Charlotte Sinclair, Helen L. Birch, Roger K.W. Smith and Allen E. Goodship The horse has not only evolved to become a high-speed animal, but has also been the subject of selective breeding as an elite animal athlete. The Thoroughbred racehorse is a prime example of selection for speed. However, it has been suggested that this selection process has reached its limit as classic race speeds in Thoroughbred horses have not increased in the last 40–60 years.1 The Thoroughbred has arisen from a somewhat limited gene pool and this may have resulted in a plateau of performance2 in contrast to the performance of human athletes in which records are broken almost year on year. However, an alternative explanation is that training methods to condition racehorses have not developed to optimize the capacity of the adaptive responses of the musculoskeletal system. In human athletics, the application of sports science has undoubtedly contributed to the enhancement of performances over recent years. Equine sports science is not yet developed or applied to the same extent. In addition, many training systems continue to be based on empirical and traditional methods. There are many factors that influence the risk of fractures in horses. The majority of research has focused on racehorses. In racehorses, the type of surface is influential on the type of fracture that occurs. For example, on all-weather and dirt tracks, bi-axial proximal sesamoid bone fractures are most common, whereas in horses jumping on turf tracks, lateral condylar fractures of the third metacarpal bone are most common, and in flat turf races, proximal phalanx fractures are most common.3 In the UK, the catastrophic fracture rate per 1000 starts was 0.33 in flat races, 1.4 in hurdling, and 2.3 in steeple chasing.3 Track conditions have also been shown to affect the risk of fracture with risk increasing on turf as the conditions become softer, and on dirt tracks the risk increased when the tracks became ‘muddier’.4 However, conversely others found that risk of fracture increased with firmer turf.5 In racehorses in training, the risk of non-traumatic (i.e. spontaneous) fracture in horses that are in flat race training was 1.15 per 100 horse months in training and 1.5 in national hunt (jump) training. An increased risk of fracture was found in horses that had more days in training following a 60 day non-racing period (i.e. lay-off) and the distance exercised in the two months prior to fracture were correlated with increased risk of condylar fractures. The odds of condylar fracture increased by 0.3% per day for every additional day that the horse was in training since the 60 day non-racing period.3 The risk of condylar fracture increased by 4% for each extra furlong that the horse was exercised at a ‘fast pace’. Horses that suffered fractures were those that had had been in training for longer, had higher exercise intensities and completed more races compared to controls.3 A further study showed that horses were at increased risk of fracture if they either exceeded 44 km at canter (<14 m/s) or 6 km at gallop (>14 m/sec) in a 30 day period. Increasing the distance cantered up to 50 km in a 30 day period further increased the risk of tibial and pelvic stress fractures. Distal limb fracture has been shown to be at higher risk in horses that do no fast work compared to those doing 4–10 furlongs of fast work per week. Hence, short periods of high intensity exercise may be protective against fracture by stimulating appropriate adaptation of the bone. A 30–90 day rest period has been shown to be detrimental. However, interpreting rest periods is difficult as the horse may have been rested due to an existing injury that increases the risk of future injury, or the lack of stimulation to the bone may be responsible for increasing osteoclastic activity and thus weakening the bone.3 Also the training protocol following a rest period may influence adaptation and related fracture risk. The conformation of the individual horse has shown to influence predisposition to fractures in Thoroughbred racehorses. For example long pasterns are associated with increased risk of forelimb fracture, and carpal valgus decreases the risk of carpal fracture.6 In racehorses in training, gender and age were not shown to significantly affect fracture rate, whereas there was a significant difference in risk between trainers. The overall incidence of fracture for horses in National Hunt training was 1.1/100 horse months.7 Foals born to primiparous mares are at less risk of fracture than those of multiparous mares and the rate of fracture also decreased with increasing dam age.8 Thus early skeletal development can potentially affect the risk of fracture later in life. There is less documentation of the incidence of fractures in other equestrian disciplines. In event horses, a study demonstrated that in over 60 000 cross-country starts during ‘eventing’ there were 0.12 per 1000 starts of fracture or ‘bone injury’ recorded which is dramatically lower than those described above for racing.9 The equine skeleton is a refinement of the basic mammalian pattern. The horse is an unguligrade (hoofed) animal and a member of the order Perissodactyla (odd toed), standing on the hoof of the third digit. The major muscle masses of the limbs are positioned proximally to reduce the energy required to move the limb as it swings backward and forward in cursorial locomotion. Bone mass is also minimized at the distal extremities and safety margins decrease toward the distal extremity of the limbs, which may explain why the incidence of fractures in racehorses is higher in the distal bones than those located proximally.10 Selection for high-speed gait is also reflected in the morphology of the equine skeleton. The lever arms at the elbow and hock result in small muscle contractions producing fast extensive movement of the lower limb segments. These skeletal refinements in the elite equine athlete are basically a fine tuning of the general pattern. Analysis of skeletal conformation has been used to assess the level of performance in several different types of horse. Elite show jumpers and dressage horses were found to have larger hock angles and more sloping scapulas than other horses11, while another study found that in Quarter Horses the ability to generate greater torque at the hip and stifle joint was due to a greater muscle physiological cross-sectional area rather than longer moment arms.12 However, these systems have not yet been perfected to identify potential winners at an early age. Interestingly, a recent study of the skeleton of the great racehorse Eclipse has shown that his skeleton was ‘average’ in all respects.13 Hydroxyapatite consists of predominantly calcium and phosphate in a hexagonal crystal system and has the formula Ca10(PO4)6(OH)2. The hydroxyl ion can be replaced by fluoride, chloride or carbonate. The deposition of calcium and phosphate into hydroxyapatite occurs initially by nucleation of extracellular, lipid bilayer-enclosed microstructures (matrix vesicles) released by cells but ultimately requires collagen fibrils.14 The normal process of mineral precipitation is primarily dependent on extracellular phosphate concentration, with calcium playing a secondary role.15 Mineralization is inhibited by molecular crystal inhibitors such as pyrophosphate (PPi) which binds to the hydroxyapatite crystals preventing phosphate ion incorporation.16 Inactivation of tissue nonspecific alkaline phosphatase (TNAP); an enzyme that is able to hydrolyse PPi, reduces tissue mineralization.14 Other inhibitors of nucleation include matrix Gla protein (MGP) and osteocalcin, both calcium binding proteins.15 Mineralization depends on the balance between inhibitors and promoters of crystal formation. The organic matrix is predominantly (>90%) type I collagen together with a small proportion of minor collagens including types III, V, XI and XIII and non-collagenous proteins. Type I collagen is a fibril forming collagen. The collagen molecule, known as tropocollagen, is formed from three polypeptide chains. Two identical α1 polypeptide chains and one α2 chain form a right handed triple helix. The initial collagen molecule is synthesized as a procollagen precursor and the propeptides are cleaved from the ends of the molecule before collagen is able to assemble into fibrils. A more detailed description of the collagen molecule and fibrillogensis is given in Chapter 9. The non-collagenous proteins make up <10% of the organic component. Proteoglycans form about 10% of the non-collagenous ECM protein. Most of the proteoglycans in immature bone are large chondroitin sulphate containing proteoglycans, as in cartilage, but as the bone matures these are replaced with the small proteoglycans, decorin and biglycan. Biglycan and decorin associate with collagen fibrils and are therefore implicated in control of fibril diameters and mineral deposition in bone17 in addition to binding growth factors such as TGF-β.18 The γ-carboxyglutamic acid containing proteins, osteocalcin (OCN) and matrix Gla protein (MGP), are present in bone and are inhibitors of mineralization. OCN is a bone specific protein while MGP is present in most tissues19 and may play a role in prevention of extra-osseous mineralization.15 The glycoproteins osteonectin, fibronectin and thrombospondin are all found in bone. Osteonectin forms ~ 15% of the non-collagenous protein and is thought to be a nucleator in collagen-mediated mineralization.20 Fibronectin is involved in cell adhesion and may also be involved in mechano-signaling.21 Thrombospondin promotes bone formation by bringing matrix components closer together.18 The sialoproteins (bone sialoprotein and osteopontin) form about 15% of non-collagenous ECM protein.18 Bone sialoprotein promotes cell attachment, is osteoconductive (encourages bone deposition), osteoinductive (promotes recruitment and stimulation of bone forming cells)22 and plays a role as a nucleator of mineralization.23 Osteopontin, expressed by bone cells, chondrocytes and fibroblasts may be involved in recruitment of cells for fracture healing and remodeling of bone.24 α2 Heremans-Schmid glycoprotein (AHSG) accounts for about 20% of the non-collagenous ECM protein. However AHSG is synthesized by the liver and 40% localizes to bone.25 AHSG is a chemo attractant for monocytes and may serve in the recruitment of osteoclast precursors.26 These cells are derived from local lining cells. Osteoblast differentiation results from activation of β-catenin through Wnt signaling by the Wnt co-receptors, low-density lipoprotein receptor-related proteins 5 and 6 (LRP5, LRP6) and Frizzled.27 The flattened mononuclear cells become plump when activated and synthesize bone matrix in the form of osteoid (Fig. 8.1). The osteoid then becomes mineralized over a period of weeks to form bone matrix. In rapidly forming surfaces some osteoblasts are entrapped in their own matrix and these then become osteocytes (Fig. 8.2). The osteoblasts communicate with osteoclasts and enable activation of osteoclasts to allow bone resorption. Osteoblasts also produce colony-stimulating factor that increases numbers of pre-osteoclasts from mononuclear precursors in the bone marrow and also osteoclast activation factor that activates the pre-osteoclasts and initiates resorption of bone matrix (Fig. 8.3). These cells are derived from circulating monocytes. They are multinucleate cells (Fig. 8.3) which, when activated, reside on a bone surface with a ruffled border to isolate the local environment between the cell and the bone surface. The perimeter of the cell membrane forms a seal against the underlying bone involving adhesion molecules, the integrins to isolate the local environment beneath the cell which is then lowered in pH by an active proton pump generating hydrogen ions.28 The pH falls to around 2–3 and the bone matrix and embedded osteocytes are resorbed, forming a resorption pit, and the resorption products are trafficked through the cell.29 This process is regulated by the osteoblasts (see below), which in turn communicate with the third population of cell; the osteocytes. The bone-resorbing osteoclasts are also influenced directly by some specific hormones such as calcitonin. These cells arise from osteoblasts that become trapped in the bone matrix within lacunae. They are cells with many long cytoplasmic processes within small tunnels in the matrix called canaliculi (Fig. 8.2). Processes from adjacent cells connect by means of gap junctions allowing cell-to-cell communication. Work by Skerry and co-workers has identified that the neurotransmitter glutamate is involved in osteocyte cell signaling.30 This transmitter also operates in the central nervous system, where complex interneuronal signaling occurs. The presence of such a signaling mechanism among osteocyte bone cells provides supporting evidence that this population of cells plays a role in the overall perception of mechanical environment on a bone and co-ordinates an appropriate response to ensure optimal bone size and architecture. The osteocytes and their cell processes are surrounded by extracellular fluid. The mechanical loading of a bone results in deformation and movement of this extracellular fluid within the matrix around the cells. Extracellular fluid contains ions and the movement of this ionic fluid with respect to the charged surfaces of the matrix induces electrical potentials. These electrical charges are referred to as ‘streaming potentials’ which are also thought to influence the cell activity and provide a putative mechanotransduction pathway.31, 32 The gap junctions linking cell communication are also modulated in number by mechanical loading of bone and thus may play a role in regulation of bone form in response to functional demands.33 Quiescent bone surfaces are covered by lining cells which have the capacity to respond to both mechanical and biological signals and are activated to change shape into plump, metabolically active osteoblasts. These cells are found in the osteogenic layer of the periosteum or endosteum; these membranes comprise a deep cellular layer and a more superficial fibrous layer (Fig. 8.4). Some pluripotent cells have been demonstrated in the osteogenic cellular layer and these have been shown to have the capacity to differentiate into other connective tissues such as cartilage. There is currently considerable interest in the various types of pluripotent cells in the adult as a source of ‘stem’ cells that play a role in tissue regeneration. These quiescent lining cells are activated by mechanical or hormonal stimuli to generate a bone-forming front of metabolically functional osteoblasts involved in a modeling or remodeling process to maintain or restructure the matrix. The bone lining cells are thought to have a specific role in coupling bone resorption to bone formation34 and it has been suggested that this is by physically defining bone remodeling compartments.35 The activity of bone producing cells and bone resorbing cells must be balanced under normal conditions to maintain the integrity of the skeleton. The coupling of bone resorption and subsequent bone formation has been shown to involve a receptor on the osteoblast cell membrane known as receptor activator of nuclear factor κβ ligand (RANKL) which binds to RANK present on the surface of pre-osteoclasts and induces activation of the intracellular cascades to activate the osteoclasts. The RANKL can also bind to a protein called osteoprotegerin (OPG), which is stimulated by canonical Wnt signaling.36 OPG prevents binding with and activation of osteoclasts. Thus a regulation of coupling of these cells and associated amounts of bone resorption and formation is effected by this system (Fig. 8.5).37, 38 More recent work has shown that RANKL is also expressed by mature osteocytes and this expression is necessary for normal bone structure39, 40 as deletion of the RANKL gene in mice results in excess bone formation. It is not clear at present how osteocytes embedded within the matrix make contact with osteoclast precursor cells.41 Factors that increase RANKL expression are important and, in less mature osteoblasts, include parathyroid hormone (PTH), parathyroid hormone-related protein (PTHrP), 1,25-dihydroxyvitamin D3 and oncostatin M.41 In mature osteocytes, apoptosis42 and the protein sclerostin may stimulate RANKL production.43 The osteoblasts can also secrete collagenase, an enzyme that removes the surface layer of osteoid, unmineralized bone matrix, on bone surfaces and allows osteoclasts access to the bone matrix. Hormonal influences on calcium metabolism and bone resorption act indirectly on receptors on the osteoblast which in turn regulates osteoclast recruitment and activity. Thus the osteoblast is central to the control of the bone modeling and remodeling process. Boyde et al used in vitro systems to measure bone resorption activity of osteoclasts and also to show the interactions between osteoblasts and osteoclasts.44 The pro-inflammatory cytokine interleukin-33 (IL-33) is a newly identified factor produced by osteoblasts that inhibits osteoclast formation in vitro.45, 46 A study has demonstrated that mice deficient in the IL-33 receptor demonstrated increased osteoclast formation and low bone mass.46 The regulation of bone resorption and bone formation also relies on coupling factors released from osteoclasts that influence the bone forming osteoblast cells. Examples are cardiotrophin-1 that stimulates bone formation47 and the recently identified Semaphorin 4D which inhibits bone formation in female mice.48, 49 Furthermore, factors released by osteocytes can influence osteoblast activity. Sclerostin, a protein released by osteocytes, is an inhibitor of bone formation and is of interest because of its potential therapeutic potential in treating osteoporosis in humans. However sclerostin mRNA and protein has been identified in other non-bone sites41 including the adult human aortic intima41 where it is suspected to inhibit aortic calcification. Sclerostin is up-regulated by calcitonin50 and inhibited by factors that stimulate bone formation such as prostaglandin E251, oncostatin M and cardiotrophin-1.52 As a composite material, bone matrix is anisotropic and the architecture of the matrix in relation to the osteocytes forms the basic unit of structure, termed the lamella. The architecture of the lamellae can be observed under polarized light microscopy as the collagen is a birefringent material. The architecture of these bone lamellae in different geometrical arrangements forms the basis of the different histological types of bone. Concentric circumferential lamellae form osteons and when these are formed in the original development of the bone they are termed ‘primary osteons’. A similar architecture is also seen in the secondary osteons that are formed to repair damage such as micro-cracks or infill porosities within the cortex. Lamellae formed around vascular networks or plexi result in laminar or the less regular plexiform bone often seen in ungulates like the horse (Fig. 8.6). This type of bone allows very rapid increases in cross-sectional area with later consolidation.10 Lamellar bone can also form circumferential lamella on the entire periosteal and endosteal surfaces of individual bones (Fig. 8.7). In embryological development and in the early stages of fracture repair a rapidly forming bone with irregular lamellae, coarse collagen fibers and large osteocyte lacunae is seen. This is called ‘woven’ bone and is rapidly remodeled to the various types of organized lamellar bone, such as primary and secondary osteonal bone, previously termed ‘Haversian’ bone (Fig. 8.8). Bone morphology has been related to the mechanical loading requirements of different specific bones. For example, the orientation of collagen fibers in the cranial and caudal cortices of the radius reflects the mechanical requirements of this bone. Strain gauge studies have shown that this bone is loaded in both compression and bending with principal tensile strains aligned with the long axis of the bone in the cranial cortex and principal compressive strains aligned to the long axis in the caudal cortex.53 The arrangement of collagen fibers in relation to this pattern of loading has been demonstrated by Riggs et al.54 This has been supported by a comprehensive analysis of matrix morphology in the equine radius55, in which the analysis of primary bone and bone within secondary osteones of the cranial and caudal cortices of the radius were arranged appropriately to optimize for the pattern of functional loading. This study also confirmed a predominant longitudinal arrangement of collagen fibers in the cranial cortex of this bone, to resist the functional tensile strains at this location. Any consistent changes in the magnitude and pattern of loading induce a modeling response in which the bone cell activity will modify the matrix to maintain the optimization of the overall bone architecture in relation to the new prevailing loading conditions. Matrix, and embedded osteocytes, can be removed by osteoclasts and new matrix formed by osteoblasts. This coupled cellular activity allows bone as both a material and structure to be changed in terms of mass and distribution throughout life. The material properties of bone matrix as a composite material vary and are largely related to the degree of mineralization, particularly with respect to modulus of elasticity. Bone such as the tympanic bulla, with a very high mineral volume fraction and density, has a high modulus eminently suitable for conduction of sound, whereas deer antler is low in mineral content and has a low modulus, again appropriate to avoid fracture in its role in fighting. Bone matrix is an anisotropic brittle elastic material and the elastic modulus is related to the level of mineralization. The influence of mineralization on material properties has also been evaluated during growth and maturation. In immature horses the level of mineralization is low and increases to a higher level with maturation to adult equine bone. With development and increased mineralization there is a significant correlation with changes in mechanical properties; it is suggested that such changes may be measured non-invasively using ultrasound transmission systems. This would provide the potential to monitor material properties of bone during growth and possibly during training.56 More recently bone microindentation testing has been used to give a direct measure of bone mechanical properties at the tissue level.57 This technique involves applying a number of cycles of load to the bone using a very small probe which is capable of passing through the skin and can be used in vivo. The probe creates microscopic fractures giving information about a bone’s ability to resist macroscopic failure. Bone tissue as a material contributes to the mechanical characteristics of the individual skeletal elements. The architectural arrangement of the material is predominantly related to the general functional requirements of the particular skeletal elements. In the long bones the diaphysis is tubular to resist the functional loading patterns of bending and torsion. In engineering terms, these loading patterns can be sustained with maximum strength and minimal material by tubular structures with the material distributed at a distance from the neutral axis. For example, if a solid beam is subjected to bending, one surface will experience tensile stresses and the opposite surface compression stresses. At some plane between these two surfaces there will be neither tensile nor compressive stresses (the neutral axis), thus no material is required in this region. When bone tissue organizes into the components of the skeleton, its structure is based on optimization of energetic efficiency. Thus to minimize the mass of material, bone is not present in the medullary cavity (Fig. 8.9). The relative thickness of the cortices also reflects the loading patterns of a particular bone. These changes may be measured using radiographic and ultrasound transmission techniques.58 At the extremities of the long bones and in short bones the functional loads are predominantly those of axial compression. This is reflected in a different architecture with a thin cortical shell supported by internal cancellous bone (Fig. 8.10). The plates and rods of bone are termed trabecula and formed of aligned bone lamellae (Fig. 8.11). These rods and plates are not randomly arranged but strategically placed in relation to the trajectories of principal compressive and tensile stresses. In the cancellous bone of the epiphyses, underlying the articular surfaces of the synovial joints, the trabecula are orthogonal (perpendicular) to the articular surface. This architecture of the plates and rods again allows the use of minimal material to provide maximum strength and minimize energy requirements for locomotion. In short bones that are loaded predominantly in compression, the internal structure is of strategically arranged bony trabecula. Some short bones, such as the calcaneus, are subjected to bending moments. The internal cancellous architecture reflects this with arcades of trabecular bone arranged orthogonally to resist the principal compressive and tensile stresses. Nature reflects the calculated stress-related bracing seen in some engineering structures such as the Fairbairn crane in which the loading and principal stresses resemble those of the femur (Fig. 8.12). Adult bone form is determined by a combination of genetic and environmental influences. Fell demonstrated that certain anatomic features of the developing skeleton are formed as a consequence of inherent genetic control whereas others require a functional loading to form.59 An elegant study by Chalmers investigated the development of a mouse femur implanted into the spleen when at the cartilage anlage (precursor) stage of development.60 This cartilaginous bone precursor developed into a bone with the basic form of the femur but lacked the architectural refinements seen in the normal adult mouse femur. The refinements, such as a waisted femoral neck, cortical thickness and trabecular architecture, are induced as a consequence of functional loading and are determined by the prevailing loading conditions (Fig. 8.13). There is a dynamic interaction between the loads imposed on the skeleton and the morphology of the bones at any point in time throughout life. It is therefore important to appreciate these interactions in order to condition the skeleton for the demands of athletic performance and to understand the modes and mechanisms of failure.
Skeletal physiology
Responses to exercise and training
Introduction
Role of the skeleton
Epidemiology of skeletal injuries
Design of the equine skeleton
Bone as a tissue
Bone matrix molecular composition
Bone mineral
Organic matrix
Bone cells
Osteoblasts
Osteoclasts
Osteocytes
Lining cells
Intercellular communication between bone cells
Bone ultra-structure
Material properties
Mechanical characteristics
Bone as a structure/organ
Skeletal physiology: Responses to exercise and training
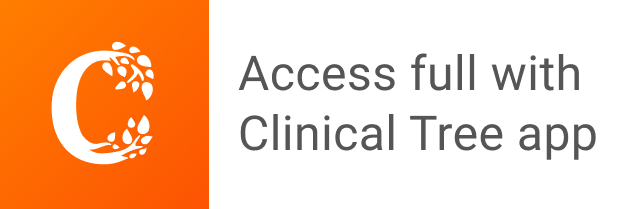