Chapter 13 Strong Ion Approach to Acid-Base Disorders
Determination of the mechanisms underlying acid-base disturbances has been an important clinical goal for more than 100 years. Landmark advancements in the clinical diagnosis and treatment of acid-base disturbances have included the Henderson-Hasselbalch equation (1916), the base excess (BE) concept (1960), calculation of the anion gap (AG) (1970s),41 introduction of the strong ion approach,50 and the simplified strong ion approach,5 and development of the strong ion gap (SIG) concept.6,30,32
The two main goals of acid-base assessment are to identify and quantify the magnitude of an acid-base disturbance and to determine the mechanism for the acid-base disturbance by identifying changes in variables that independently alter acid-base balance.5,14 Independent variables influence a system from the outside and cannot be affected by changes within the system or by changes in other independent variables. In contrast, dependent variables are influenced directly and predictably by changes in the independent variables. Singer and Hastings proposed in 194847 that plasma pH was determined by two independent factors, Pco2 and net strong ion charge, equivalent to the strong ion difference (SID, or the difference in charge between fully dissociated strong cations and anions in plasma). Stewart suggested in 1983 that a third variable, [Atot] or the total plasma concentration of nonvolatile weak buffers (e.g., albumin, globulins, and phosphate), also exerted an independent effect on plasma pH.50 One of Stewart’s major contributions to clinical acid-base physiology was his proposal that plasma pH was determined by three independent factors: Pco2, SID, and [Atot] (Figure 13-1). An understanding of the three independent variables (Pco2, SID, Atot) is required to apply the strong ion approach to acid-base disorders in dogs and cats.
Pco2
with pK′a being the negative logarithm of the apparent dissociation constant for carbonic acid (H2CO3) in plasma, and S being the solubility of CO2 in plasma. Because plasma pH is vigorously defended by animals to maintain a homeostatic environment, and because values for pK′a and S are fixed at constant temperature and ionic strength, the dependence of [] on Pco2 is clearly appreciated.
SID
Simple ions in plasma can be divided into two main types: nonbuffer ions (strong ions or strong electrolytes) and buffer ions. Strong ions are fully dissociated at physiologic pH and therefore exert no buffering effect. However, strong ions do exert an electrical effect because the sum of completely dissociated cations does not equal the sum of completely dissociated anions. Stewart termed this difference the SID.50 Because strong ions do not participate in chemical reactions in plasma at physiologic pH, they act as a collective positive unit of charge (SID).5,50 The quantitatively most important strong ions in plasma are Na+, K+, Ca2+, Mg2+, Cl−, lactate, ß-hydroxybutyrate, acetoacetate, and . The influence of strong ions on pH and [
] can always be summarized in terms of the SID. Changes in SID of a magnitude capable of altering acid-base balance usually occur as a result of increasing concentrations of Na+, Cl−,
, or organic anions or decreasing concentrations of Na+ or Cl−. An increase in SID (by decreasing [Cl−] or increasing [Na+]) will cause a strong ion (metabolic) alkalosis, whereas a decrease in SID (by decreasing [Na+] or increasing [Cl−], [
], or organic anions) will cause a strong ion (metabolic) acidosis.5,50 A Gamblegram of normal plasma (Figure 13-2) shows the relationship between strong cations, strong anions, and buffer ions (
and the nonvolatile weak acids, A−). A graphic representation of SID and the AG also is shown in Figure 13-2.
[Atot]
In contrast to strong ions, buffer ions are derived from plasma weak acids and bases that are not fully dissociated at physiologic pH. The conventional dissociation reaction for a weak acid (HA) and its conjugated base (A−) pair is HA ↔ H+ + A−. At equilibrium, an apparent dissociation constant (Ka) can be calculated.5,50 For a weak acid to act as an effective buffer, its pKa (defined as the negative logarithm of the weak acid dissociation constant Ka) lies within the range of pH ± 1.5. Because normal plasma pH is approximately 7.4, substances with a pKa between 5.9 and 8.9 can act as buffers. The main nonvolatile plasma buffers act as weak acids at physiologic pH (e.g., phosphate, imidazole [histidine] groups on plasma proteins). Also known as the non- buffer system, they form a closed system containing a fixed quantity of buffer. The non-
buffer system consists of a diverse and heterogeneous group of plasma buffers that can be modeled as a single buffer pair (HA and A−). An assumption in Stewart’s strong ion model is that HA and A− do not take part in plasma reactions that result in the net destruction or creation of HA or A−. When HA dissociates, it ceases to be HA (therefore decreasing plasma [HA]) and becomes A− (therefore increasing plasma [A−]). The total amount of A, or Atot, is the sum of A in dissociated [A−] and undissociated [HA] forms, which remain constant according to the law of conservation of mass.5,50
The great advantage of Stewart’s strong ion approach is that it provides a mechanistic view as to why pH is changing and fully integrates electrolyte and acid-base physiology. However, his approach is heavily mathematical and states that pH is a function of eight factors50 (for comparison, the Henderson-Hasselbalch equation is mathematically simpler, stating that pH is a function of four factors, whereas Constable’s simplified strong ion equation states that pH is a function of six factors).5 With that in mind, this chapter will focus on the concepts behind the strong ion approach, emphasizing the relationship between weak and strong ions and acid-base balance and developing an understanding of why pH and [] are changing. Frameworks adapting the strong ion approach to clinical uses also will be reviewed. The mathematical and physicochemical background of this approach is described in detail elsewhere.* A comparison of diagnostic approaches using routine screening (total CO2), the Henderson-Hasselbalch approach, and the simplified strong ion approach is shown in Table 13-1.
Because clinically important acid-base derangements result from changes in Pco2, SID, or Atot, the strong ion approach distinguishes six primary acid-base disturbances (respiratory, strong ion, or nonvolatile buffer ion acidosis and alkalosis; Figure 13-3) instead of the traditional four primary acid-base disturbances (respiratory or metabolic acidosis and alkalosis) characterized by the Henderson-Hasselbalch equation.5,7,8,13 Acidemia (decrease in plasma pH) results from an increase in Pco2 and nonvolatile buffer concentrations (albumin, globulin, phosphate) or from a decrease in SID. Alkalemia (increase in plasma pH) results from a decrease in Pco2 and nonvolatile buffer concentration or from an increase in SID.
Disorders of Pco2
Increases in Pco2 are associated with respiratory acidosis, whereas decreases in Pco2 lead to respiratory alkalosis. There are no differences between the Henderson-Hasselbalch approach and the strong ion approach in relation to Pco2. See Chapter 11 for further discussion of respiratory acid-base disorders.
Disorders of [Atot]
Values for the total plasma concentration of nonvolatile weak acids and the effective dissociation constant (Ka) for plasma nonvolatile buffers are species specific. Values for Atot and Ka have been experimentally determined in the plasma of humans,49 cats,37 and dogs12 and theoretically determined for the plasma of humans.8 Canine and feline plasma proteins have a greater net negative charge than human plasma proteins because their albumin contributes proportionally more to net protein charge, has a different amino acid composition, and carries a greater net negative charge at physiologic pH. These characteristics explain the higher AG in dogs and cats because the AG in healthy dogs and cats essentially reflects the total protein concentration. Atot in dogs is 17.4 mmol/L (equivalent to 0.27 mmol/g of total protein or 0.47 mmol/g of albumin), whereas Ka is 0.17 × 10−7 (pKa = 7.77).12 Atot in cats is 24.3 mmol/L (equivalent to 0.35 mmol/g of total protein or 0.76 mmol/g of albumin), whereas Ka is 0.67 × 10−7 (pKa = 7.17).37
The contribution of proteins to Atot has been determined in vitro for dogs.12 The net protein charge of canine plasma at pH = 7.40 is approximately 16 mEq/L, equivalent to 0.25 mEq/g of total protein or 0.42 mEq/g of albumin. The overall effect of a 1-g/dL increase in total protein concentration is a decrease in pH of 0.047.
The contribution of proteins to Atot has been determined in vitro for cats.37 The net protein charge of feline plasma at pH = 7.40 is approximately 14 mEq/L, equivalent to 0.19 mEq/g of total protein or 0.41 mEq/g of albumin. The overall effect of a 1-g/dL increase in total protein concentration is a decrease in pH of 0.093.
Nonvolatile buffer ion alkalosis
Hypoalbuminemia
The fact that hypoalbuminemia tends to increase pH and cause metabolic alkalosis was first identified in people in 1986 by McAuliffe et al.36 Phosphate is quantitatively the second most important component of [Atot] and normally is present in plasma at a low concentration (<4 mmol/L). Therefore hypophosphatemia is not a clinically important cause of nonvolatile buffer ion (metabolic) alkalosis. Globulin is quantitatively the third most important component of [Atot]; however, decreases in plasma globulin concentration usually accompany decreases in plasma albumin concentration. In other words, nonvolatile buffer ion alkalosis in dogs and cats is usually due to hypoalbuminemia.
The effects of a decrease in Atot (Atot alkalosis) on [] are shown in a Gamblegram in Figure 13-4. Hypoproteinemic alkalosis has been identified clinically in dogs and cats.18,32,52 In vitro, a 1-g/dL decrease in albumin concentration is associated with an increase in pH of 0.093 in cats37 and 0.047 in dogs.12 The increase in pH secondary to hypoalbuminemia should result in ventilatory compensation (hypoventilation) or a decrease in SID because plasma pH is vigorously defended and any deviation in pH from the optimal range for a given core temperature will be energetically inefficient. Data are not currently available regarding the presence or absence of ventilatory compensation for nonvolatile buffer ion alkalosis in dogs or cats. However, compensatory hypoventilation and increased Pco2 have been documented in human patients with hypoalbuminemic alkalosis.32,36 In contrast, hyperventilation and decreased Pco2 were identified in humans with congestive heart failure and cirrhosis, although it is likely that hyperventilation may have been induced by the underlying diseases and not by the metabolic acid-base disorder.45 Metabolic compensation with decreased SID caused by an increase in chloride concentration occurs in human patients with hypoproteinemic alkalosis.36,54
The most common causes of hypoproteinemic alkalosis are shown in Box 13-1. Hypoalbuminemic alkalosis is common in the critical care setting.22 Presence of hypoalbuminemia complicates identification of increased unmeasured anions (e.g., lactate, ketoanions) because hypoproteinemia not only increases pH but also decreases AG.20,24,28 Thus the severity of the underlying disease leading to metabolic acidosis may be underestimated if the effects of hypoalbuminemia on pH, [], and AG are not considered. Calculation of the SIG is therefore preferred in dogs and cats with hypoalbuminemia because hypoproteinemia will not alter the SIG. Treatment for hypoalbuminemic alkalosis should be directed at the underlying cause and the decreased colloid oncotic pressure.
Nonvolatile buffer ion acidosis
Hyperphosphatemia
Hyperphosphatemia, especially if severe, can cause a large increase in [Atot], leading to metabolic acidosis (see Box 13-1). Although extremely rare, an increase in albumin concentration also can lead to metabolic acidosis.46 A Gamblegram representing Atot acidosis is shown in Figure 13-4. The most important cause of hyperphosphatemic acidosis is renal failure. Metabolic acidosis in patients with renal failure is multifactorial but mostly is caused by hyperphosphatemia and increases in unmeasured strong anions such as sulfate.40,44 Hyperphosphatemic acidosis also has been observed after hypertonic sodium phosphate enema administration in cats.2 In experimental cats that have been given hypertonic sodium phosphate enemas, serum phosphate concentration changed from a mean of 2.8 mEq to 8.1 mEq/L within 15 minutes after a 60-mL dose and from 2.3 mEq/L to 8.8 mEq/L within 30 minutes with a 120-mL dose.2 In both groups, the increase in [SID] was enough to offset the increase in lactate concentration, whereas protein concentration did not change. Because Pco2 also did not change, the metabolic acidosis could be caused by an increase in unmeasured anions or phosphate, but an increase in any organic acid other than lactate would have been unlikely. Using the data from this study, a strong correlation can be found between changes in phosphate and changes in BE (r = 0.95 with the 60-mL dose, and r = 0.96 with the 120-mL dose). More than 90% of the change in the AG in the first 4 hours can be explained entirely by changes in lactate and phosphate. Clinically, hyperphosphatemic acidosis also occurred in a cat that received a phosphate-containing urinary acidifier.26 Treatment for hyperphosphatemic acidosis should be directed at the underlying cause. Sodium bicarbonate administered intravenously may be used as adjunctive therapy in patients with hyperphosphatemic acidosis3 because the increase in plasma sodium concentration results in an increase in plasma SID (strong ion alkalosis) expansion of the extracellular fluid volume (which is the distribution space for phosphorus), and increased urine production. The combined effect of these changes is to decrease plasma phosphorus concentration.
Disorders of SID
Changes in SID usually are recognized by changes in [], BE, [Na+], or [Cl−] from their reference values. It is important to understand that the change in SID from normal is equivalent to the change in [
] or BE from normal whenever the plasma concentrations of nonvolatile buffer ions (albumin, phosphate, globulin) are normal.7 In other words, the Henderson-Hasselbalch and strong ion approaches are equivalent whenever plasma albumin, phosphate, and globulin concentrations are within their reference ranges. It is also important to realize that the sodium-chloride difference ([Na+] – [Cl−]) provides a clinically valuable index of SID, particularly in animals that do not have hyperlactatemia.
A decrease in SID results in a strong ion (metabolic) acidosis, whereas an increase in SID results in a strong ion (metabolic) alkalosis.5,7 There are three general mechanisms by which SID can change (Table 13-2): (1) an increase in strong anions relative to strong cations; (2) a decrease in strong anions relative to strong cations; and (3) a change in the free water content of plasma (change in extracellular fluid volume) with no change in strong anions relative to strong cations.
Table 13-2 Mechanisms for SID Changes
Disorder | Mechanism | Clinical Recognition |
---|---|---|
SID Acidosis | ||
![]() | ![]() ![]() | Dilutional acidosis |
![]() | ![]() | Hyperchloremic acidosis |
![]() | Organic acidosis | |
SID Alkalosis | ||
![]() | ![]() ![]() | Concentration alkalosis |
![]() | ![]() | Hypochloremic alkalosis |
SID Alkalosis
There are three general mechanisms by which SID can increase, leading to metabolic alkalosis: an increase in [Na+], a decrease in [Cl−], or a decrease in plasma free water, which occurs commonly in dehydration. Strong cations other than sodium are tightly regulated, and changes of a magnitude that could affect SID clinically either are not compatible with life or do not occur. Conversely, chloride is the only strong anion present in sufficient concentration to cause an increase in SID when its concentration is decreased. Common causes of SID alkalosis are presented in Box 13-2.
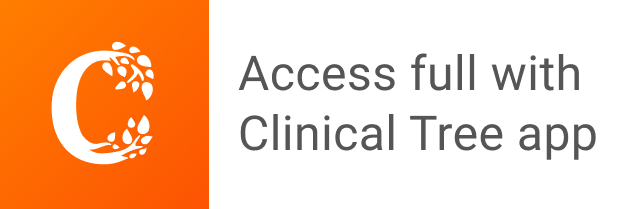