Chapter 57 Small Intestine
Structure and Function
The small intestine (SI) is, in essence, an interface between the external environment and the body, and is both an absorptive surface and a barrier; it must digest and absorb nutrients while excluding antigens and microbes and eliminating fecal waste. It faces a frequently changing dietary and bacterial intake, and yet has to maintain a dynamic but balanced microflora within its lumen while being intermittently exposed to pathogens. All of its functions—mixing and propulsion, secretion, digestion, absorption, regulation of blood flow, immunologic reaction and tolerance, and elimination—are fully integrated through both local and remote neuroendocrine and immunologic mechanisms (see Chapter 1). It thus has a complex task and requires specialized anatomic arrangements to perform them.
Gross Structure
Anatomic Regions
The SI is basically a tube, beginning at the pylorus of the stomach and ending at the ileocolic valve. However, this tube is ultimately in continuity with the external environment, proximally from the mouth via the esophagus and stomach, and distally to the anus via the large intestine (Figure 57-1, A).1–3 It is relatively short, reflecting the typical dietary intake of cats and dogs. It is approximately 1 to 1.5 meters long in adult cats and ranges from 1 to 5 meters in adult dogs, in proportion to the size of the individual. It is divided arbitrarily into three anatomic segments: the duodenum proximally, then the jejunum, and finally the ileum distally (see Figure 57-1, A).
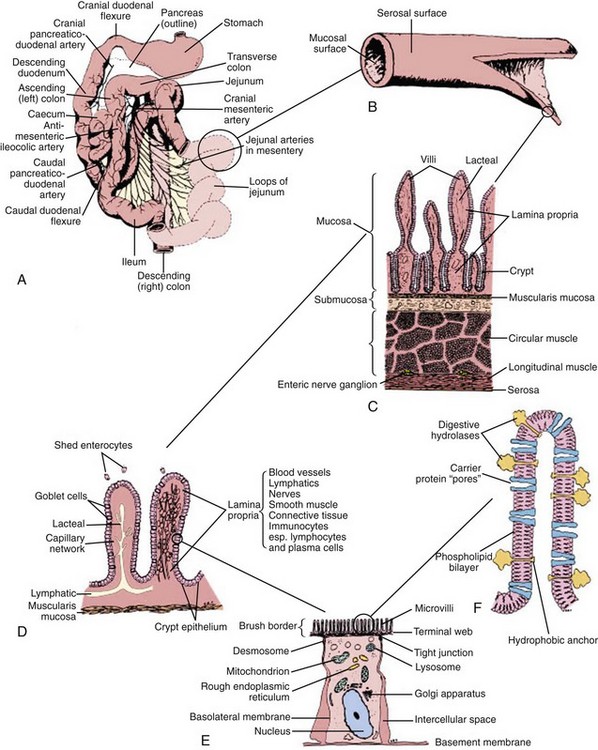
Figure 57-1 Functional anatomy of the small intestine.
(From Hall EJ: Small intestinal disease. In: Gorman NT, editor: Canine Medicine and Therapeutics, ed 4, Oxford, UK, 1998, Blackwell Science, p 488.)
Duodenum
The common bile duct and one pancreatic duct enter the duodenum via the major papilla. In dogs an accessory pancreatic duct often enters at a minor papilla more distally and slightly more ventrally (Figure 57-2, A), but there is a range of variations in the actual number of ducts and their drainage pattern from the pancreas (see Chapter 60). The papillae are notable endoscopic landmarks in dogs, but may not be obvious in cats.
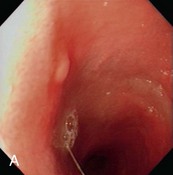
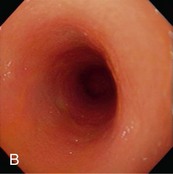
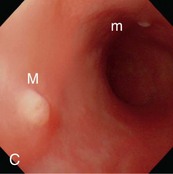
Figure 57-2 Videoendoscopic appearance of the normal upper small intestine.
(Reprinted with permission from Lhermette P, Sobel D: BSAVA Manual of Canine and Feline Endoscopy and Endosurgery. Gloucester, UK, 2008, BSAVA Publications.)
The distal duodenal flexure, where the duodenum courses to the left side of the abdomen (see Figure 57-2, B) is often at the limit of the reach of a standard 1-meter gastroscope, except in cats and small dogs. In dogs the antimesenteric side of the duodenum is marked by a line of whitish, mucosal depressions signifying the presence of specialized lymphoid areas, the Peyer patches (see Figure 57-2, C). Secretory Brunner glands and annular mucosal folds are features of the human proximal duodenum, but are not present in dogs and cats. After the distal duodenal flexure, the ascending limb of the duodenum crosses the midline and ends at the level of L6 close to the root of the mesentery near the left kidney, with a mesenteric attachment to the colon, the duodenocolic ligament.
Intestinal Compartments
Microflora
The microflora of the SI is an integral part of its structure and function. There is a gradual increase in bacterial numbers and a shift from aerobic to anaerobic organisms progressing distally down the SI. Chapter 2 provides a more detailed description of the composition of the microflora and its interaction with the mucosa.
Mucosa
One of the most important structural modifications of the mucosa is a vast increase in its surface area relative to the size of the animal, with an almost 600-fold increase compared with the basic tubular structure of the intestine. The surface area of the human intestine has been estimated at 175 m2, and although the adult human intestine is longer than in even the largest dog, the villi in cats and dogs are almost twice as long (approximately 1 mm) compared with those of humans. The increase in surface area is created by folds in the mucosal wall (tripling the surface area), villus projections into the intestinal lumen (providing an approximate 10-fold increase), and microvilli on the surface of each epithelial cell (providing a further 20-fold increase in area) (see Figure 57-1, C). Diseases causing villus atrophy or even just microvillus damage are likely to produce profound malabsorption and diarrhea.
Gut-Associated Lymphoid Tissue
The GI tract is the largest immunologic organ in the body, and the SI comprises a large component of the mucosal immune system. Within the SI, the Peyer patches (see Figure 57-2, C) act as inductive sites and are covered with a specialized epithelium containing microfold (M) cells, which sample luminal antigens. Activated lymphocytes migrate via mesenteric lymph nodes to the circulation, from where they home to their effector sites, the lamina propria and epithelium. Chapter 3 details the structure and role of the gut-associated lymphoid tissue.
Microstructure
An identical, basic, tubular, cross-sectional structure is present throughout the length of the SI (see Figure 57-1, C): the external serosa surrounds the muscularis, submucosal and mucosal layers which are present throughout, and can be detected ultrasonographically (Figure 57-3).4–10 A very narrow hyperechoic interface between the lumen and mucosal surface is usually visible above the four true layers: (a) a slightly hypoechoic mucosa, (b) hyperechoic submucosa, (c) hypoechoic muscularis, and (d) brightly hyperechoic serosa.
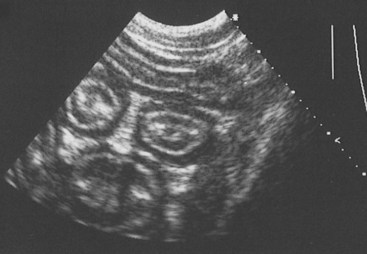
Figure 57-3 Ultrasound Image of the Small Intestine.
(From Ettinger SJ and Feldman EC, editors: Textbook of Veterinary Internal Medicine, ed 7, Philadelphia. 2010, Saunders, p 1541.)
The mucosal layer is responsible for secretion and absorption as well as being a barrier to the luminal environment. The submucosa, between the muscularis mucosa and muscularis, provides connective tissue support and delivers blood vessels, nerves, and lymphatics. Within the muscularis, the outer longitudinal and inner circular muscular layers provide propulsive and segmental peristaltic contractions that mix chyme and ultimately propel it aborally. Neural plexuses are found between the muscle layers (the myenteric or Auerbach plexus) and in the submucosa (Meissner plexus), and communicate with all layers of the intestinal wall. They help coordinate intestinal motility and secretory activity, and even mucosal immune responses (see Chapters 1 and 3).
Mucosa
This is the most important layer of the intestine clinically. It is comprised of the epithelium and lamina propria overlying the muscularis mucosa, and is modified by gross folds and the villi (see Figure 57-1, C). The muscularis mucosa is a thin sheet of smooth muscle, from three to 10 cells thick, separating the mucosa from the submucosa. Smooth muscle branches within the villus lamina propria enable shortening and lengthening movements of the villi.
The lamina propria is a continuous connective tissue space bounded by the muscularis mucosa below and the epithelium above, and contains aggregates of lymphoid tissue, and nonaggregated immunocytes (see Chapter 3), enteric neurons, and blood and lymphatic vessels. A central lymphatic vessel (lacteal) within each villus drains chylomicrons into intestinal lymphatics and ultimately to the cisterna chyli.
Crypt-Villus Unit
A group of crypts and their associated villus comprise the functional unit of the SI (see Figure 57-1, D).11 Crypts are continually replenished by cell division, producing undifferentiated epithelial cells. It is estimated that there are between four and 40 stem cells per crypt in the adult intestine, with further division of daughter cells occurring as the cells pass up the crypt. As the crypt cells pass through a maturation zone they undergo a final division and differentiate into immature epithelial cells. The predominant epithelial cell type is the enterocyte, but as a number of crypts may supply the enterocytes to one villus, each villus epithelium may consequently represent a polyclonal cell population.
Mucosal Epithelium
The intestinal surface is covered by a monolayer of polarized epithelial cells; their luminal surface is structurally and functionally distinct from their basolateral membrane.12–18 The epithelial basement membrane is readily permeable to nutrients, but has an important role as the structural matrix on which the epithelium grows. It expresses glycoproteins, called laminins, that interact with integrins, transmembrane recognition molecules expressed by epithelial cells. These interactions promote cell adhesion, growth, polarization, and differentiation. Enterocyte differentiation during migration up the villus may be programmed, but is likely also to be modulated by the expression of different integrins at different sites on the crypt–villus axis. Communication between epithelial cells is mediated by E-cadherin, a transmembrane molecule, linked to intracellular catenins, proteins that transmit signals to the actin cytoskeleton and to intracellular growth control pathways.
A mucosal barrier is formed by the intestinal epithelium (Box 57-1). This barrier depends on intercellular tight junctions between enterocytes, encircling their lateral aspects and excluding antigens and bacteria. Effete enterocytes are shed from the villus tip by a mechanism that maintains the mucosal barrier (see Figure 57-1, D). Studies in rodents suggest intercellular bridges develop between neighboring enterocytes below the effete cell before it is shed, thus maintaining mucosal integrity. However, epithelial integrity is likely to be altered in some intestinal diseases, and the integrity of the tight junctions is actually least in the crypts, where fluid secretion occurs. There is an association of cryptal lesions with the development of PLEs.
In the Peyer patches, enterocytes overlying lymphoid aggregates are modified into follicle epithelium and M cells, probably in response to signals from underlying lymphoid cells. The M cells sample the luminal contents and help present antigen to the mucosal immune system (see Chapter 3).
Enterocytes
Enterocytes contain the intracellular organelles, such as mitochondria, lysosomes, and endoplasmic reticulum, common to all cells, and which support normal cellular functions. However, enterocytes also perform specific digestive and absorptive functions.19,20 Enzymes expressed on the surface of enterocytes perform terminal digestion of polysaccharides and peptides in conjunction with luminal hydrolysis of food polymers by pancreatic enzymes. The enterocytes then absorb the simple nutrients. These functions depend on the polarity of the enterocyte, involving a specialized portion of the cell membrane on the luminal surface, the microvillar membrane (MVM). The microscopic appearance of the MVM is the basis of its alternative name, the “brush-border” (see Figure 57-1, E and F). It consists of thousands of parallel cylindrical processes (microvilli) bearing the digestive enzymes and specific carrier proteins.
The MVM is a phospholipid bilayer that has specific proteins inserted into it. Enzymes responsible for the terminal stages of carbohydrate and protein digestion are usually anchored in the MVM by a small hydrophobic terminal and have an active site exposed to the intestinal lumen (see Figure 57-1, F). Specific carrier proteins traverse the MVM or basolateral membrane and, through conformational changes, shuttle nutrients into and out of the enterocyte across the cell membrane. The maximal brush-border enzyme and transport activities are expressed in the mid-villus region. Diseases damaging enterocytes often accelerate cell production and the more immature enterocytes are not as effective functionally.
Enterocyte metabolism is geared toward the production of brush-border proteins and the transfer of nutrients and water from the lumen to the blood. Basolateral cell membranes export sodium from the cell via an energy-dependent N+-K+-ATPase. Water can follow osmotically, or compensatory sodium influx at the luminal surface can drive carrier-mediated nutrient absorption. Natural inhibition of glycolysis through expression of an alternate phosphofructokinase isoenzyme in enterocytes facilitates the transfer of glucose from the lumen to the blood. Gluconeogenesis is also inhibited, and so enterocytes can utilize ketone bodies. However their major energy source is actually glutamine (Figure 57-4). A surge in enterocyte glutamine metabolism during digestion is probably partly responsible for the postprandial rise in blood ammonia seen in some patients with hepatic dysfunction.
Muscularis
Two muscle layers, the outer longitudinal and inner circular layers, encircle the submucosa. The intermuscular plane is a connective tissue layer bearing the myenteric (Auerbach) neural plexus. Ring contractions by the circular muscle and sleeve contractions by the longitudinal muscle may be tonic or rhythmic, and intestinal movements may be standing or migrating, allowing mixing and propulsion. Contraction of the muscle layers is coordinated by the enteric nervous system to produce peristaltic and segmental movements, with interstitial cells of Cajal acting as pacemakers. Chapter 1 details how intestinal motility is integrated with other functions of the SI.
Small Intestinal Function
The basic functions of the SI, that is digestion, absorption, and elimination, occur as a result of complex intercellular interactions between epithelial cells, immune cells, mesenchymal and neuronal cells and with luminal nutrients and microbes.21 The SI is also the largest immunologic organ in the body, interacting with the intestinal microbial flora and a diverse range of food antigens (see Chapters 2 and 3, respectively).
Digestion
To be transported across the MVM, major dietary constituents must be hydrolyzed from their initial polymeric structure into monomers. This digestive process is achieved within the SI lumen by mechanical disruption (in conjunction with bile salt emulsification of fats) that allows enzymatic hydrolysis of polysaccharides, proteins, and triglycerides.22
Carbohydrate
Starch and glycogen are the major carbohydrates in the diet and must be hydrolyzed completely to glucose for absorption (Figure 57-5, A). There is no salivary amylase activity in dogs and cats, and these complex carbohydrates are hydrolyzed by pancreatic α-amylase. Straight-chain starch molecules (amylose) are split to maltose, maltotriose, and some glucose. Branched-chain starch molecules (amylopectin) and glycogen are also hydrolyzed to the same products, except that the branched parts of their molecules remain as α-limit dextrins as their 1,6-glycosidic bond cannot be hydrolyzed by α-amylase. The digestion products of α-amylase are subsequently hydrolyzed, particularly by brush-border maltase (glucoamylase) and isomaltase (α-dextrinase). The brush-border enzyme trehalase hydrolyzes the 1,1 link in the fungal sugar trehalose, but is not expressed in cats.
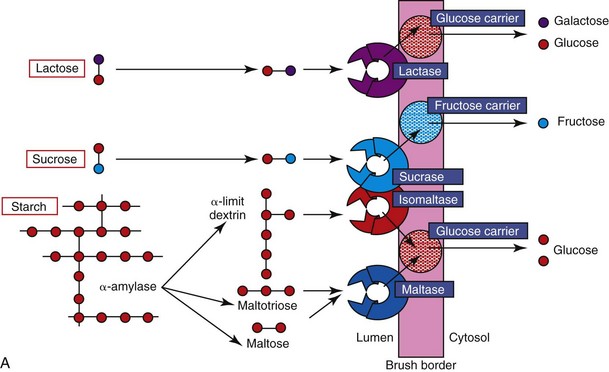
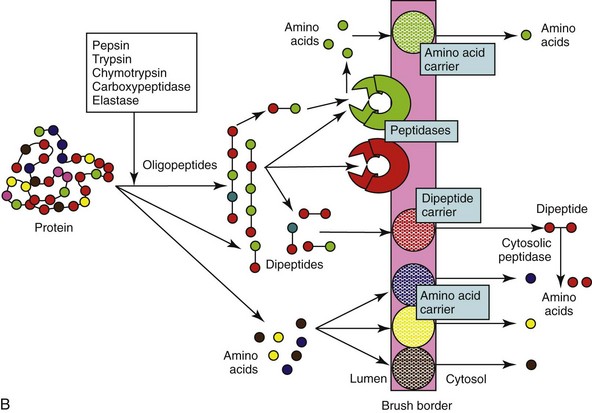
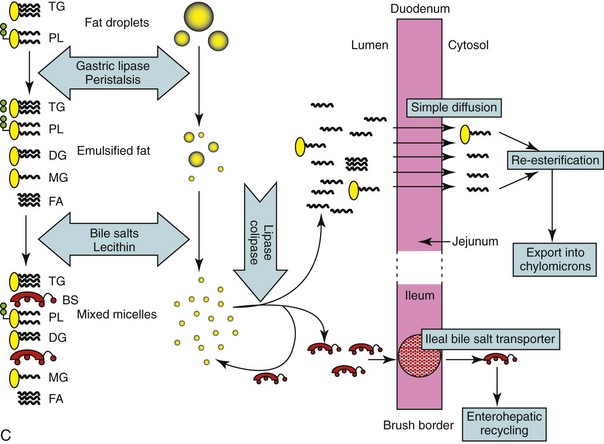
Figure 57-5 Diagram of the digestion and absorption of (A) carbohydrate, (B) protein, and (C) fat.
(Adapted from Batt RM: The molecular basis of malabsorption. J Small Anim Pract 21:555, 1980.)
Sucrose is an unusual constituent of canine and feline diets unless semimoist pet foods or human foods are fed. It is hydrolyzed directly at the brush-border to glucose by sucrase, part of the sucrase–isomaltase brush-border enzyme complex. Congenital sucrase–isomaltase deficiency is a rare genetic defect of man, but has not been recorded in small animals. Sucrase activity in cats is lower than dogs (Table 57-1), probably reflecting the average composition of their diets.
Protein
Digestion of proteins follows a similar overall pattern to carbohydrate digestion (see Figure 57-5, B), and the amounts of pancreatic enzyme secreted and mucosal peptidases expressed are influenced by the protein content of the diet. Digestion is initiated by acidic denaturation and the proteolytic activity of pepsin in the stomach. Luminal digestion under a more neutral pH is continued in the SI by pancreatic proteases (trypsin, chymotrypsin, elastase, and carboxypeptidase), which are initially secreted as inactive proforms, and are subsequently activated by enterokinase and trypsin. Luminal proteolysis results in a mixture of oligo-, tri-, and dipeptides as well as free amino acids. Oligopeptides are subsequently hydrolyzed by brush-border peptidases, which have some selectivity for particular amino acid residues. However, there is considerable overlap in specificity, and a selective deficiency of aminopeptidase N reported in dogs is of no clinical consequence. Furthermore, any tri- and dipeptides can still be absorbed on a brush-border carrier. Theoretically a deficiency of enterokinase could cause protein malabsorption through failure of trypsin activation, but this has never been documented in dogs and cats, and trypsin autoactivation would probably still occur.
Lipid
Fat digestion is completed entirely within the GI lumen by secreted enzymes and bile salts. Partial digestion is begun in the stomach by the action of gastric lipase secreted by gastric epithelial mucus cells. Subsequent mixing of the fat emulsifies it into small droplets. Further mixing with bile and pancreatic juice results in the formation of mixed micelles, which are approximately the size of the smallest fat droplet and solubilize approximately 1000 times more fatty acids. At the surface of mixed micelles, triglyceride is hydrolyzed by pancreatic lipase to di- and monoglycerides and free fatty acids (Figure 57-6). Maximal lipase activity in the gut lumen is dependent on a protein cofactor, colipase, which is secreted by the pancreas as inactive procolipase. There is some reserve capacity for fat digestion if pancreatic function is normal, and a fat-rich diet, especially one rich in unsaturated fatty acids, also stimulates increased pancreatic lipase secretion. However, neuroendocrine mechanisms initiated by the presence of fat in the duodenum and ileum, control the rate of gastric emptying and hence the rate of fat delivery. Thus a fat-rich diet or intestinal fat malabsorption delays gastric emptying.
Absorption
Digested Nutrients
Simple sugars, amino acids and oligopeptides, and fatty acids and other lipids are delivered to the body across the mucosal barrier and then via the lymphatics or bloodstream.23,24 Uptake occurs by passive diffusion or by active or facilitated carrier-mediated transport mechanisms (see Figure 57-6). Endocytosis of small, antigenic peptides is of no nutritional significance, but is involved in the neonatal absorption of colostral antibodies, and is crucial to the mucosal immune response.
Mechanisms of Absorption
Carrier-Mediated Transport
Active transport of substrates across the MVM into the enterocyte is usually against a concentration gradient and energy must be expended to drive the process (see Figure 57-6). Usually the uptake of the nutrient is linked to the entry of sodium down its electrochemical gradient, with energy expenditure by a N+-K+-ATPase on the basolateral membrane of the enterocyte pumping sodium back out of the cell.
Facilitated transport is the carriage of substrates by a transport protein across the MVM, down a concentration gradient without energy expenditure (see Figure 57-6). Some sugars, oligopeptides, and folate are absorbed by this process. The number of carriers is finite, and the process saturable and subject to competitive inhibition.
Endocytosis
Small antigenic peptides may be engulfed nonspecifically within endocytotic vesicles of epithelial cells. The amounts absorbed by this route are negligible from a nutritional standpoint, but this sampling of luminal contents is crucial to the mucosal immune response (see Chapter 3). Receptor-mediated endocytosis enables the uptake of small amounts of a specific intact nutrient and is the mechanism of cobalamin absorption.
Nutrient Absorption
Carbohydrate
The main product of carbohydrate digestion, glucose, is absorbed by active transport on a stereo-specific carrier that recognizes a D-pyranose structure with a C-2 hydroxyl group. Glucose is cotransported with sodium; the energy required for the coupling is provided through the entry of sodium down a concentration gradient but with the basolateral N+-K+-ATPase reexporting sodium against the concentration gradient. The carrier molecule in the brush-border has been identified in many species, including dogs and cats, as the sodium-glucose cotransporter protein (SGLT1; Figure 57-7). This molecule has the highest affinity for glucose, but it is also the carrier for galactose. Indirect evidence for this is shown by the inability to absorb either sugar in people with glucose–galactose malabsorption in whom a single amino acid mutation (Asp28 → Asn28) in the SGLT1 protein has been identified. Glucose and galactose thus may exhibit competitive inhibition, but glucose is the major substrate. There is circumstantial evidence for another aldohexose carrier in cats.
Facilitated transport of glucose across mammalian cell membranes is performed by a family of facilitated glucose transporters (GLUTs) with different isoforms found in different tissues. One member of this family, GLUT2, is found on the basolateral membrane of enterocytes, where it shuttles glucose, galactose, and fructose out of the enterocyte by facilitated diffusion (see Figure 57-7). GLUT2 is absent from the brush-border, and so a mechanism exists for active transport of glucose across the MVM into the enterocyte by SGLT1 and facilitated transport into the body by GLUT2. Most of the glucose is not used within the enterocyte, because of expression of a phosphofructokinase isomer that directs metabolism away from glycolysis.
Protein
The products of protein digestion are absorbed on carriers that are stereo-specific for L-amino acids (Figure 57-8; also see Figure 57-5, B).25 Sodium-linked active transport is responsible for free amino acid uptake via one of four different carriers that have a variable degree of selectivity for neutral (Gly, Ala), acidic (Asp, Glu), basic (Arg, Lys), and imino (Pro, HO-Pro) amino acids. The cat has the highest rate of uptake of basic amino acids perhaps because it has an essential requirement for arginine.
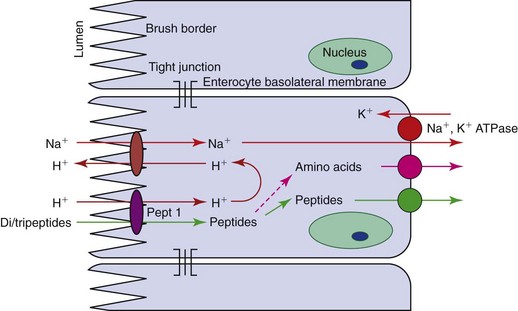
Figure 57-8 Diagram of the absorption of di- and tripeptides by enterocytes. Pept 1, a peptide carrier.
Traditionally, peptide uptake has been considered to be facilitated diffusion, with the concentration gradient being maintained by intracellular peptide hydrolysis, and only free amino acids being exported from enterocytes into the portal blood (see Figure 57-8). A single carrier for di- and tripeptides with no selectivity for their amino acid content has been demonstrated. However, in people this peptide carrier, Pept-1, is involved in the active influx of peptides, being linked to the influx of H+ down an electrochemical gradient. The protons are exchanged across the MVM with sodium, which is pumped out by the basolateral N+, K+ ATPase. A mixture of peptides and free amino acids is exported to the blood, but it appears that peptides are absorbed more readily than free amino acids. This has clinical significance as the inclusion of dipeptides in elemental diets has a theoretical advantage over simple amino acid solutions. This transport protein is also the carrier for peptidomimetic drugs such as β-lactams and angiotensin-converting enzyme inhibitors.
Lipid
The products of fat digestion are absorbed by passive diffusion from mixed micelles into lacteals (see Figure 57-5, C). The limiting factors, assuming normal pancreatic function, are the intestinal surface area and lymphatic functionality, and so villus atrophy and lymphangiectasia are likely to cause malabsorption of fat.
Water-Soluble Vitamins
Folic acid is present in adequate amounts in most commercial foods, but is also produced by the enteric flora. It is usually conjugated in a poorly absorbable polyglutamate form and must be hydrolyzed by folate deconjugase, a brush-border enzyme, before absorption. Folate (pteroyl monoglutamate) is absorbed by a carrier-mediated process at low luminal concentrations and by passive diffusion at high concentrations (Figure 57-9). After absorption folate is methylated in the cell to form methyltetrahydrofolate.
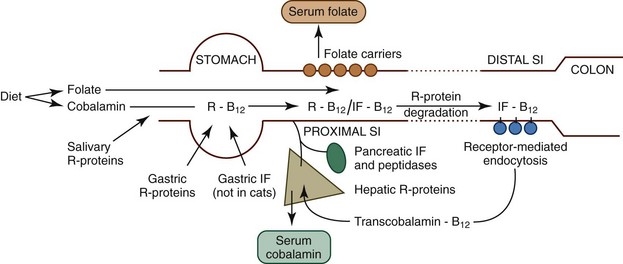
Figure 57-9 Diagram of the absorption of folate and cobalamin.
(From Ettinger SJ, Feldman EC, editors: Textbook of Veterinary Internal Medicine, ed 7, Philadelphia, 2010, Saunders, p 1528, Figure 270-1A.)
Vitamin B12 (cobalamin) is absorbed by receptor-mediated endocytosis in the ileum (see Figure 57-9), but the process is complex so that intact cobalamin is absorbed and potentially harmful analogues are excluded. Following ingestion, cobalamin is released from food in the stomach and then bound by R proteins (haptocorrins), which are nonspecific binding proteins of salivary and gastric origin. At acidic pH, cobalamin has high affinity for R proteins, but on entering the more alkaline environment of the SI, R proteins bind cobalamin less avidly and undergo proteolysis. Thus cobalamin is transferred to another binding protein, intrinsic factor, which promotes cobalamin absorption in the ileum. The source of intrinsic factor is the stomach and pancreas in dogs and solely the pancreas in cats. Intrinsic factor–bound cobalamin complexes pass to the ileum until they bind specific receptors and are endocytosed. Cobalamin is passed into the portal blood where it is bound to a protein, transcobalamin 2, enabling it to enter tissues and to be reexcreted in bile. Inherited abnormalities of the cobalamin–intrinsic factor receptor in breeds such as the Giant Schnauzer and Border Collie cause selective cobalamin deficiency.
Motility
Slow wave, segmental, and peristaltic contractions of the SI are generated by the coordinated contraction of smooth muscle in response to spontaneous electrical activity.26–34 Interstitial cells of Cajal are considered coordinating/pacemaker cells and smooth muscle contraction is also modulated by coordinated neurohumoral and neurochemical molecule release. Many of these molecules are also involved in the regulation of intestinal secretion and absorption and the mucosal immune response, producing a complex coordinated process for the digestion of food.
Secretion and Absorption of Water and Electrolytes
Control of Fluid Balance
Intestinal fluid balance is regulated by the neurocrine systems in the submucosal plexus as a largely autonomous process.35,36 Acetylcholine and vasoactive intestinal polypeptide are major mediators of secretion, increasing intracellular calcium and cyclic adenosine monophosphate (cAMP), inhibiting neutral sodium and chloride absorption, and facilitating transcellular chloride efflux. Many bacterial agents exert their diarrheagenic effects by increasing cAMP in enterocytes. The principal regulators of absorption—noradrenaline, somatostatin, and opioids—lower intracellular cAMP and calcium concentrations and stimulate neutral NaCl absorption and thereby can have therapeutic antidiarrheal effects.
Diagnostic Evaluation
History
Clinical Signs
The cardinal sign of small intestinal disease is diarrhea, but other signs (Box 57-2) may occur in the absence of diarrhea. Vomiting may be stimulated by intestinal distention or inflammation, and, indeed, vomiting is the most common manifestation of inflammatory bowel disease (IBD) in cats. Vomiting of blood may indicate gastric and/or upper GI bleeding, and copious volumes of bilious vomit are suggestive of upper GI obstruction. More distal obstructions of the SI may cause infrequent vomiting of a fecal-like material.
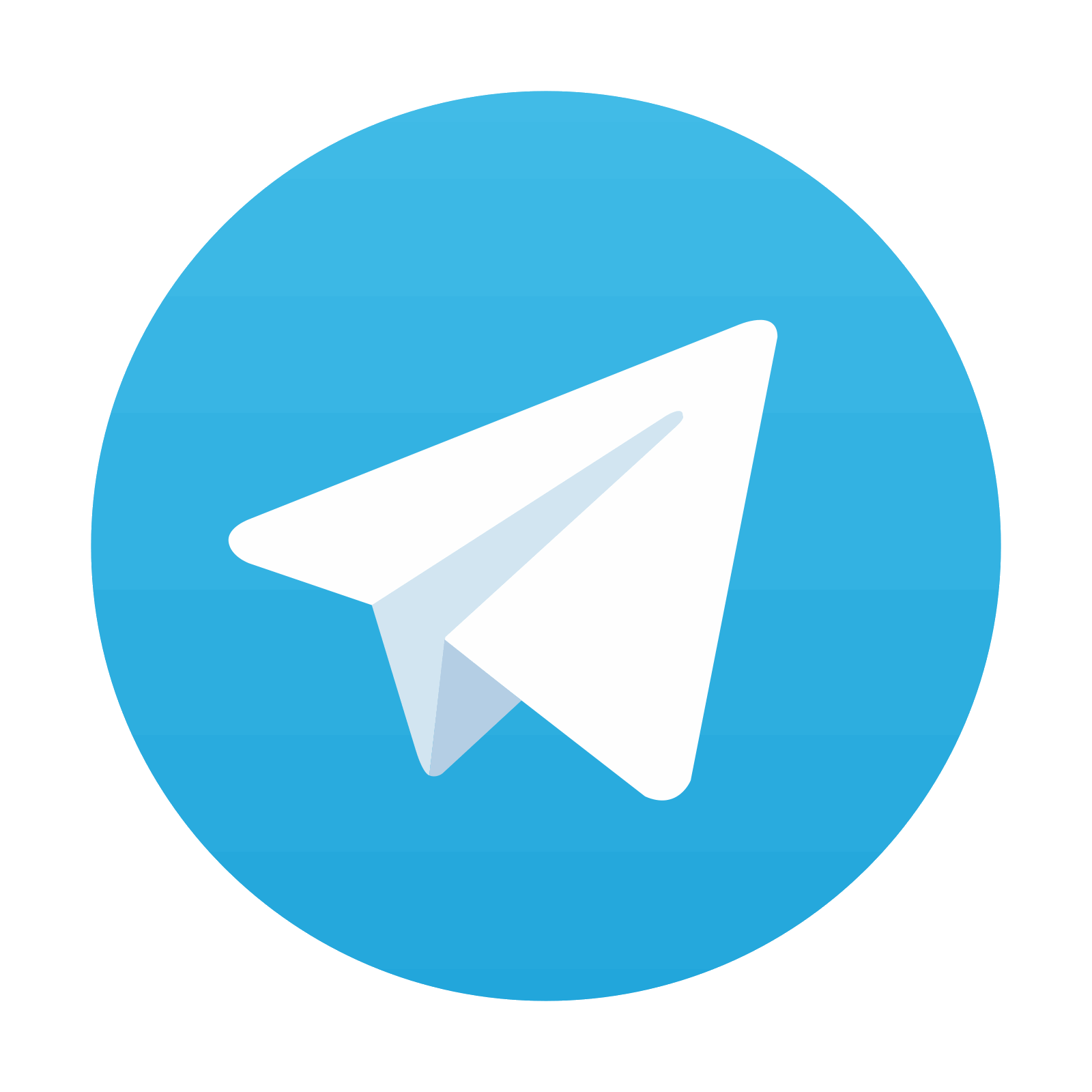
Stay updated, free articles. Join our Telegram channel
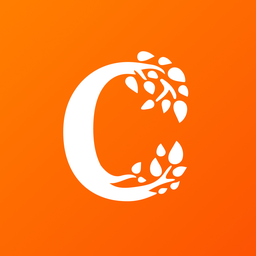
Full access? Get Clinical Tree
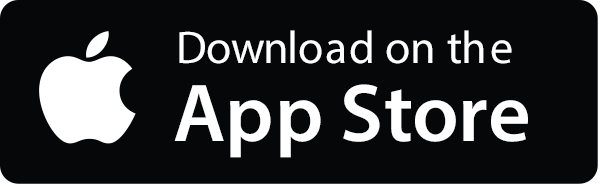
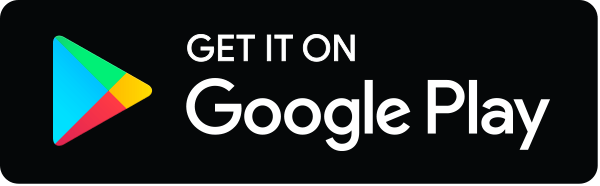