Chapter 8
Relaxation of the skeletal muscles
Relaxation using agents which act centrally
Utilizing drugs which have a peripheral action
Effector mechanisms (nicotinic acetylcholine receptors (nAChRs))
Non-depolarizing NMBs (competitive)
Strength and pattern of stimulation
Assessment of muscle contraction
Recognition of paralysis when a nerve stimulator is not used
Suxamethonium (succinylcholine)
Use of neuromuscular blocking drugs in veterinary anaesthesia
Essential requirements for use of NMBAs
Postoperative complications of neuromuscular blocking agents
Introduction
To relax skeletal muscles, it is necessary to abolish voluntary muscle contractions and modify the slight tension, which is the normal state (the ‘tone’ or ‘tonus’ of the muscle). Tone is maintained by many complex mechanisms but, briefly, it can be said that all result in the slow asynchronous discharge of impulses from cells in the ventral horn region of the spinal cord. This discharge gives rise to impulses in the motor neurons, which cause the muscle fibres to contract. Activity of these ventral horn cells is controlled by impulses from the higher centres (cerebrum, cerebellum, or medulla oblongata) exciting the motor neuron direct, or by impulses through the small motor nerve fibre system (the γ-efferents) which activate them indirectly via the stretch reflex arc. Movements controlled by the γ-fibre system are essentially directed towards governing the length of the muscle. In contrast, voluntary movement involving direct activity in the γ-fibres, results in muscle tension of a given magnitude. The small motor nerve fibre system is, like the motor fibres to the skeletal muscles themselves, a cholinergic one and any drug which can affect the neuromuscular junction may also interfere with the effect of the γ-fibres on the muscle spindles, thus reducing the afferent inflow from the muscle spindles to the brainstem. Thus, there is a possibility that a drug, which paralyses the γ-fibres and so reduces muscle spindle proprioceptive inflow to the higher centres, actually contributes to a sleep-like state.
Relaxation using agents which act centrally
Centrally acting drugs, such as guaiphenesin and benzodiazepines, produce muscle relaxation by selectively depressing the transmission of impulses at the internuncial neurons of the spinal cord, brainstem and subcortical regions of the brain, but the relaxation produced by them is seldom profound. Some degree of muscle relaxation can also be produced by the α2-adrenoceptor agonists.
Most anaesthetic agents cause decreased activity of ventral horn cells in the spinal cord, and thus muscle relaxation, although there is variation between agents in the degree of relaxation produced at a given ‘plane’ of anaesthesia. In general (with a few exceptions such as ether anaesthesia), profound muscle relaxation from general anaesthetic agents is obtained only when doses are such to produce a deep generalized depression of the whole central nervous system with accompanying dose-dependent depression of the cardiovascular and respiratory systems.
Utilizing drugs which have a peripheral action
Local analgesics injected so as to block the transmission of impulses in motor nerves result in profound muscle relaxation. This is demonstrated strikingly by paravertebral nerve block in cattle, and also following the use of epidural analgesia where the motor nerves are blocked as they leave the spinal cord. The major disadvantage is that the motor block may last longer than desired, and can be a problem at recovery in large animals. Combinations of local analgesia and general anaesthesia are used frequently. Peripheral nerve blocks such as brachial plexus, femoral or epidural blocks, provide excellent intraoperative analgesia, prevent wind-up in the dorsal horn cells of the spinal cord and can thus make a marked contribution to postoperative pain control, and have the added advantage of providing excellent muscle relaxation.
Using specific neuromuscular blocking agents
Modern neuromuscular blocking agents (NMBA) act at the neuromuscular junction, and by their use it is possible to produce quickly, and with certainty, any degree of muscle relaxation by neuromuscular block (NMB) without influencing the excitability and functioning of the central nervous and cardiovascular systems. They are commonly called ‘muscle relaxants’ or simply ‘relaxants’. They do not cross the blood–brain barrier and thereby have no direct action on the central nervous system.
In order that the mode of action of NMBAs be understood, it is essential that the phenomena which occur at the neuromuscular junction upon the arrival of an impulse in the motor nerve should be appreciated. The following brief review of neuromuscular transmission is concerned with those aspects that are of importance in anaesthesia. For a more detailed study, reference should be made to the standard texts of physiology, and the reviews cited below.
Theory of neuromuscular transmission
The neuromuscular junction is the most accessible of the synapses in the body to study and over the last 100 years very much has been revealed about it. Broadly, motor nerve endings contain vesicles of acetylcholine that are released as a result of an action potential in the nerve. After release, the acetylcholine diffuses across the synaptic gap (cleft) and interacts with nicotinic acetylcholine receptors (nAChRs) embedded in the postjunctional membrane of the muscle, directly opposite the sites of its release (the end plates), triggering the chain of events leading to muscle contraction. The acetylcholine is immediately broken down by acetylcholinesterase, which is situated in the synaptic cleft. However, within this synaptic transmission system, there are many processes involved which have been further elucidated or which still await explanation. Martyn et al. (2009) have reviewed recent knowledge of clinical relevance.
Release of acetylcholine
Acetylcholine is synthesized in the nerve cytoplasm by choline acetyl-O-transferase and must be pumped into vesicles (each containing a ‘quantum’) against its concentration gradient. The vesicles are situated close to the junctional surface. An action potential in the motor nerve triggers the opening of voltage-gated Ca2+-channels, calcium enters the nerve terminal, and the increase in intracellular calcium initiates a very rapid series of events terminating in the exocytotic release of quanta of acetylcholine into the synaptic cleft. Electron microscopy shows small protein particles in the active zone of the nerve endings between the vesicles, and it is thought that these are the Ca2+ channels. Abnormalities of some of these proteins have been identified as causing loss of function therefore potentially reduced acetylcholine release and muscle weakness. Ca2+ does not directly cause the vesicles to release their acetylcholine, but initiates their exocytosis via a complex protein fusion pathway (Jones, 1984; Lang & Jahn, 2008).
The amount of acetylcholine (number of quanta) released is very dependent on the concentration of ionized extracellular calcium, evidence suggesting that it is proportional to the fourth power of the change in Ca2+ concentration. Ca2+ continues to flow into the nerve terminal until the outflow of potassium returns the membrane potential to normal. The build-up of calcium within the nerve terminal explains the condition of ‘post-tetanic potentiation’ seen when the degree of effect a non-depolarizing NMBA is tested by stimulating the nerve with high tetanic frequencies (see ‘monitoring block’ below). Under these conditions, intracellular calcium increases, and remains higher than normal for some time. A stimulus applied during this time evokes a greater than normal release of acetylcholine which, in turn, antagonizes the non-depolarizing NMB.
A number of clinically relevant factors may interfere with this complex Ca2+ and/or protein mechanism. High extracellular concentrations of magnesium inhibit calcium entry into the cell, thus resulting in muscle weakness. Ca2+ antagonists, such as verapamil, may act by inhibiting protein combinations. In dogs, a dose of 1 mg/kg of verapamil has been shown to produce a significant interaction with the non-depolarizing agent, pancuronium, which persists long beyond the period of the calcium antagonist’s cardiac effects (Jones, 1984). Clostridial toxins exert their effects by damaging the proteins involved in the exocytotic process. Inhalation anaesthetics may be considered to be non-specific calcium antagonists and so potentiate neuromuscular blockade.
Acetylcholinesterase is an enzyme that is secreted by the muscle and is located in large amounts in the synaptic cleft of the end-plate. It hydrolyses acetylcholine (to choline and acetate) very rapidly, thus terminating the muscle contraction. Choline is taken up into the nerve terminal and used to synthesize more acetylcholine.
Effector mechanisms (nicotinic acetylcholine receptors (nAChRs))
Muscle contraction is initiated by acetylcholine released at the neuromuscular junction acting on the nAChRs on the end-plate at the neuromuscular junction. All nicotinic receptors so far isolated and characterized, function as cation channels, the activation of which causes a change in postjunctional membrane potential. Thus, they behave in a similar manner to receptors for other known chemical transmitters and belong to a family of closely related receptors (5-HT3 receptor, GABAA receptor, glycine receptor and kainate-type glutamate receptor). The common element in this receptor family is that the receptors consist of five glycosylated protein subunits of varying molecular type. Each of the subunits traverses the muscle membrane at the end-plate region and they are arranged to form the walls of an aqueous pore representing the ion channel through which mainly sodium and potassium ions flow to produce the single channel current measurable by the physiologists’ patch-clamping technique. The subunits have been designated α, β, γ, δ and ε with further subdivisions given numbers. In the postsynaptic nAChRs in the end-plates of mature skeletal muscle there are two α1, and one each of β, δ and ε. The neurotransmitter acetylcholine must bind to sites on two α subunits for the ion channel to open, produce the single channel current and initiate muscle contraction. Non-depolarizing NMBAs also attach to these sites so, if even one α subunit is occupied, acetylcholine cannot bind to two and open the channel. In fetal muscle, the ε is ‘replaced’ by a γ (hence termed the γ-subunit). Although some change from fetal to adult receptors occurs before birth, further conversion continues in the neonatal period. Mature receptors are situated at or very close to the neuromuscular junction, but the fetal γ-subunit may be more widely spread over the muscle surface. In clinical cases of immobility (e.g. by burns or denervation), mature receptors revert to the γ-subunit and also become more widely distributed over the muscle surface – this process commences within hours of immobility so can be a factor in many situations, although it may take days to complete. The clinical importance is the different responses of the two types of receptors to NMBAs. The γ-subunits show long opening times. This makes them very sensitive indeed to depolarizing agents, such as suxamethonium, not only to the relaxant effects, but also for the release of potassium. However, they are very resistant to non-depolarizing NMBAs. The changes in these receptor subtypes (and also other variations, for example α7, a further subtype thought to be of importance and currently under investigation) may explain unexpected differences in response to NMBAs of some patients.
Presynaptic nAChRs are present on the nerve terminal and have an influence on normal neuromuscular transmission. Although different types have been identified pharmacologically, their types in relation to action are not as clear as for the postsynaptic receptors. However, one acts as a positive feedback and responds to low concentrations of acetylcholine by facilitating its release at the end-plate while the second group (or effect), which contain α3β2 units, respond to a high concentration of acetylcholine and act in a negative feedback mechanism. This negative feedback, i.e. the increase in acetylcholine in the synaptic cleft leading to a decrease in further acetylcholine release, is thought to be the cause of post-tetanic fade and train of four (TOF) fade (see ‘Monitoring’ below) with non-depolarizing NMBAs. Suxamethonium does not stimulate the α3β2 receptor and with a single dose of this agent, TOF fade is not seen, although it will occur if there is a Phase II block (see below).
Other influences on the neuromuscular receptor
General anaesthetics, local analgesics and antibiotics are all potential causes of end-plate ion channel block.
A remarkable property known as ‘desensitization’ is displayed by the acetylcholine receptor of striated muscle and its associated systems. This was first described by Katz & Thesleff in 1957, and appears as the waning of a stimulant effect or development of repolarization (usually partial but under some circumstances complete) despite the continued presence of acetylcholine or some other depolarizing substance at the end-plate. The rate of this repolarization increases with the concentration of the drug and is faster when the extracellular concentration of Ca2+ is high. The extent of desensitization appears to vary between individuals of any one species. Acetylcholine has been shown to change the affinity of the receptor for certain blocking agents in a way which may be connected with the desensitization process.
Neuromuscular block
Consideration of the mechanisms of neuromuscular transmission outlined above suggests many ways in which the process may be modified to produce failure or block of transmission. There are two types of NMBAs, the non-depolarizing and the depolarizing, with very differing properties.
Non-depolarizing NMBs (competitive)
Non-depolarizing NMBs are also termed competitive, curare-like or antagonist NMBs. All non-depolarizing NMBAs block transmission by attaching to the nAChRs, where they themselves exert no direct action. However, even by blocking one α site, they prevent the acetylcholine released following motor nerve stimulation competitively from causing depolarization of the postsynaptic membrane. When the reduction in the degree of depolarization is such that a threshold depolarization of the membrane adjacent to the end-plate is not achieved, a neuromuscular block is present. As the effect is ‘all or none’ for each motor end-plate, what is seen in any particular muscle during this type of block represents a spectrum of these thresholds. For complete suppression of the motor response to occur, even the most resistant synapses must be blocked. In normal neuromuscular transmission, an excess of acetylcholine is produced by motor nerve stimulation. There also exist many more receptors than necessary for the production of a total increase in cation conductance required to trigger an action potential. This results in a substantial ‘safety factor’ (Jones, 1984). It has been shown that under certain conditions in cat tibialis muscle, four to five times as much acetylcholine is released as is needed for threshold action. Expressed in terms of receptors this means that 75–80% of the receptors must be occluded before the threshold is reached (Cookson & Paton, 1969). The existence of a safety factor means that the action of the drug is far from terminated at the time when transmission is apparently normal. There is likely to be considerable ‘subthreshold action’ which is only detectable when a tetanic stimulus is applied to the motor nerve or when the relaxant action of some other drug is potentiated.
As discussed above, acetylcholine is normally metabolized very rapidly by the acetylcholinesterase in the synaptic cleft, thus enabling the non-depolarizing NMBA to occupy the receptor. The use of an anticholinesterase, such as neostigmine, slows the metabolism of acetylcholine, increases the number of molecules available in the synaptic cleft, and therefore ‘shifts’ the competitive balance in favour of acetylcholine for occupancy of the receptors. This is the basis of reversal of NMB with neostigmine. However, it must be remembered that the increase in acetylcholine will also act on the muscarinic receptors causing a number of side effects and, as long as the NMBA remains in the synaptic cleft (time depending on the metabolism of individual NMBA), there will remain a degree of NMB.
Depolarizing NMB (non-competitive)
Suxamethonium (also termed succinylcholine) and decamethonium (now only used for research purposes) are examples of depolarizing NMBAs. Suxamethonium’s structure is that of two molecules of acetylcholine. It works first as an agonist, binding to the muscle nAChRs, depolarizing the end-plate, and therefore triggering muscle contraction, which usually manifests as generalized fasciculation. However, in contrast to acetylcholine that has a rapidly terminated action (ms), with suxamethonium the end-plate remains depolarized until the drug is removed from the plasma, by plasma (pseudo) cholinesterases. Relaxation occurs because the membrane remains depolarized and non-responsive to further stimulation (Burns & Paton, 1951). As discussed above, the depolarizing drugs do not affect the α3β2 receptor so, with a single relatively small dose, tetanic or TOF fade does not occur, or occurs only minimally. The depolarizing NMBA, however, will affect some other nAChRs and muscarinic acetylcholine receptors, with resultant multiple side effects. In this phase (Phase I) of a depolarizing NMB, use of anticholinesterase to increase available acetylcholine will augment the NMB.
The above simplistic description of the depolarizing NMB does not explain the condition known as Phase II (dual) block that occurs if a suxamethonium NMB is continued for longer than a single short acting dose. Lee (2009) has reviewed the practical aspects of this block, but the actual mechanism is not yet fully understood. The condition as it occurs with infusion of suxamethonium is that, following the standard Phase I block when there is minimal tetanic or TOF fade, there is then a period of tachyphylaxis, whereby a much higher dose of suxamethonium appears to be required before reaching the Phase II block. The Phase II block behaves as if it were a non-depolarizing block, there is tetanic and TOF fade, presumed to be through the same presynaptic feedback mechanism that occurs in the standard non-depolarizing NMBA block; in some but not all stages there can be partial (but not total) reversal by the use of anticholinesterase, and the block is massively increased by very small doses of non-depolarizing NMBAs. Once a Phase II block is established, the duration is very prolonged, and it is for this reason that suxamethonium is no longer used by infusion or incremental doses. However, a Phase II block can occur with a single dose if sufficiently large, and the anaesthetist should be aware of its existence.
Pattern of neuromuscular block
Sensitivity of muscles to neuromuscular block
The concept of sensitivity refers to the concentration of drug at the neuromuscular junction needed to produce a specific degree of blockade. Different muscles have different sensitivities to NMB. The standard often quoted order from most sensitive (lowest doses needed) onward is muscles of facial expression, jaw, tail, neck and distal limbs, proximal limbs, laryngeal/pharyngeal, abdominal wall, intercostal, then most resistant, the diaphragm. It is unlikely that this order is true for all animals and all NMBAs. Whilst Sarrafzadeh-Rezaei & Clutton (2009) found that, in anaesthetized dogs, the nasaolabialis muscles were more sensitive to a vecuronium block than the carpal flexor muscles, but also pointed out that all facial muscles do not have the same sensitivity. Clutton (2007), for small animals, disagrees with the classical list in that he places the diaphragm as being more sensitive than the limbs, although dogs may still breathe through actions of the intercostal muscles. There is considerable evidence that, in horses, the limb muscles are far more sensitive, at least to the onset of NMBAs, than are some of the superficial facial muscles often used for monitoring block (Hildebrand et al., 1986; Mosing et al., 2010).
There are several practical aspects to the potential differences in sensitivity. The first is to find a suitable site for monitoring the NMB by nerve stimulation (see ‘Monitoring of neuromuscular block’, below). If a very sensitive site is chosen, then a full block may be registered there, while the block of the muscles that the surgeon requires is not optimal. More importantly for recovery, monitoring at a very insensitive site might suggest good reversal of NMB when many important muscles are still at least partially paralysed. The other major use made of differences in muscle sensitivities is to attempt to use low dose NMBAs to achieve relaxation of some muscles while still enabling the animal to breathe spontaneously. Currently, this is most used in the dog in order to keep the eye central and still for ocular surgery. Although the dog may continue to breathe, there is likely to be diaphragmatic and costal muscle weakness that will impair ventilation, increase arterial carbon dioxide, and limit any possible ventilatory response to hypoxia. These authors strongly recommend that the dogs are artificially ventilated, if only to obtain the best ventilatory and cardiovascular conditions necessary for good intraocular surgery.
In human anaesthesia, there are many studies investigating the relative sensitivity of nerve/muscle groups which are used for monitoring NMB in relation to the diaphragm or to other muscles in which it is particularly important to avoid postanaesthetic residual block (e.g. the larynx). Similar investigations in veterinary medicine have been limited. Also, there is still little understanding of the mechanisms underlying the cause of differing muscle sensitivities. Among the mechanisms suggested as being responsible for differing responses are:
This is important in relation to onset of action, as NMB can only be produced when the drug binds with acetylcholine receptors at the neuromuscular junction (Donati, 1988). For the onset phase, concentration gradients between the plasma and the receptors are large and thus perfusion plays a major part in the development of neuromuscular block. During recovery from block, plasma concentration changes slowly with time, so that the concentration gradient between plasma and receptors on the different muscles is likely to be small. As a result, perfusion plays only a minor role, duration of blockade being determined mainly by plasma concentration and sensitivity of each muscle.
The motor innervation pattern of muscles, acetylcholinesterase activity and number and density of receptors at end-plate regions may all play a part, but it is not known exactly how these may contribute to muscle sensitivity to neuromuscular blocking drugs.
In goats, there is a direct association between time to spontaneous recovery from vecuronium or suxamethonium blockade and size of fibres in the diaphragm, posterior cricoarytenoideus, thyroarytenoideus and ulnaris lateralis muscles (Ibebunjo & Hall, 1993). This evidence for influence of fibre size is supported by the fact that laryngeal and facial muscles contain very small fibres while larger fibres are found in the diaphragm and still larger ones in peripheral muscles, a rank order identical to the relative sensitivities of these muscles to neuromuscular block (Donati et al., 1990). Subsequent similar work (Ibebunjo et al., 1996) found that recovery was shortest in the laryngeal muscles and longest in the abdominal muscles, and that this correlated directly with fibre size and inversely with end-plate/fibre size ratio. They concluded that, in the goat, recovery from NMB was shortest in muscles composed of small fibres with large end-plates. Incidentally, in this study, there was no difference in relative muscle sensitivity to depolarizing or non-depolarizing NMBAs.
Monitoring of neuromuscular block
Knowledge of the degree of NMB is necessary to ensure that the block is adequate for the procedures but, more importantly, to monitor recovery and to be as certain as possible that no residual NMB remains once the person/animal regains consciousness and is no longer being ventilated. In human anaesthesia, inadequate reversal of NMB leads, among other side effects, to inadequate ventilation, impaired hypoxic respiratory response, laryngeal weakness and airway obstruction (Fink & Hollmann, 2012). The same problems can be anticipated to occur in veterinary patients. In the authors’ previous experience when only long acting NMBAs were available and monitoring techniques were not, in dogs post-block laryngeal weakness could be a serious problem. In horses, ensuring that limb muscles have returned to normal strength is a prerequisite for a good quality recovery.
In many places, in human and veterinary anaesthesia, degree of NMB is assessed subjectively from the responses of the patient. However, the advent of monitoring by the response to peripheral nerve stimulation enables a more objective, and therefore much safer assessment of onset, depth and recovery from NMB.
Peripheral nerve stimulation
Simple peripheral nerve stimulators (Fig. 8.1) are readily available. However, when monitoring NMB by peripheral nerve stimulation, it is necessary consider the following:
Figure 8.1 This shows a relatively simple nerve stimulator, capable of delivering a range of different stimulation patterns, which is used to monitor neuromuscular block. The needles are placed over the ulnar nerve, approximately 2-4 cm apart. On stimulation, presence or absence of movement of the paw can be seen. The lead on the paw is, in fact, an ECG lead, but the position is where the sensor for acceleromyography would be placed. Photograph courtesy of Hatim IK Alibhai.
Site of stimulation
The requirement is a superficially placed motor nerve with easy access that innervates a muscle with contraction that can be observed/monitored, and the movement of which will not be detrimental to surgery. It is important to avoid accidental direct stimulation of the muscle. In humans, the site most used is the ulnar nerve to the adductor pollicis, movement of the thumb being observed/measured. As the NMB differs between different muscles, a specific measured result may differ depending on site. This was well demonstrated in a study in Shetland ponies (Mosing et al., 2010) in which the peroneal, the radial and the facial nerves (to the orbicularis orbis muscle) were stimulated, the response being measured by acceleromyography. Following injection of 0.6 mg/kg rocuronium, within the 5-minute measuring period before reversal with sugammadex (see Evoked recovery from NMB below), a full block did not occur in the orbicularis oculi, but did in the limb muscles. This finding is similar to those of previous studies in horses, where muscles stimulated by the peroneal nerve show greater sensitivity to NMBAs than muscles innervated by the facial nerve (Manley et al., 1983; Hildebrand & Arpin, 1988).
A number of different nerve/muscle combinations have been employed for assessment of NMB. In horses, the facial nerve (Fig. 8.2) can be palpated on the masseter muscle ventral to the lateral canthus of the eye and, when this is stimulated, produces easily visible contractions of the muscles of the lip and nostrils. The peroneal nerve can be stimulated as it crosses the head of the fibula to produce contractions of the digital extensor muscles (Fig 8.3; see also Fig. 8.7). It is advisable to restrain the hind leg when this nerve is stimulated even if no mechanical recording of the response is proposed. Mosing et al. (2010) describe location of the radial nerve in the non-dependent limb by palpation of the groove between the lateral extensor digitorum and the lateral ulnaris muscles, approximately 4 cm (in Shetland ponies) distal to the lateral tuberosity of the radius. In dogs, muscle responses to electrical stimulation of the ulnar, tibial, peroneal and facial nerves have been reported in many studies. The ulnar nerve is stimulated at its most superficial location on the medial aspect of the elbow and contraction involving the forepaw is assessed visually by palpation or by acceleromyography (see Fig. 8.1). The peroneal nerve is stimulated on the lateral aspect of the stifle and muscle twitch of the hind foot assessed; this site is particularly useful when the head end of the animal is covered, for example during ocular surgery. Peroneal nerve stimulation has been used in the assessment of neuromuscular block in cows, calves, llamas and pigs.
Strength and pattern of stimulation
The observed muscle response following stimulation of a peripheral nerve is only reliable when one electrical stimulus causes the nerve to depolarize, the same number of nerve fibres are stimulated each time, and direct stimulation of muscle fibres is avoided. After a nerve has been depolarized to produce an action potential, it is resistant to further stimulation for its refractory period of some 0.5–1.0 ms. Most clinically available peripheral nerve stimulators (see Fig. 8.1) will deliver all of the various stimulation patterns described below. These patterns and their responses have been well illustrated by Viby-Mogensen (2010). A supramaximal stimulus is essential to ensure that all the muscle fibres in the effector muscle contract. With the majority of stimulators, this is achieved at a ‘calibration’ test prior to administering the NMBA. This generally involves the anaesthetist increasing the current until the maximal muscle contraction is achieved, although some units can self-calibrate. In recent publications on NMB monitoring in horses, currents in the region of 40–60 mA have been employed (Mosing et al., 2010). Supramaximal stimulation can be achieved using surface or needle electrodes; paediatric ECG silver/silver chloride gel electrodes are excellent provided the underlying skin is properly prepared (clipping and cleaning with alcohol). However, in veterinary anaesthesia, needle electrodes are often preferred, especially in animals with thick skin. It is recommended that the negative electrode of the stimulator is placed distally, and skin temperature (for surface electrodes) should be kept >32°C as hypothermia increases electrical impedance (Fuchs-Buder et al., 2009).
A number of different ‘stimulation patterns’ are in use, each having advantages/disadvantages under different circumstances. It must be remembered that, as explained earlier (release of acetylcholine), ‘fade’ following multiple stimulations occurs only with a non-depolarizing or a Phase II block, and is not a feature of a Phase I depolarizing block. This is because at the early stages of recovery, a non-depolarizing block can be partly antagonized by an increase in acetylcholine. The classical theory is that tetanic or multiple stimulations decrease available acetylcholine, probably both by depleting the acetylcholine available for release but also by reducing further acetylcholine release through the negative feedback system. In contrast, a depolarizing block is not antagonized by acetylcholine; hence the absence of fade.
For all patterns, characteristics of the stimulation is usually the same, a monophasic impulse, duration 0.2 ms and rectangular wave form (Fuchs-Buder et al., 2009).
Single twitch stimulation at frequencies of 0.1–1 Hz has been used extensively to investigate effects of NBAs in human subjects. At a stimulus frequency greater than about 0.15 Hz, the response becomes progressively smaller (i.e. fade) owing to the presynaptic ‘feedback’ effects impairing further release of acetylcholine. Using a single stimulation, a ‘control’ twitch is needed prior to giving the NMBA for comparison of subsequent twitches.
While repetitive stimuli at a frequency of <0.05 Hz causes fade, there is also a condition known as the ‘staircase phenomenon’ whereby, in the absence of NMBAs, repetitive stimuli over time potentiate the mechanical response of the muscle, i.e. the twitch size increases, until a point in time where the response appears to stabilize. Both single twitches, 1 Hz every second for 10 minutes, and TOF (see below) pattern, 15-second intervals for 15 minutes, have been shown to cause this, while a tetanic stimulation prior to such stimulation reduced the effect (Kopman et al., 2001). Martin-Flores et al. (2011b) demonstrated that the ‘staircase’ also occurred in dogs, and that approximately 25 minutes of stimulation was required to obtain an acceptably stable baseline. The clinical importance of this phenomenon is that the anaesthetist must be aware that an increasing ‘baseline’ of twitch might be occurring throughout the early intraoperative period of monitoring and thus introduce a bias into the subsequent results.
The single twitch is used primarily to assess the speed on onset of NMB. It is of less use for monitoring recovery as it depends on comparison with the pre-NMB twitch, impossible to judge visually and even with measurement, such as acceleromyography, the ‘staircase effect’ may make the result unreliable.
Since its description by Ali et al. (1970), the TOF (four pulses at 2.0 Hz) has become the most popular of the methods available to the clinical anaesthetist to monitor competitive NMB. The method involves recording four twitch responses evoked in a muscle by supramaximal stimulation of its motor nerve at a rate of 2 Hz, with an interval of at least 10 seconds (15 seconds is more usually employed) between trains to avoid ‘fade’ between successive trains of stimuli.
With no NMB, all four twitches should be of equal height. Loss of the fourth response represents 75–80% NMB; loss of twitches 3, 2 and 1 represent 85%, 90% and 98–100% block, respectively. The twitch–height ratio, height (amplitude) of the fourth response (T4) divided by the height of the first (T1) is known as the TOF ratio (Fig. 8.4). With a complete NMB, the TOF ratio will be 0, as T1 will be 0; with complete recovery (or pre-block), the TOF ratio should be 1, i.e. the fourth twitch will be at the same height as the first. In human anaesthesia, it is considered that a TOF ratio of 0.7 means adequate diaphragmatic recovery, but it requires recovery to TOF of 0.9 for return of adequate laryngeal/pharyngeal function, and a TOF of 0.9 is now usually taken as the being acceptable level of recovery from NMB. In veterinary anaesthesia, there is no reason to accept less and, for large animals that require perfect muscle function for recovery, a TOF of 1 would be preferable.
Figure 8.4 Pattern of responses to TOF stimulation during recovery from non-depolorizing neuromuscular block. Twitch height calculated as percentage inhibition of first response to TOF stimulation.
Practically, the major advantage of the TOF is that for normal clinical use it is not necessary to establish a control response before the administration of the NMB. In veterinary anaesthesia, the TOF is now used routinely both clinically and in research studies to assess the recovery from NMB in a large number of species.
The DBS consists of two short lasting bursts of tetanus (2–4 pulses at 50 Hz) separated by 0.75 seconds. The interval of 0.75 seconds allows the muscle to relax completely between tetanic bursts, so that response to this pattern of stimulation is two single separated muscle contractions perceived as two twitches. The tetanic bursts fatigue the neuromuscular synapse more than two single twitches so that fade is exaggerated during the second burst. Several different combinations of tetanic stimulation have been used but the pattern known as DBS3,3, or DBS3,2 seem satisfactory (Fig. 8.5). The larger response to DBS means that the first response reappears just before that from TOF stimulation and the second response occurs slightly before the fourth response to TOF (Gill et al., 1990). A fading second response is comparable with TOF ratio <0.6 (Fuchs-Bader et al., 2009). DBS was designed to improve clinical detection of residual curarization because it is easier to compare visually or tactilely the strength of the two contractions to DBS than to compare the strength of the first and fourth contractions with TOF ratio.
Figure 8.5 Stimulation patterns and muscle response at various degrees of non-depolarizing neuromuscular block. The stimulation pattern shown is a TOF followed by a double burst. At partial recovery, the difference in the degree of ‘fade’ at partial recovery is more obvious with visual monitoring of movement with the double burst. From JørgenViby-Mogensen. 2010. Neuromuscular monitoring. In: Miller, R.D., Eriksson, L.I., Fleisher, L.A., et al. (Eds.), Miller’s Anesthesia, seventh ed. Churchill Livingstone Elsevier, Philadelphia, with permission.
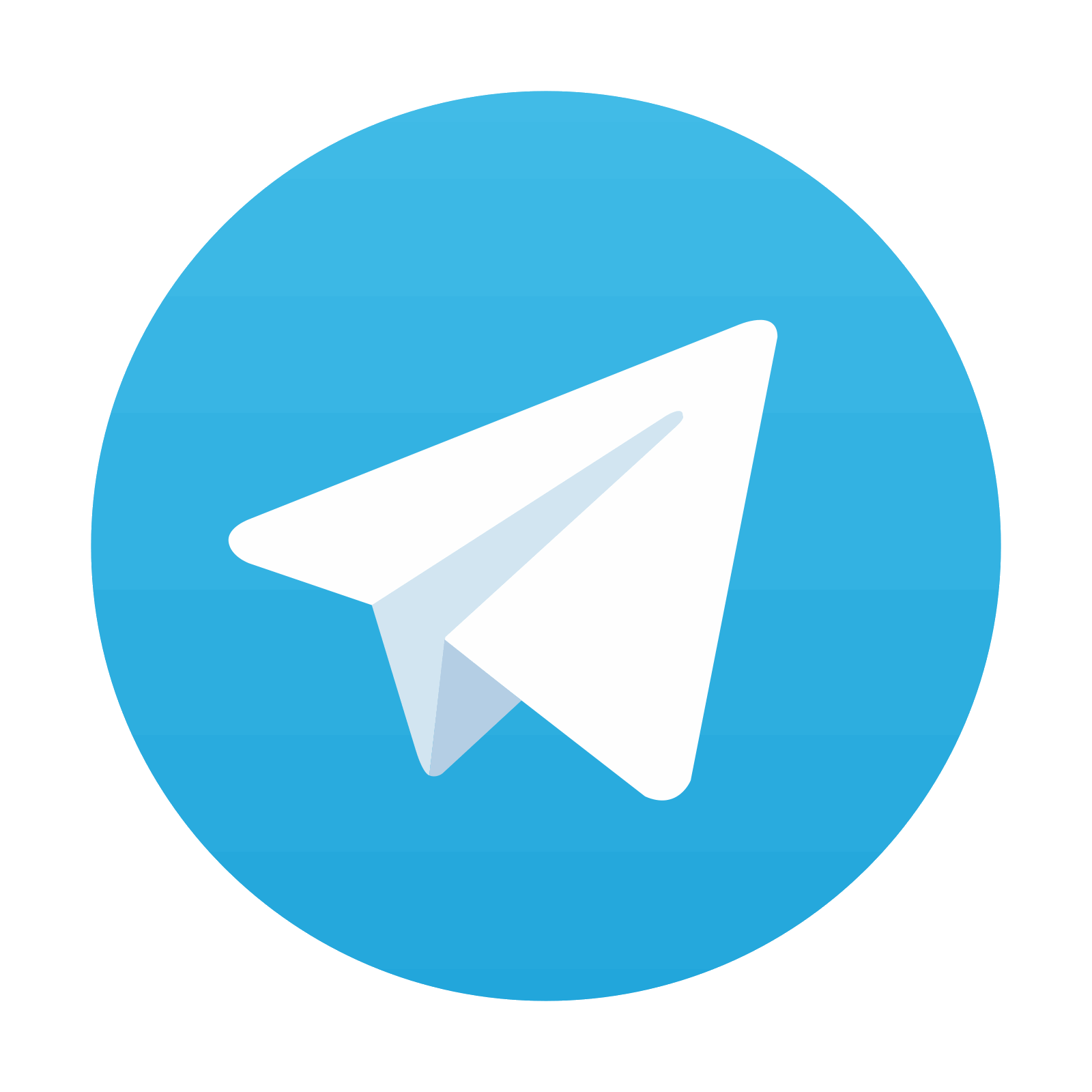
Stay updated, free articles. Join our Telegram channel
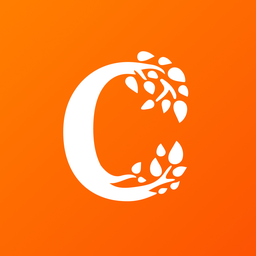
Full access? Get Clinical Tree
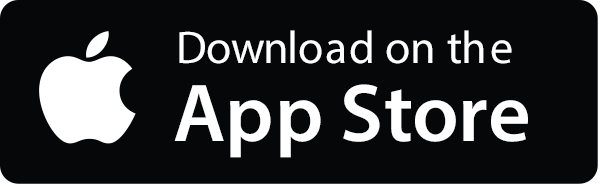
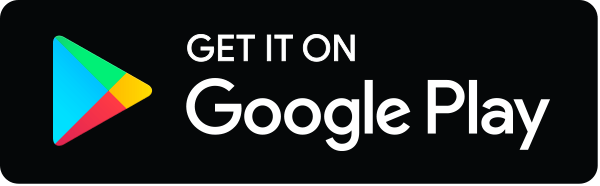
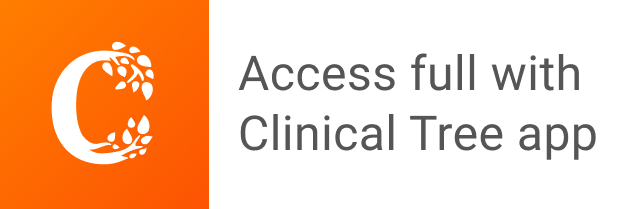