Chapter 9
Pulmonary gas exchange
Artificial ventilation of the lungs
Introduction
The techniques used to ventilate the lungs during anaesthesia usually involve endotracheal intubation, then periodic inflation of the lungs. The commonest method used in these circumstances is known as ‘intermittent positive pressure ventilation’ or IPPV. It is used widely in anaesthesia to counteract anaesthetic-induced respiratory depression, or whenever neuromuscular blocking agents have been used (see Chapter 8). It is also a routine technique in intensive care, but here more sophisticated methods of ventilation are required than for anaesthesia. There are differences between spontaneous breathing and IPPV, and IPPV when the thoracic cage is opened widely at thoracotomy from when it is intact. The anaesthetist must appreciate what these differences are if IPPV is to be correctly managed under all circumstances.
Spontaneous respiration
In a spontaneously breathing animal, active contraction of the inspiratory muscles lowers the normally subatmospheric intrapleural pressure still further by enlarging the relatively rigid thoracic cavity. The decrease in intrapleural pressure lowers the alveolar pressure (Fig. 9.1) so that a pressure gradient or driving force is set up between the exterior and the alveoli. This overcomes the airway resistance and air flows into the alveoli until, at the end of inspiration, the alveolar pressure becomes equal to the atmospheric pressure. During expiration the pressure gradient is reversed and air flows out of the alveoli.
Figure 9.1 Changes in lung volume, pleural and transpulmonary pressures during normal spontaneous breathing (diagrammatic only).
The transpulmonary pressure is a measure of the elastic forces which tend to collapse the lungs (Fig. 9.1). There is no one single intrapleural pressure; in the ventral parts of the chest it is just sufficient to keep the lungs expanded but because of the influence of gravity acting on the lungs, in the dorsal parts of the chest the intrapleural pressure should be much more below atmospheric. However, it is not at all certain how uniform the pressure on the pleural surface of the lung really is. The hilar forces, the buoyancy of the lung in the pleural cavity and the different shapes of the lung and chest wall are all possible sources of local pressure differences. Thus, it is customary to measure the intraoesophageal pressure as being representative of the mean intrapleural pressure (Fig. 9.2).
Figure 9.2 Pressure changes (A) at the mouth end of endotracheal tube, (B) in the thoracic oesophagus during spontaneous breathing and IPPV with closed chest (diagrammatic only).
The alveolar pressure changes generate airflow into and out of the lungs against a resistance in a way analagous to that stated by Ohm’s Law for electricity, where:
So that:
Airway resistance is largely influenced by the lung volume because the elastic recoil of lung parenchyma exerts traction on the pleural surfaces and walls of airways (holding them patent) when the lungs are inflated above residual volume. As the lungs are further inflated, elastic recoil pressure increases, thus further dilating the airways and decreasing resistance to air flow. This relationship between airway resistance and lung volume is hyperbolic in nature, as shown in Figure 9. 3. Airway resistance also depends on the nature of airflow through the airway. With a clear airway and a low gas flow rate, intrapulmonary flow is largely laminar (streamlined) and airway resistance is also low, but obstruction or a high flow velocity will give rise to turbulence and a greatly increased resistance. Measurement of airway resistance must be made when gas is flowing. During IPPV, when the chest wall is intact, resistance to expansion of the lungs is also offered by the chest wall which then contributes to the total respiratory resistance.
Figure 9.3 Resistance and volume curves together with lower airways conductance curves (Glaw). (1): Resistance curves; (2): (Glaw); (A): normal airway; (B): obstructed airway. Lehane et al., 1980, by permission of Oxford University Press.
Total respiratory resistance (Rrs) may be estimated by the application of an oscillating airflow to the airways with measurement of the resultant pressure and airflow changes. A technique was developed by Lehane et al. (1980) to measure airway resistance as a function of lung volume during a vital capacity manoeuvre and so to derive specific lower airways conductance, s.Glaw (conductance being the reciprocal of resistance) and the expiratory reserve volume (ERV). The method was modified by Watney et al. (1987, 1988) for use in anaesthetized and paralysed horses and dogs and it was demonstrated that, in ponies, xylazine, acepromazine, halothane and enflurane produce bronchodilation and a decrease in ERV while isoflurane appears to increase ERV. In dogs, it was concluded that both bronchoconstriction and changes in lung volume may be responsible for changes in airway resistance seen during hypoxia. During spontaneous breathing, changes in resistance may necessitate a great increase in the work of breathing. The effect of inhalation anaesthetics on total respiratory resistance in conscious horses was studied by Hall & Young (1992) who showed that halothane appeared to have no effect while enflurane and isoflurane seemed to increase it. In contrast in humans, neither isoflurane nor sevoflurane altered résistance, although desflurane at higher concentrations did cause an increase (Nyktari et al, 2011).
Resistance is not the only factor opposing movement of air in and out of the chest; a full analysis includes the effects of compliance and inertance. Adding the compliance and inertance forms the reactance and this can be combined with the resistance in one complex term called the ‘impedance’. If the impedance of the respiratory system is known then the resistance and reactance can be determined. A non-invasive method (Michaelson et al., 1975) that does not require patient cooperation has been adapted for use in conscious animals as described by Young and Hall (1989) for horses but it is difficult to use in anaesthetized, intubated animals because the impedance of the tube alone is much greater than that of a non-intubated animal. Small airways contribute little to the total lung resistance; although each one has a large individual resistance, there are large numbers in parallel so that the overall effect is small. This is important because small airway disease (which increases local resistance) is not detected by measurement of total airway resistance until the condition is well advanced.
Anaesthetic apparatus may afford resistance that is considerably higher than that offered by the animal’s respiratory tract. However, it is unlikely that moderate expiratory resistance will cause serious problems in spontaneously breathing animals and, indeed, positive end expiratory pressure (PEEP) (see PEEP and CPAP below) has many positive advantages. However, resistance increases the work of breathing, and common sense suggests that apparatus resistance should be kept to a minimum. Purchase (1965a,b) studied the resistance afforded by four closed breathing systems used in horses and cattle and in three, all of which had internal bores of 5 cm, found it to be of the order of 1 cmH2O (0.1 kPa) per 100L/min at flow rates of 600 L/min. The resistance of endotracheal tube connectors was relatively high in comparison with that of the remainder of the apparatus.
During a breathing cycle, mean intrathoracic pressure may be above or below atmospheric pressure as a result of apparatus resistance. For example, if the expiratory flow through a piece of apparatus with a high resistance is great enough to induce turbulence, while the inspiratory rate is low (as it often is in horses) so that during inspiration the flow is laminar, the mean intrathoracic pressure will be above atmospheric. Conversely, if the inspiratory flow rate is greater, there may be a subatmospheric mean intrathoracic pressure. Mean intrathoracic pressures above atmospheric reduce the effect of the thoracoabdominal pump for venous return, with subsequent cardiovascular effects. Large subatmospheric mean intrathoracic pressures may be equally dangerous, perhaps by producing pulmonary oedema, but probably more importantly by reducing lung volume. Trapping of gas in the lungs occurs more readily at low lung volumes and gas trapping produces widespread airway obstruction with serious impairment of respiratory function.
IPPV when the chest wall is intact
IPPV is applied easily during anaesthesia by rhythmical compression of the reservoir bag of a breathing circuit. This is most simply achieved by manual squeezing, but machines have been designed and built to relieve the anaesthetist of the bag-squeezing duty. If the bag is squeezed as the animal breathes in, the tidal volume may be augmented (‘assisted ventilation’). The increased ventilation produced results in ‘washout’ of CO2 and the PaCO2 falls below the threshold for stimulating the respiratory centre so that spontaneous breathing movements cease and the anaesthetist can impose whatever respiratory rhythm is required – ‘controlled ventilation’. A machine provides the most efficient means of ventilating the lungs for prolonged periods, but to use it properly or even to squeeze a bag correctly, it is necessary to understand the principles underlying IPPV and under what circumstances any possible harmful effects may arise.
Compliance has been defined in many ways but the simplest definition is that it is the volume change produced by unit pressure change (δV/δP). Compliance shows hysteresis (Fig. 9.4). It is changes in surfactant which seem to be responsible; airway resistance and tissue viscosity play only a small part. Ideally, measurements needed for the calculation necessary to obtain this value should be made when no air is flowing into or out of the lungs (i.e. at the end of inspiration). Compliance measures cannot be compared unless related to a lung volume such as the functional residual capacity (FRC). Unfortunately, measurement of FRC is not a simple procedure and such measurements of compliance as have been made in animals have often omitted this refinement.
Figure 9.4 Total thoracic compliance curves showing hysteresis. The compliance can be altered by a number of factors: (A) the lung compliance is reduced by lack of surfactant (respiratory distress syndrome), reduction of elastic tissue in the lungs (as in emphysema), and fibrosis or scar tissue; (B) the chest wall compliance is altered by obesity and splinting of the diaphragm by abdominal disorders.
As commonly measured, compliance has two components and compliance values can be found for both the lungs themselves and the thoracic cage. When the chest wall is not intact, the total compliance measured approaches that of the lungs alone. It might seem that methods using high airway pressures to inflate the lungs might be safe if, when compliance is reduced, it is the thoracic cage itself which is uncompliant, because alveoli only rupture when overdistended. However, decreased compliance of the lungs themselves is apt to be non-uniform; an airway pressure which produces little ventilation of some regions may overdistend and even rupture alveoli in other regions. During anaesthesia, compliance may be altered by assistants resting their weight on the chest, by the use of retractors and by the degree of muscle relaxation.
Airway resistance has to be overcome to deliver gas to the alveoli at inspiration and to expel it during expiration. Resistance during anaesthesia is increased by the resistance of apparatus used, such as endotracheal tubes. Animals with pulmonary disease may also have increased airway resistance so that it is necessary to allow a more prolonged expiratory period if the lungs are to deflate to FRC. If this is not done, lung volume will be greater at the start of the next inspiration and there will be a steady increase in FRC until the retractive forces of the lung, which increase with increase in lung volume, become sufficient to empty the lungs to a new FRC in the time available and the inspiratory and expiratory tidal volumes become normally related. While conscious, the animal with expiratory obstruction empties its lungs by active expiratory movements but, when anaesthetized and paralysed or made otherwise apnoeic, expiration may become passive and, consequently, of longer duration. The pattern of IPPV used must make allowance for this, and large tidal volumes should be delivered with long expiratory pauses between each inspiration to allow the chest to return to its original resting position.
The induction of general anaesthesia is usually associated with an increase in resistance due to a decrease in lung volume but this may be countered to some extent by bronchodilation, depending on the agents given.
The most obvious effect of IPPV when the chest is closed is on the circulatory system. During spontaneous breathing, by lowering intrathoracic pressure, inspiration augments the venous return to the heart; in many animals, as can often be seen on the arterial blood pressure trace, there are indications that a increased stroke volume is produced. During IPPV, however, intrathoracic pressure rises during inspiration, blood is dammed back from the thorax, venous return and stroke volume decrease; blood flows freely into the thoracic vessels during the expiratory period. Fortunately, by causing distension of veins this damming back of blood during inspiration produces a reflex increase in venous tone which, in normal animals, appears to compensate for the changed intrathoracic conditions during the inspiratory period and restores the venous return towards normality. Obviously, the extent to which an increase in venous tone can compensate will depend on the degree of venomotor integrity (which can be affected by drugs), the blood volume, the magnitude of the intrathoracic pressure rise and its duration.
The magnitude and duration of the increased pressure within the thorax during the inspiratory phase of IPPV are, therefore, critical and are reflected in the ‘mean intrathoracic pressure’. This mean pressure, like the mean arterial blood pressure, is not the simple arithmetical mean between the highest and lowest pressures reached in the system and its calculation is not always easy for the non-mathematician. It is clearly important to keep this mean pressure as low as possible during the respiratory cycle and this can be accomplished in a variety of ways.
The shorter the inspiratory period during IPPV the lower the mean intrathoracic pressure will be for any given applied pressure. Theoretically, it might seem that the peak pressure should never be maintained – expiration should commence as soon as the peak pressure is achieved – or the circulation will suffer. However, the short application of a positive pressure may not result in very good distribution of fresh gas within the lungs. In humans, very short inspiratory periods have the effect of increasing the physiological dead space, while Hall et al. (1968) found a decrease in the physiological dead space/tidal volume ratio in horses ventilated with a ventilator which had a relatively long inspiratory phase. A compromise seems to be necessary here, but exactly what it is likely to be for any one animal of any one species remains pure speculation. It is usually taught that in small animals (dogs, cats, foals, calves, sheep), the inspiratory time should be 1.0–1.5 seconds and in adult horses and cattle 2–3 seconds provided the lungs are healthy.
If the necessary tidal volume of gas is to be delivered to the lungs in a short inspiratory period, it is clear that the flow rate will need to be high. The rate at which gas can flow into the lungs, however, is largely dictated by the resistance offered by the apparatus used and the airway resistance. The airway resistance to the various lung regions may not be uniform. For example, a bleb of mucus may partially obstruct a small bronchus and greatly increase the resistance to gas flow through it. A high gas flow rate through a neighbouring, unobstructed bronchus may result in overdistension of the alveoli supplied by it in an interval of time so short that the alveoli supplied by the partially obstructed bronchus will not have time for more than minimal expansion. Theoretically, it would seem that under these circumstances alveolar rupture might occur but, in practice, this complication seems rare unless pulmonary contusions exist from auto-trauma.
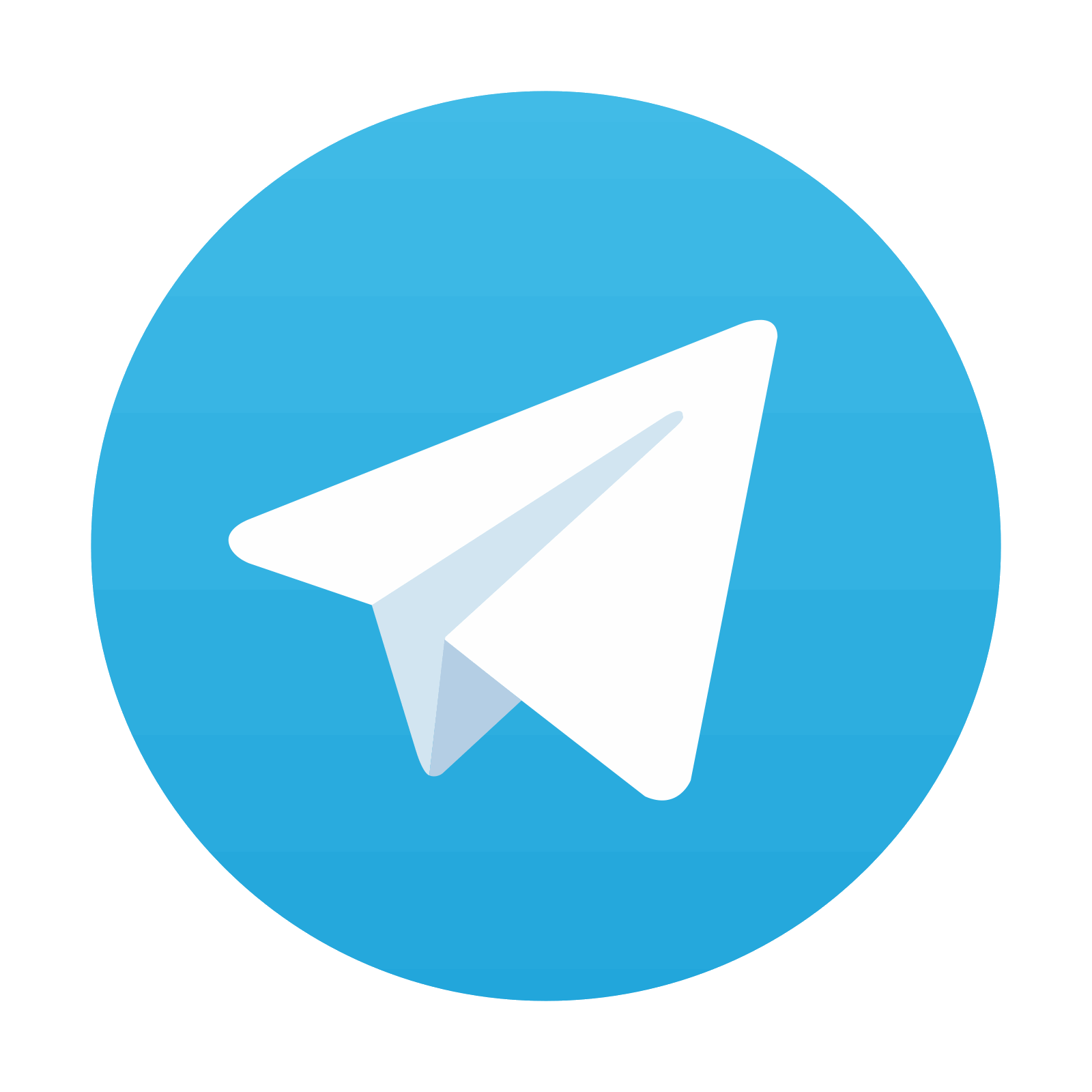
Stay updated, free articles. Join our Telegram channel
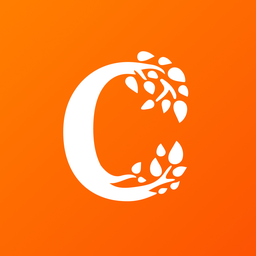
Full access? Get Clinical Tree
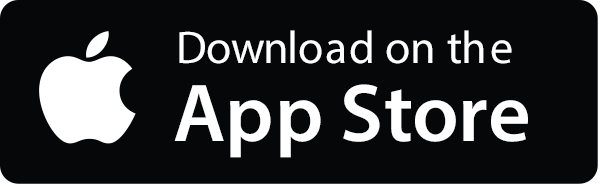
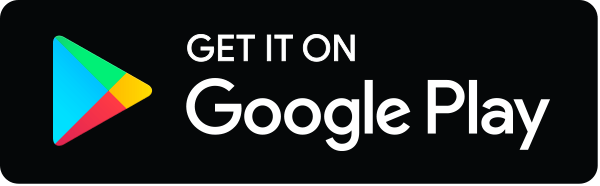
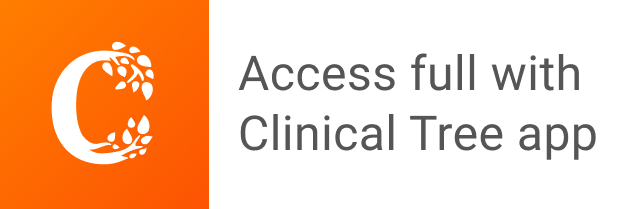