Helen L. Birch, Charlotte Sinclair, Allen E. Goodship and Roger K.W. Smith Tendons and ligaments are soft connective tissues that form part of the skeletal system. The distinction between tendons and ligaments is an anatomic one; tendons join muscle to bone whereas ligaments join bone to bone. In terms of their biomechanical, ultra-structural and matrix compositional properties however, the two structures are similar and investigations have revealed an overlap of properties resulting in a continuum from ‘pure’ tendon to ‘pure’ ligament.1 In connecting muscle to bone, tendons have been considered rather inert force-transmitting structures that are involved in the movement of joints. While this positioning function is important, certain tendons in cursorial animals have developed another more specialized role acting as springs to store energy for efficient locomotion.2 This is particularly true in the horse, where the tendinous structures on the palmar aspect of the metacarpal region, particularly the superficial digital flexor tendon (SDFT) and the musculus interosseous medius tendon (suspensory ligament; SL), act to support the hyper-extended metacarpophalangeal joint during weight bearing (Fig. 9.1), releasing energy when the limb is protracted. Hence, at fast gaits, the horse effectively bounces up and down on springs, similar to a child’s pogo stick thereby decreasing the energy required for high speed locomotion.3 As further modifications to this role, the digital flexor tendons have accessory ligaments (AL), which attach the tendon directly to bone providing a direct bone-to-bone tendinous connection (Fig. 9.2) when under full weight bearing so further reducing the requirement for muscular exertion. It would be expected that these energy storing tendons have mechanical characteristics suited for this function as a spring, while tendons with a less specialized positional role (e.g. human digital flexor tendons and the common digital extensor tendon (CDET) of the horse) require different characteristics. Indeed up to a two-fold difference in material stiffness has been found between the equine SDFT and CDET indicating differences in molecular composition and organization.4 Strain-induced injury of the tendons and ligaments is the most common orthopedic injury in athletic animals, be they equine or human. Increased risk of certain injuries has been associated with both discipline and competition. For example, in elite event horses (intermediate level and above) there is a significantly increased risk of SDFT injuries; in elite show jumpers (grade A and above) there is a significantly increased risk of both SDFT and DDFT injuries; in elite (advanced-medium and above) and non-elite dressage horses there is an increased risk of hindlimb suspensory injury.5 Epidemiologic surveys of race horse injuries sustained at UK race tracks between 1996 and 1998 showed that of all limb injuries (82% of all incidents), almost one-half (46%) were due to flexor tendon and/or SL injuries.6 As observed clinically, this study confirmed that these injuries were much more common in older horses racing over jumps (steeplechasers or hurdlers) than in younger race horses racing on the flat. Furthermore, the data from these flat races correlated well with previous data published from studies in the USA (0.760 per 1000 starts in 1992).7 However, this epidemiologic data, being obtained from only those injuries occurring during racing, represents only the tip of the iceberg. Evaluation of a cohort of National Hunt racehorses in training revealed a prevalence of SDFT pathology detected using ultrasonography of 24% (n = 148), with a non-significant variation between yards of 10–40%.8 Older horses had a significantly higher prevalence of SDFT pathology compared to younger horses, and horses with tendinopathy were more likely to suffer an acute injury compared to horses with no evidence of pathology. The rate of tendon and ligament injury in 1223 National Hunt racehorses in training was shown to be 1.9/100 horse months, however this varied significantly between trainers.9 Of these injuries 89% were SDFT injuries with the remainder being suspensory injuries. Interestingly, the incidence of Achilles tendinopathy in man has doubled in incidence in Europe in the last 10 years, believed to be associated with greater levels of exercise and increased longevity.10 SDFT injury has been shown to account for the cause of 14% of racehorse retirements in Hong Kong and was the single most common reason for retirement.11 It is interesting that ponies rarely suffer from superficial digital flexor tendinopathy, although they do have a relatively high incidence of desmitis of the accessory ligament of the DDFT. A single digit has resulted in a simplified tendon and ligament anatomy (see Fig. 9.2). The distal fore and hind limb has an arrangement of three structures on the palmar aspect; the SDFT and DDFT with their associated accessory ligaments, and the SL which serve to support the hyperextended metacarpo- or metatarsophalangeal joint. On the dorsal aspect are two or three digital extensor tendons, which function only to extend the distal limb during protraction. Hence the loads experienced by these tendon groups are very different; the extensor tendons experience only low loads while the palmar tissues are subjected to high loads of weight bearing (7–10kN on the SDFT).12 Despite large amounts of connective tissue, the muscle belly of the SDFT is not redundant although its proposed role is different from the classical function of flexing the limb. The muscle fibers are short (2–6 mm) and not capable of contributing to limb flexion or ‘tuning’ the spring-like mechanism.3 However it has been shown that the SDF muscle belly is capable of dampening high-frequency vibrations in the limb, which are generated when the foot is placed on the ground at high speeds.3 These high frequency vibrations could cause fatigue damage to the tendon. The blood vessels are an important supplier of nutrients for the tendon cells. Intra-tendinous blood supply emanates from the musculo-tendinous junction, the osseous insertion (enthesis) and blood vessels entering the tendon via mesotenon attachments within tendon sheaths or the paratenon. Another potential source of nutrients is the synovial fluid within the tendon sheath. The relevant importance of these components depends on the tendon and tendon site. For the metacarpal region of the SDFT, a study has suggested that the intra-tendinous supply from the myotendinous junction and enthesis is the most important, as necrosis was only achieved by ligation of the intratendinous blood vessels whereas stripping the paratenon had no effect.13 The blood flow in the SDFT has been recorded between 1 and 2 mL/min/100g14, 15 which is of similar magnitude to that within resting skeletal muscle.16 Blood flow has been shown to increase two-fold with exercise,15 although this can be delayed in horses that have not been trained. Injury caused an even greater increase in blood flow (>300%), which has been recorded in both limbs of a horse with clinically unilateral tendinitis, suggesting bilateral nature of tendon disease. Tendon cells are known as tenocytes and these reside between the parallel aligned collagen fibers. Tenocytes are unlikely to be a uniform population of cells because they differ considerably in nuclear morphology on light microscopy and when grown in culture. Previous descriptions have described three types based on nuclear morphology17, 18 although a fourth type is evident within the endotenon tissue (see Fig. 9.3 and Table 9.1), and in other species, the synovial cells lining the outside of the tendon are differentiated from those within the tendon.19 Table 9.1 Types of cells within tendon and ligament based on nuclear morphology and location. Unlike bone, the function of these cell types is not known but their location, morphology and presence in young or adult tendon suggest functions which are activity based and these are reflected in the type classification (Table 9.1). A study on SDFT and CDET tissue sections has shown that immature horses have tenocytes with a rounder nuclei than adult horses and a greater tenocyte density than the adult horses.18 This study also found that tenocyte density was higher in the SDFT than the CDET. The tenoctyes are responsible for synthesizing and degrading the matrix components and gene expression studies on equine tendon tissue have shown a pattern of expression that reflects the composition of the tendon tissue.20 The actual synthetic activity of these different cell types is unknown, however the turnover of the non-collagenous component is much more rapid than the collagen component21 in equine tendons supporting the theory that plumper cell nuclei, such as those seen in the endotenon, are more active than the thin elongated nuclei located between the collagen fibers. Work performed on laboratory animals22 and equine tendons23 has shown that tenocytes have a large number of cytoplasmic extensions (Fig. 9.4), which connect to neighboring cells via gap junctions. Gap junctions allow the passage of small molecules to move between the cytoplasm of neighboring cells. The transmembrane proteins forming these channels are known as connexins (Cx) of which there are different forms. Connexin 32 (Cx32) and Connexin 43 (Cx 43) in equine tendon have been shown to be location specific, with Cx32 occurring only between cells in the same row and Cx43 occurring between cells in the same row and adjacent rows.23 This three-dimensional network provides an efficient system for mechanotransduction, similar to that occurring between osteocytes in bone. Following the surge of interest in stem cell therapy, tendon has been shown to possess an innate population of progenitor cells.24 These progenitor cells have stem cell characteristics of clonogenicity, multipotency and self-renewal capacity and reside in a unique niche organized by the proteoglycans biglycan and fibromodulin. More recently tendon progenitor cells have been isolated from human25 and equine26 tendons. As the cellular component of tendon is small, the functional properties of tendon rely on the extracellular matrix. This is determined, in turn, by both the composition and, equally importantly, the arrangement of these proteins within the matrix. It is constructed from a series of increasingly sized subunits into a hierarchic arrangement (Fig. 9.5). Tendon is predominantly composed of water, which makes-up approximately two-thirds of the weight of the tissue. The presence of this water is fundamental to maintaining the elasticity of the tissue because dehydration results in an increase in stiffness and tendons containing less water tend to be stiffer.27 Tendon has been shown to exhibit strain-induced flow of ionized fluids (streaming potentials) when subjected to mechanical stress.28 These fluid movements could provide a mechanism for mechanotransduction, whereby mechanical forces on the tendon influence the metabolic activity of the tenocytes. Furthermore, it has recently been shown that the change in electrical resistance is proportional to tendon strain leading to the suggestion that tendon electric properties could be used as an in vivo strain sensor.29 The remaining third of the content of tendon (the dry weight) is predominantly composed of type I collagen. This protein is a major component of all connective tissues. Each type I collagen molecule is assembled within the golgi apparatus of the cell from two α1(I) polypeptide chains and one α2(I) polypeptide chain, forming a right-handed triple super-helix. The initial product is a procollagen molecule with non-helical N and C terminal extensions (propeptides). These individual collagen molecules are assembled by cleavage of their propeptides at the N and C terminal ends by specific N- and C- proteinases, either after secretion into the immediate pericellular environment30 or intracellularly.31 This results in a tropocollagen molecule that is 285 nm long and 1.4 nm wide. These molecules are then assembled into collagen fibrils in a highly organized fashion, each collagen molecule staggered relative to its neighbor by a quarter length. This results in a gap and overlap region (D-period) that is responsible for giving the banded pattern seen in electron microscopy. Five collagen molecules make up a subunit of the collagen fibril (Fig. 9.6). Although collagen molecules will spontaneously self-assemble, other proteins play a role in orchestrating this assembly (see below). Collagen molecules are stabilized within the fibril by the formation of covalent cross-links between lysine and hydroxylysine residues mediated by the enzyme lysyl oxidase.32 Lysyl oxidase converts lysine and hydroxylysine residues in the telopeptide region into aldehydes. Lysine aldehydes and hydroxylysine aldehydes undergo a spontaneous reaction with lysine or hydroxylysine residues in the helical region of neighbouring molecules to form the immature bivalent cross-links. These immature cross-links undergo further spontaneous reactions to form mature trivalent pyridinoline and pyrrole cross-links. The type of crosslink formed depends on the degree of lysine hydroxylation in the helical part of the molecule and non-helical telopeptide region. Extensive hydroxylation of lysine residues throughout the collagen molecule results in the formation of hydroxylysyl-pyridinoline (HL-Pyr). In the equine SDFT, HL-Pyr is the predominant lysyl oxidase mediated mature crosslink.33 In tissues where less hydroxylation of the helical or telopeptide lysine residues occurs, the mature cross-links lysyl-pyridinoline (L-Pyr) and pyrrole form, respectively. Proteoglycans consist of a central protein core with O-linked glycosaminoglycan side-chains. The extracellular matrix proteoglycans can be divided into two classes; the large proteoglycans (such as aggrecan, the large proteoglycan of cartilage, and the soft tissue equivalent, versican) and the small leucine-rich proteoglycans (such as decorin, biglycan, fibromodulin, lumican and keratocan) all of which are present in tendon.34 The large proteoglycans have numerous highly sulfated glycosaminoglycan side-chains, which hold water and therefore are present where the tendon has to resist compression. Aggrecan is also present in the tensional region of tendon however there appear to be differences in the GAG side chains suggesting a different physiological function of aggrecan in this region.34 The small proteoglycans are closely associated with the collagen fibrils and are believed to regulate collagen fibril diameters. Knock-out of the decorin gene in mice resulted in large, irregular-sized collagen fibrils believed to be caused by un-regulated lateral fusion and this was associated with weak and fragile skin.35 A more recent study has shown that reduced decorin increases the material stiffness of patellar tendon in mice but does not change fibril diameters suggesting that decorin influences mechanical properties in a way that cannot be explained completely by collagen fibrillogenesis.36 Fibromodulin knock-out mice showed paradoxically smaller collagen fibrils but also a compensatory increase in another small proteoglycan, lumican, which binds to the collagen molecule at the same site to fibromodulin37, demonstrating that many of these small proteoglycans can ‘cross-function’. During growth of the digital flexor tendons, one of the most abundant proteins is the glycoprotein cartilage oligomeric matrix protein (COMP). There are only low levels of this protein in tendon at birth but it accumulates during growth within the digital flexor tendons with the highest levels achieved in the tensional region of the SDFT at skeletal maturity in the horse (at approximately two years of age) of approximately 10 mg/g wet weight.38 This equates to approximately 30 mg/g dry weight or approximately 1% of the wet weight. After the period of growth, however, levels of COMP in the tensional, but not the compressed, region of the SDFT fall. COMP binds to fibrillar collagens (including type I) via a zinc-dependent mechanism.39 Each COMP molecule can bind to five collagen molecules possibly for assembly into a collagen fibril, once the fibril is formed COMP is displaced. Recent studies have shown that COMP can accelerate collagen fibrillogenesis in vitro and it is possible that this protein acts more as an organizational molecule rather than in a structural role.40 Correspondingly, the highest levels are present during the growth of the tendon when matrix is being synthesized and levels fall after skeletal maturity when there is little change in the structural properties of the tendon. It has been suggested that the more COMP synthesized during growth, the stronger the resulting tendon and this is supported by the finding that mechanical properties of SDFT at skeletal maturity demonstrate a significant positive relationship to COMP levels.41 Small amounts of the protein elastin and other components of elastic fibres can be found in varying amounts in tendon and ligament and these appear to be localized to between the collagen fascicles.42, 43 The glycoprotein lubricin has also been found in tendon in an inter-fascicular location where it facilitates sliding between fascicles44, 45 and on the surface of tendon in both intra- and extra-synovial locations.46 Other proteins expressed by tendon cells include thrombospondin 4, tenascin-C, and tenomodulin which have been used to identify a tendon cell phenotype.47 Collagen fibrils are assembled in a longitudinally oriented pattern into increasingly larger subunits, which ultimately form the whole tendon (Fig. 9.5). The precise arrangement of at each sub level of the hierarchal arrangement has an influence on the mechanical properties of the final tendon structure and is therefore vital for tendon function. The quarter staggered arrangement of collagen molecules during fibrillogenesis results in micro-fibril structures which group together to form fibrils. The collagen fibrils in tendon follow an essentially straight path, as opposed to the right-handed helical course taken by fibrils in tissues such as skin.48 However scanning electron microscopy studies have shown that collagen fibrils in tendon show ‘knots’ or fibrillar crimps. These ‘knots’ are formed as the fibrils first twist leftwards, changing their plane of running, and then bent sharply also changing the direction of coursing.49 The fibrillar crimp functions as a biological hinge opening when tensional load is applied and recoiling when load is removed.49 Transverse sections viewed under an electron microscope show the collagen fibrils as electron dense circular structures of varying sizes. The distribution of collagen fibril diameters can be determined from these sections, as well as assessment of the mass average diameter, which takes into account the relative proportion of the area taken up by different-sized fibrils. The adult SDFT has a bimodal distribution of fibrils with large numbers of small fibrils and lower numbers of large-diameter fibrils50 (Fig. 9.7). Furthermore, there are differences between tendons, with the DDFT having fewer small-diameter and greater numbers of large-diameter fibrils.51 The size of the collagen fibrils shows a significant correlation with the stiffness of the tendon tissue; larger fibrils resulting in a stiffer tissue.27 Collagen fibrils group together to form fibers which have a diameter of approximately 10 µm. The fibrillar crimps align resulting in the waveform seen in longitudinal tendon histologic sections at the fiber level (Fig. 9.3). When viewed under polarized light, crimp results in alternating light and dark bands. Crimp can be described by its angle and length and these change as the animal ages (see below). Collagen fibres group together to form sub-fascicles and sub-fascicles assemble to form fascicles which finally come together to form the whole tendon (Fig. 9.8). The tendon is surrounded by a thin connective tissue membrane known as the epitenon, which continues into the tendon surrounding the fascicles where it is known as the endotenon. In vitro loading experiments generate a load-deformation curve from which the structural properties of ultimate tensile strength (kN) and stiffness (N/mm) can be derived (see Fig. 9.9). There are four regions to the curve: 1. The ‘toe’ region, where there is non-linear stretch to the tendon. This has been associated with the elimination of the crimp pattern of the collagen fibers. More recent work suggests that fascicle sliding also contributes significantly to initial elongation at low loads.52 2. Linear deformation is the area of the curve from which the stiffness is determined (load divided by deformation for the linear portion of the curve). The mechanism for this elongation is thought to arise from elongation of the collagen fibrils, fibrillar crimp straightening and/or sliding of fibrils and fibers relative to one another. Fiber sliding appears to provide the major mechanism enabling tendon fascicle extension within rat-tail tendon53 and equine SDFT.54 3. The yield region represents irreversible lengthening of the tendon, arising from slippage of collagen fibrils, fibers or fascicles. 4. Rupture, where the stress-strain curve falls quickly to zero as the collagen structures sequentially rupture. The ultimate tensile strength for the equine SDFT (rupturing at the mid-metacarpal region) in the horse is approximately 12 kN or 1.2 tonnes.52 The approximate ultimate tensile stress is 100MPa for the equine SDFT52, which agrees well with previously documented figures for other species (45–125MPa).55, 56 Equine SDFT strains of 16.6% have been recorded in vivo at gallop57 while values of around 25% have been reported before the tendon ruptures in vitro.27, 52 However, the ultimate tensile strain reflects only the final strain before rupture and includes that yield portion of the stress-strain curve that represents irreversible damage to the tendon tissue. In addition, the ultimate tensile strain may not be constant along the length of the SDFT in vitro58 Work has demonstrated that the normal strains in the digital flexor tendons in vivo (in ponies) are in the region of 2–4% at the walk and 4–6% at the trot.59 Riemersma and colleagues59 also noted that different results were obtained in vivo to in vitro, thereby indicating caution in the interpretation of in vitro measurements. Tendon is a viscoelastic tissue and as such, its response to mechanical loading is time and history dependent. The property known as hysteresis is demonstrated by the difference in the stress-strain relationship when the tendon is loaded compared to when it is unloaded (Fig. 9.10). The area between these two curves represents the energy lost during the loading cycle. This is usually about 5% in equine tendons. Much of this energy is lost as heat and is responsible for the rise in temperature within the tendon core associated with repeated loading (as in an exercising horse).60 These temperatures can rise to as high as 46°C, which is potentially damaging to either tendon matrix or tenocytes. However, tenocytes recovered from the center of equine SDFT remain viable when subjected to rises in temperature of this magnitude, whereas fibroblasts from other locations do not.61 This property is also present in fetal tenocytes, which suggests that the tendon has an inherent genetic adaptation to this physical process. The viscoelastic properties of tendon are demonstrated by movement of the stress-strain curve to the left or right (Fig. 9.10). Repeated loading results in shifting of the curve to the right (i.e. the tendon becomes less stiff), a process known as conditioning. This change is recoverable but significant resting time is necessary. This property, however, has been demonstrated in vitro and may not reflect the normal behavior in vivo.
Tendon and ligament physiology
Introduction
Definition and role of tendons and ligaments
Incidence of injury
Structure of tendon
Anatomical arrangement of the muscle-tendon-bone unit
Tendon as a structure
Tendon cells
CELL CLASSIFICATION
TYPE
NUCLEAR MORPHOLOGY
LOCATION
I
‘Resting’
Spindle-shaped nuclei lying between collagen fibers
Within the tensional region of all adult tendons
II
‘Active’
Cigar-shaped nuclei lying between collagen fibers
Within young tendon and both young and adult ligaments
III
‘Chondrocytic’
Round nuclei
In compressed regions of tendons and areas of chondroid metaplasia
IV
‘Precursor’
Round nuclei with prominent nucleoli
Endotendon
Tendon matrix
Molecular composition
Water content
Collagen component
Non-collagenous components
Other proteins
Morphology
Ultra-structural morphology
Micro- and macro-structural morphology
Functional characteristics of tendon
Load/deformation characteristics
Ultimate tensile strength and stress
Ultimate tensile strain
Hysteresis
Conditioning
Tendon and ligament physiology
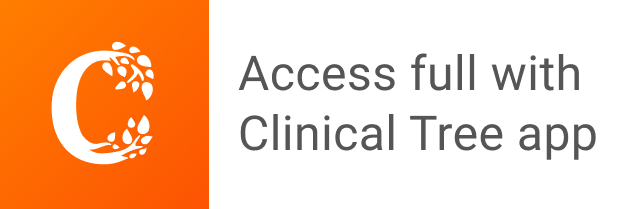