 45°C (Burrows et al., 2008). Loss of even small numbers of tenocytes in this lowly cellular tissue could have significant consequences in terms of collagen synthesis and repair potential; however, it has not been directly demonstrated that apoptotic cells occur in equine tendon that is degenerate but not clinically injured. Given that the SDFT has narrow mechanical safety margins and that the number of tenocytes and their level of synthetic activity and matrix turnover in the adult tendon are low under normal circumstances, (1) this energy-storing tendon may occasionally or even frequently be loaded into the “yield” phase of the stress–strain curve during exercise, resulting in microdamage; and (2) the risk of an imbalance between cellular repair and matrix microdamage is likely to be high. A “tendonosis cycle” has been proposed, with accumulation of damage that weakens the matrix and predisposes to further insult. The ultimate result in some individuals will be a partial or complete rupture during normal athletic activity. It is generally agreed that a large proportion of tendon injuries in humans occur in this way, as opposed to rupture of a healthy tendon under extreme physical circumstances, and there is also significant evidence for exercise-induced degenerative change (i.e., “overuse”) in the SDFT of significant numbers of horses (Goodship et al., 1994; Józsa and Kannus, 1997; Smith et al., 1999). This concept is supported by results of epidemiologic studies, showing an increased risk of SDFT and suspensory apparatus injury in older horses that have theoretically experienced greater numbers of loading cycles at high speed (Avella et al., 2009; Perkins et al., 2005; Williams et al., 2001). Results from several studies have suggested that exercise and age have synergistic effects that can be difficult to separate in uncontrolled circumstances. In one study of feral horses, that is, those experiencing “natural” levels of activity, mean crimp angles in the core of the mid-metacarpal SDFT reduced with age such that they were lower than those in the periphery of the tendon cross-section in horses 10 years of age and older (Patterson-Kane et al., 1997a). This normally age-related change was found to be accelerated in racehorses, and in the horses in the Bristol study that were trained on a high-speed treadmill for 18 months, that is, in animals experiencing a greater number of loading cycles earlier in life (Patterson-Kane et al., 1997c, 1998b) (Table 13-3). As fibrils with lower crimp angles will fail at lower levels of strain, the exercise-induced changes in crimp morphology were interpreted as microdamage. TABLE 13–3 *significantly greater than the corresponding value for the exercised group (p<0.05) (Patterson-Kane et al. 1997d). Similarly, collagen fibril diameters have been previously shown to reduce with aging in the equine SDFT and SL (Parry et al., 1978b). In the 18-month Bristol study, there was a reduction in the MAD in the SDFT core of the exercised horses, which did not occur in equivalent segments of the DDFT, the SL, or the CDET (Edwards et al., 2005; Patterson-Kane et al., 1997d; 1998a) (see Table 13-3). As there was no evidence of synthesis of new collagen in the SDFT core in the form of detectable levels of immature collagen cross-links or alterations in levels of tissue fluorescence, it was suggested that there had been breakdown of larger fibrils (Birch et al., 2008a; Patterson-Kane et al., 1997d; Smith et al., 2002); fibrils from rat tendons were noted to split longitudinally in response to high mechanical loads in in vitro experiments (Torp et al., 1975). Additionally, in the same site, there was exercise-related acceleration of normal maturational loss of COMP and a reduction in GAG content (Birch et al., 2008a; Smith et al., 1999). As SDFT COMP concentrations for 2-year-old horses have been correlated with ultimate tensile stress, modulus of elasticity, and tendon stiffness, this change in the 18-month Bristol study was interpreted as being one that would predispose to further injury (Smith et al., 1999; 2002a). Increased amounts of (weaker) type III collagen have been measured in the SDFT core in older horses relative to the periphery of the cross-section (Birch et al., 1999a). Levels of type I collagen degradation markers, that is, collagenase-generated neoepitope and cross-linked telopeptide of type I collagen have also been determined to increase with age in this tendon (Thorpe et al. 2010). In areas of red discoloration of the SDFT core in other horses (Figure 13-9), there were also increases in the proportion of type III collagen in addition to increased total sulfated GAG content and a decrease in collagen-linked fluorescence relative to the periphery, that is, an increase in collagen turnover and deposition of an abnormal collagen type (Birch et al., 1998). Such changes were previously interpreted as subclinical damage. However, in vitro analysis has revealed that tendons with red core lesions are enlarged and contain central hypoechoic areas; that is, this is more likely to be a reparative response than preclinical microdamage (Smith, 2003).
Tendon, ligament, bone, and cartilage: Anatomy, physiology, and adaptations to exercise and training
Tendons and ligaments
Tendon and ligament anatomy and function
Hierarchical structure, microanatomy, and matrix composition
Biomechanical properties of digital tendons and ligaments
Cellular basis of tendon and ligament matrix maintenance and response to exercise
Functional adaptation of tendon
Evidence of exercise-induced microdamage
< div class='tao-gold-member'>
Stay updated, free articles. Join our Telegram channel
Full access? Get Clinical Tree
Tendon, ligament, bone, and cartilage: Anatomy, physiology, and adaptations to exercise and training
Only gold members can continue reading. Log In or Register a > to continue
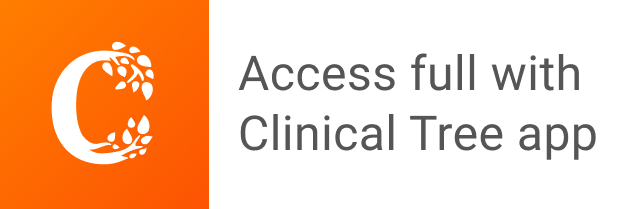