Chapter 5 Proteins, Proteomics, and the Dysproteinemias II. CLASSIFICATION OF PROTEINS B. Functions of the Plasma Proteins C. Factors Influencing the Plasma Proteins D. Handling and Identification of Proteins B. Fractionation of the Serum Proteins C. Electrophoretic Fractionation of the Serum Proteins VI. NORMAL PLASMA AND SERUM PROTEINS G. Multiplex Assays, Protein Arrays, and Acute Phase Index VII. INTERPRETATION OF SERUM PROTEIN PROFILES Protein is the most abundant component of plasma. There is in the region of 6 to 7 g/dl (60 to 70 g/liter) of protein in plasma in comparison to 0.1 g/dl (1.0 g/liter) of glucose (5.5 mmol/liter) or 0.35 g/dl (3.50 g/liter) of sodium (150 mmol/liter). However, this large mass of protein consists of many different individual protein molecules. Complete analysis of this complex mixture of different proteins is not possible at present in the diagnostic laboratory. Proteins contain approximately 95% of all nitrogenous material in blood in the form of chains of amino acids linked by peptide bonds. Protein can be separated from the nonprotein nitrogen (NPN) component of plasma by precipitation with reagents such as trichloracetic acid. The NPN consists of nucleic acids along with low molecular weight compounds such as urea (50% of NPN), free amino acids (25% of NPN), glutathione, and creatinine. Analysis of serum protein is an area of clinical biochemistry of domestic animals, which has seen a rapid advance since the 1990s, and with current developments in analytical technology and interpretation, the rate of advance is likely to accelerate rather than decline. At the forefront of these advances in the diagnostic application of serum protein analysis has been the development of specific assays for individual proteins. In particular, it has been recognized that quantification of a group of serum protein called the acute phase proteins (APP) can greatly assist the assessment of infection, inflammation, and trauma in animals. These advances are now being applied in clinical biochemistry laboratories for the immediate benefit in the diagnosis, prognosis, and monitoring of treatment of domestic animals. In the future, technology may be developed to characterize all proteins (the proteome) of serum, which would make a major contribution to the diagnosis of disease. However, it is a salutary lesson that currently only a limited number of the many plasma proteins are used for diagnostic analysis (Anderson and Anderson, 2002). This chapter focuses on the biochemistry, the diagnostic methodology, and use in disease diagnosis of measuring the concentration of serum or plasma proteins, but it will exclude a number of groups of proteins where the interpretation of results is more appropriate for other chapters. Immunoglobulins and complement will be covered in Chapter 6, lipoproteins in Chapter 4, and fibrinogen in Chapter 10. Studies on the proteins of blood have been performed on serum or plasma. Where appropriate, a distinction is made between these fluids, although apart from the absence of fibrinogen in serum, most diagnostic investigations can be applied to either. Nevertheless serum is the preferred sample for most of the diagnostic assays used to investigate the proteins of blood. The structure of proteins is defined by increasing levels of complexity. The primary structure of a protein is the sequence of amino acids that makes up the unique composition of the individual protein. The amino acids are joined together by peptide bonds linking the carboxylic acid group of one amino acid to the amino group of the neighboring amino acid in the chain. With 20 different amino acids occurring in proteins and with the possibility of more than a hundred or more amino acids making up the primary structure of each protein, there are an almost infinite number of potential proteins that could be present in cells and tissues. However, the sequence of the amino acids in a particular protein is predetermined by the order of nucleotide bases in nuclear DNA, which contains the genetic code for that protein. Secondary structure is the presence in protein of regular structures formed by the linked amino acids giving identifiably similar three-dimensional conformations. These may be repeated at intervals in the three-dimensional molecular structure of the protein. The most important of these structures are the α-helix and the β-sheet. The α-helix is a right-handed helix stabilized by hydrogen bonds between the C = O group of one amino acid residue and the N-H group of another amino acid located four residues along the peptide chain. The β-sheet is also stabilized by hydrogen bonds between carboxyl and amino groups of amino acid residues, but the interacting residues are at different parts of the same chain. An example of the α-helix is shown by the structure of albumin (Section IV.A), whereas the contribution of α-sheets to protein structure is illustrated by the structure of C-reactive protein (CRP) (Section IV.B.1). The α-helices and α-sheets can associate together into supersecondary structures forming recognized motifs among which the α-meander, Greek key, and α-barrel structures have been described (Walsh, 2002) The tertiary structure of proteins is the three-dimensional structure of the protein and is dependent on its primary and secondary structures. This native conformation of the protein is essential for its activity and depends on the correct folding of the protein after synthesis. Most proteins above a certain size can be subdivided into domains, which are independent folding units. The conformation of the protein is held together by weak forces between amino acid side chains such as hydrogen bonds, electrostatic and hydrophobic interaction, and also by covalent disulphide bonds between cysteine residues. Great strides have been made in determining the structure of proteins using X-ray crystallography and nuclear magnetic resonance (NMR). Structures of many proteins, including serum protein, can be obtained from online, open-access databases such as the Research Collaboratory for Structural Bioinformatics Protein Data Base located on the Internet at www.rcsb.org/pdb. The structures can be manipulated by protein modeling software, among which Protein Explorer or RasMol can be downloaded from www.umass.edu/microbio/rasmol. The quaternary structure of proteins is the combination of protein subunits to create a multisubunit complex. Thus, hemoglobin requires the combination of four subunits (two α-chains and two α-chains) into a tetramer for the fully functional protein. Examples of serum proteins that have quaternary structure include immunoglobulins, formed from two light chains and two heavy chains, and CRP, in which five subunits combine to form a pentameric structure. A further classification of protein based on their structure is between “fibrous” and “globular” proteins. The former adopt elongated three-dimensional shapes in their quaternary structure and are usually involved in structural roles in biological systems such as α-keratin, collagen, and elastin. Apart from fibrinogen (Section VI.B.5), which has as its function the formation of fibrin, fibrous proteins are not found in plasma protein. Thus, the majority of plasma proteins are globular proteins, adopting complex three-dimensional shapes by folding of the polypeptide chain. Proteins are also classified as “simple” or “conjugated.” Simple proteins contain only a polypeptide chain of linked amino acids, whereas conjugated proteins contain nonamino acid components. These can be carbohydrate residues (glycoprotein and proteoglycan), metal ions such as Fe2+ or Ca2+ (metalloproteins), phosphate (phosphoproteins), lipid (lipoproteins), and nucleic acids (nucleoproteins such as histones). Many plasma proteins are conjugated to carbohydrate and are present in the circulation as glycoproteins. Proteins can be classified by physical properties and behavior, by their size (relative molecular mass, Mr), or by the charge on the protein. The charge on a protein results from a combination of the acidic and basic groups on free side chains of the amino acids of the protein and is dependent on the pH of the aqueous environment. For every protein, there is a specific pH where the protein has an equal number of negative and positive charges on its side chains and the protein has a net charge of zero. This is the isoelectric point of the protein (pI). The higher the proportion of basic amino acids, such as lysine or arginine, the higher the pI of the protein will be, whereas with more acidic amino acids, such as glutamate or aspartate, the protein will have a low pI. The proportion of aromatic amino acids tyrosine, tryptophan, or phenylalanine contained by a protein influences its spectral properties because these amino acids absorb light at 280 nm, which can be measured in a spectrophotometer. The spectral property can also be influenced by factors such as the presence of heme groups and the binding of metal ions. The proportion of hydrophobic amino acids defines the hydrophobicity of a protein, which can be predicted from the primary sequence. Chemical composition in terms of the primary structure and the physical properties of proteins that have been sequenced are available from online databases such as the UniProt database at www.ebi.uniprot.org. The metabolism of nitrogenous compounds in animals is largely related to the processes of anabolism and catabolism of amino acids and proteins. Proteins in the diet are broken down by protease digestion to yield free amino acids and small peptides, the latter being finally degraded in the intestinal cells during absorption. The products of protein digestion enter the portal vein as amino acids. In the healthy animal, an equilibrium is established between intake and synthesis of amino acids, on the one hand, and breakdown and excretion of excess nitrogenous material, in the form of urea, on the other. Excessive loss of nitro-genous material can occur in illness because of cellular breakdown, lactation with production of milk protein, and in urinary or gut losses. During growth, pregnancy, and recovery from disease, there is a positive nitrogen balance as amino acids and other nitrogenous compounds are supplied to meet the body’s requirements. Proteins are made from amino acids in the cytoplasm of cells when the appropriate mix of amino acids is present. Among the 20 naturally occurring amino acids found in protein, nearly half cannot be synthesized by mammalian cells. These are the essential amino acids that have to be obtained in the diet (Table 5.1). The nonessential amino acids can be synthesized by transamination reactions in which the amino group of glutamate is transferred to a carbon skeleton in the form of an α-ketoacid. An example of this is the action of alanine transaminase, which cata-lyzes the transfer of the amino group of glutamate to the α-ketoacid, pyruvate, with the formation of alanine and α-ketoglutarate. Alanine transaminase (ALT; EC 2.6.1.2) is an important diagnostic enzyme, used as a marker of liver damage (Chapter 12) in small animals. The nonessential amino acids can be synthesized in animals from components of the central metabolic pathways, whereas the essential amino acids have to be present in the diet. However, in ruminants, the symbiotic relationship with ruminant microbes allows production of the full range of amino acids so that these species do not require all the essential amino acids in their dietary intake. The intricate process of synthesis of protein in the ribosomes of the rough endoplasmic reticulum, under the instruction of messenger ribonucleic acid (mRNA), is a major part of the discipline of molecular biology and will not be described here in detail as authoritative texts are devoted to the subject (Alberts et al., 2002). The primary structure of the protein is determined by the gene sequence of nuclear DNA on a chromosome in the nucleus. The genetic code, which is the sequence of nucleotides in DNA (adenine, cytosine, guanine, thymine), controls the sequence of amino acids in the protein. During protein synthesis, the code is transcribed from DNA to mRNA, which moves from the nucleus to the ribosomes in the cytoplasm. Here, specific amino acids are added to the growing peptide chain following linkage to a specific transfer RNA (tRNA). The specificity of production of the amino acid chain during this process of translation is dependent on the triplet of nucleic acids in the mRNA (codon) binding accurately to the anticodon of the tRNA. By this means, the genetic code in nuclear DNA directs the primary sequence of amino acids during protein synthesis. Formation of the peptide bonds between the amino acids of the protein is followed by folding of the protein into its natural conformation. With up to 20 different amino acids in protein chains of 100 residues or more, several million structural arrangements are feasible for any one protein. It is essential for protein function that they form the correct native conformation, and protein folding is an essential process following synthesis. Folding is the responsibility of chaperones, which guide the growing protein chain to produce the single structure that will ensure its full activity (Walsh, 2002). Throughout an animal’s body, proteins are continually being synthesized and broken down, resulting in a continuous turnover of protein. In a healthy animal, the rates of synthesis and degradation are in equilibrium, but during disease these can alter. Plasma proteins are subject to the same process, and a function of albumin, the most abundant plasma protein, is to provide amino acid for the natural turnover of protein in peripheral tissues. Albumin is taken up by pinocytosis into tissues where lysosomal proteases hydrolyze the protein, releasing the amino acids for utilization by the cells for synthesis of their own proteins (Evans, 2002). There is no storage capability in the body for protein. As a result, amino acid in excess of requirement for cellular protein synthesis is utilized for the central pathways of metabolism. The carbon skeleton of amino acids can be used for provision of energy via the tricarboxylic acid cycle and oxidative phosphorylation or may be converted to glucose or lipid and stored for later use. Carnivores derive as much as 40% to 50% of their energy from dietary protein, whereas omnivores and herbivores derive from 10% to about 20% from this source. The rate of degradation of the plasma proteins is expressed as their turnover, fractional clearance, or as their half-life, which is the time taken for their concentration to fall by 50%. Plasma half-lives were originally determined by measurement of the rate of disappearance of radioisotope labeled protein. More recently, proteins labeled with stable isotopes and measured by mass spectrometry have been used for this purpose (Preston et al., 1998; Prinsen and de Sain-van der Velden, 2004). Clearance half-lives for cellular proteins range from a few hours (enzymes) to as long as 160 days for hemoglobin in bovine red cells. The clearance half-life for plasma protein can be as long as 3 weeks. Plasma albumin in humans has a normal half-life of 19 days, α1-acid glycoprotein has a half-life of 5.5 days (Putnam, 1975), and γ-globulins have a half-life of 7 days (Andersen et al., 1963). The plasma half-life of albumin shows considerable variation between species (Table 5.2). It is associated with the size of the species with murine albumin having a T1/2 of 1.9 days, whereas equine albumin has a T1/2 of 19.4 days. During digestion, protein is not only broken down to amino acid, but gut bacteria can degrade the amino acids releasing ammonia, which is absorbed along with the amino acids. This is an important consideration in the management of liver disease, as sterilization of the gut by antibiotics can reduce the ammonia load on the liver. Once absorbed, amino acids, along with ammonia, are transported in the portal vein to the liver and then to other tissues where the amino acids are utilized for protein synthesis. The liver is the central processing organ for nitrogen metabolism, and approximately 75% of the amino acid (and ammonia) absorbed from the intestine is transported into the hepatocytes. Transaminase reactions facilitate the transfer of amino groups to appropriate α-ketoacids in the formation of the required balance of nonessential amino acids. If not required for protein synthesis, amino acids undergo deamidation by mitochondrial enzymes including glutamate dehydrogenase and glutaminase. Amino groups are also transferred to oxaloacetate, with the formation of aspartate, which along with an ammonium ion and a carbonate group are the precursors of urea. In this way, amino groups from excess amino acids are transferred into urea for safe excretion. In the urea cycle (Fig. 5-1), which takes place in hepatocytes, the initial step is the formation of carbamoyl phosphate in the mitochondria from an ammonium ion, a carbonate ion, and ATP. This step, which is under metabolic control and is activated by an increase in the cellular arginine concentration, occurs when there is an excess of amino acids in the hepatocyte. The carbamoyl phosphate combines with ornithine to form citrulline. This metabolite leaves the mitochondria and combines with aspartate to form arginosuccinate, which separates into arginine and fumarate. Urea is then released from arginine leaving ornithine, which reenters the mitochondria and the cycle repeats. There is a close link between the urea cycle and the tricarboxylic acid (TCA) cycle as the fumarate released from arginosuccinate is converted to malate and then oxaloacetate in reactions of the TCA cycle. Aspartate transaminase catalyses the transfer of an amino group for production of aspartate from the oxaloacetate, thus providing the amino group for further urea production. Division of the urea cycle between mitochondria and cytoplasm aids in coordination between cycles. The urea produced in the liver is transported in the circulation to the kidney where it is excreted by the kidney tubules and eliminated in urine. Other routes for the excretion of nitrogenous material, such as uric acid or nucleic acid, are relatively minor in mammalian species. FIGURE 5-1 The urea cycle. Formation of urea from precursors of aspartate, All animals are quite intolerant of free ammonia (NH3), but at physiological pH the protonated ammonium ion form predominates: The ammonium ion does not readily transfer across membranes unlike free ammonia, which readily enters cells where it is reconverted to the ammonium ion. Ammonia is particularly toxic to cells of the central nervous system where it acts by reducing the activity of the TCA cycle by removing a critical intermediate, α-ketoglutarate. Increased ammonia leads to the production of glutamate from α-ketoglutarate by the action of glutamate dehydrogenase. The α-ketoglutarate is lost to the TCA cycle, causing ATP production in the neurons to be restricted. Ammonia may also be directly toxic as it can decrease neurotransmitter concentrations. Ammonia is associated with hepatic encephalopathies of humans, horses (Hasel et al., 1999), and dogs (Reisdesmerie et al., 1995), possibly by affecting the expression of neuron proteins such as glial fibrillary acidic protein, glutamate transporters, and peripheral-type benzodiazepine receptors (Butterworth, 2002). Apart from the immunoglobulins, produced by B-lymphocytes, the major plasma proteins are synthesized and secreted from hepatocytes. Control of secretion is exerted by varied mechanisms. The secretion of albumin is stimulated by a fall in osmotic pressure (Evans, 2002) but can also be affected by pathophysiological changes such as during infectious or inflammatory disease when the secretion is reduced. This is caused by proinflammatory cytokines such as interleukin (IL)-1, IL-6, and tumor necrosis factor-α (TNF). These cytokines are simultaneously responsible for the increased synthesis and secretion of the APP (see Section VI.B). The immunoglobulins are produced by B-lymphocytes in the spleen, lymph nodes and bone marrow following stimulation by the presence of pathogen in the circulation or tissues. Use of molecular biological techniques such as Northern blots and the polymerase chain reaction (PCR) has revealed that nonhepatic tissues have the capability to synthesize some of the plasma proteins and that in certain circumstances the expression of their mRNA is up-regulated. Thus, in tissues such as intestine, lung, and adipose tissue, the mRNA for the plasma proteins haptoglobin and serum amyloid A are increased during infections and inflammation (Friedrichs et al., 1995; Vreugdenhil et al., 1999; Yang et al., 1995). The proportion of the proteins in the circulation derived from these nonhepatic sources has not been determined. A further site of nonhepatic synthesis is the mammary gland, which has been shown to produce a mammary associated serum amyloid A and haptoglobin during mastitis. However, the protein produced in the mammary gland does not appear in plasma but is secreted in the milk during the disease (Eckersall et al., 2001; Jacobsen et al., 2005b). The origin of low-abundance plasma proteins is varied. They may be made and secreted for specific functions such as the protein and peptide hormones. For example, the gonadotropins and adrenocorticotropin are released into the circulation from the pituitary gland, insulin and glucagon from the pancreas, and parathyroid hormone and calcitonin from the parathyroid gland. Similarly, the adipose tissue releases adiponectin and leptin, peptides affecting the appetite and nutritional status, whereas the cytokines are derived from cells of the immune system. The plasma also contains tissue proteins lost during normal turnover of cells, which can be valuable biomarkers of disease in identifying when pathological events occur. For example, enzymes determined in clinical enzymology for liver or muscle damage and the troponins released from cardiac or skeletal muscle are minor plasma proteins. The functions of proteins in the body of animals are innumerable and include forming the basis of structure of cells, organs, and tissues; maintaining colloid osmotic pressure; catalyzing biochemical reactions; and buffering acid-base balance. The proteins are also multifunctional in plasma. The functions of specific plasma proteins will be described later in this chapter, but main functions of protein in blood are in blood coagulation (fibrinogen), in host defenses against pathogens (immunoglobulins, complement), in transport of metabolites (transferrin, albumin), in regulation of cellular metabolism (hormones), in prevention of proteolysis (α1-antitrypsin), in provision of nitrogen balance for nutrition (albumin), and in maintaining osmotic pressure (albumin). The biological activities of the plasma proteins in these functions depend ultimately on their primary, secondary, tertiary, and quaternary structures. At birth, plasma proteins of most species are low because of minimal quantities of immunoglobulins. As the newborn animal ingests colostrum, a rapid rise in immunoglobulins occurs as a result of absorbed maternal immunoglobulins. As the maternal immunoglobulins reduce in concentration because of natural turnover, the neonate rapidly gains immunocompetence and begins to synthesize its own immunoglobulins. Upon reaching young adulthood, adult levels of globulins are reached. In contrast to this general trend, α1-acid glycoprotein is elevated in serum at birth in piglets but declines over the first few months of life (Itoh et al., 1993b). With increasing age, the plasma protein concentration increases as a result of a small decrease in albumin and a progressive increase in globulins. In some species, the total plasma protein concentration in the maternal blood decreases during gestation because of a decline in albumin, even though there is a slight increase in globulins. In a number of species pregnancy associated proteins have been observed during pregnancy. The only one in regular diagnostic use is equine chorionic gonadotropin (formerly pregnant mare serum gonadotropin) used to confirm pregnancy in mares (Henderson et al., 1998). In pregnant bitches, an acute phase reaction occurs about 21 days after fertilization and acute phase proteins, especially C-reactive protein, increase in the maternal serum (Eckersall et al., 1993; Vannucchi et al., 2002). In cattle approaching term, there is a rise in γ-globulins and a corresponding rise in total plasma protein, but at term a fall occurs in this fraction because of transfer to colostrum (Weaver et al., 2000). The acute phase protein, serum amyloid A (SAA), also increases in the maternal plasma around parturition in cows (Alsemgeest et al., 1993). During lactation, the total plasma protein decreases in some species because of albumin decrease. Some hormones (testosterone, estrogens, growth hormone) promote an increase in total plasma protein because of their anabolic effects, whereas others (thyroxine, cortisol) tend to decrease the total plasma protein because of their catabolic effects. Protein denaturation is the net effect of alterations in the biological, chemical, and physical properties of the protein by mild disruption of its structure. When blood samples are taken for protein analysis, it is important that they are handled correctly so that no artifacts are introduced that could affect the investigation and its interpretation. If the protein is allowed to even partially degrade, the assay will not be accurate. Therefore, it is essential that denaturation is avoided. The ability of plasma proteins to resist denaturation in a blood sample taken for diagnostic analysis varies between proteins; consequently, the sample should be handled according to the analysis required. Fortunately, most major plasma proteins are relatively resistant to denaturation and can be assayed in samples that have been handled carefully and have been kept away from elevated temperatures. However, separation of plasma or serum from the blood cells by centrifugation should be performed as early as possible. Thereafter, many proteins are stable at 4°C for several days and at –20°C for much longer (months to years). Some proteins are less stable, with enzymes being particularly susceptible to loss of activity with time, while the stability of the peptide hormone ACTH is so low that samples should be snap-frozen immediately to preserve the intact peptide. For identification and quantification of serum protein, the protein component in serum must either be separated or individual proteins must be measured independently. The primary separation of the proteins in serum is between albumin and the globulins. Albumin is a water-soluble, globular protein that is usually identifiable as a single discrete molecule. The globulins are also globular proteins, but many of them, in contrast to albumin, precipitate in pure water and require salts to maintain their solubility. The globulins are a mix of proteins of various types, which migrate in groups in an electric field (electrophoresis) as families of proteins identified as α-, β-, or γ-globulins. The nomenclature of the globulin fractions is based on their location during separation by electrophoresis. Albumin has the most rapid migration of the major proteins (in some species it is preceded by prealbumin), followed by the α-globulin, β-globulin, and γ-globulin fractions, respectively. The γ-globulins are largely composed of immunoglobulins, the antibodies that bind to invading pathogens or other foreign matter. In contrast, the α– and β-globulin fractions contain a great variety of different proteins. Electrophoresis is a well-established diagnostic method that was first introduced to the clinical biochemistry laboratory with cellulose acetate as the support medium for the separation. This has largely been replaced with agarose, so that serum protein electrophoresis (SPE) in agarose gels, followed by protein staining and densitometry to quantify the protein in each of the main fractions, is common in clinical biochemistry laboratories. This has evolved into an extremely useful technique because aberrations are observed in many disease states though there are only a few diseases where the electrophoretic pattern can provide a definitive diagnosis. Interest has advanced the investigation armory for serum protein analysis with the development of specific analytical methods for individual proteins. Though specific assays have been used for a long time for determination of proteins such as albumin and fibrinogen, it is only relatively recently that specific assays for other diagnostically useful proteins such as haptoglobin, CRP, SAA, and α1-acid glycoprotein (AGP) have become commonly available. In most cases, this has been achieved by the use of immunoassays, which has often required the development and validation of species-specific methodology. Assays for total protein can be performed on serum or plasma. The method employed to measure the total amount of protein in solution varies with the amount of dissolved protein and is therefore chosen according to the biological fluid under investigation. The technology used to measure total protein can be based on chemical or physical measurements. In the diagnostic laboratory, chemical methodologies are used because they can be readily adapted to automated analyses. On the other hand, point-of-care determination of total protein, for instance, in a veterinary practice, can be performed by use of refractometry, which depends on the physical properties of protein in solution. a. Biuret Reaction The biuret reaction, in which protein forms a complex with copper (Cu2+) in alkaline solution, has become the standard chemical test for total serum or plasma protein. This complex, which is dependent on the presence of peptide bonds, is blue-purple in color. This method is used in automated wet biochemical analyzers and is also the basis for total protein assays in dry chemistry analyzers. The biuret method is highly accurate for the range of total protein found in serum (1 to 10 g/dl, 10 to 100 g/liter) but is not sensitive enough for the protein concentrations found in other body fluids where the concentration range is lower, for example, cerebrospinal fluid. More sensitive protein assays should be used for these fluids. b. Precipitation Methods Proteins in solution are sensitive to changes in the pH of the environment that result in alteration of the ionization of the side groups of acidic and basic amino acids. This distorts the electrostatic forces between residues, which normally keep the protein in its native conformation. Changing the pH, especially to the extremes of the pH range, therefore disrupts the tertiary and quaternary structures of proteins leading to reduced solubility and causing precipitation of the protein from solution. Reagents such as trichloroacetic acid, sulphosalicylic acid, and tungstic acid cause the precipitation of protein and are used to quantify the total protein concentration in biological fluids when the protein concentration is in a range of 0.1 to 1 g/dl (1 to10 g/liter). c. Sensitive Chemical Methods For measurement of total protein in fluids at concentrations less than 0.1 g/dl (1 g/liter), more sensitive protein assays have to be used. For many years, the Phenol-Folin-Ciocalteau method (Lowry et al., 1951) was the method of choice to measure low concentrations of protein. This method is based on the reaction of the phenolic group of tryptophan and tyrosine with the Folin-Ciocalteau reagent yielding a blue chromogen. A less laborious modification of this method, which is even more sensitive, has been developed using bicinchonic acid (Smith et al., 1985), whereas methods based on the binding of the dye, Coomassie blue to protein in acidic solution, are also useful in quantifying dilute protein solutions (Bradford, 1976). These sensitive methods are conveniently performed in microtiter plates, but their use is mainly restricted to the research laboratory. These sensitive methods depend on the reactions between reagent and a number of specific amino acids in the proteins, such as with the phenolic group of aromatic amino acids. Results vary depending on the proportion of these amino acids in the proteins being measured. The protein used to calibrate the assay may have a significant effect on results. Conventionally, bovine serum albumin is used as calibrant. a. Refractometric Light is refracted when it passes from air to liquid, and if the liquid contains dissolved proteins, the degree of refraction (refractive index) changes in proportion to the concentration of protein. With appropriate instruments and careful use, determination of the refractive index of serum can give an accurate assessment of total protein concentration. The use of hand-held refractometers allows rapid determination of protein in serum, plasma, or other body fluid and is one of the most widespread point-of-care methods in use. It is important to frequently check the calibration of the refractometer, as this may be a source of error. Most refractometers are scaled to read directly both total serum protein and urine-specific gravity. Studies have shown that results for protein estimates from hand-held refractometers correlate well with results from the biuret method, though there are reports of major discrepancies in samples from avian species (George, 2001). Because of the dependence on the transmission of light, it is important that the sample is clear, nonturbid, nonlipemic, and nonhemolyzed. In a comparison of refractometry to the biuret method for the determination of the total protein in plasma from dogs and cats, the correlation coefficients were high, but there were differences between the methods of 0.6 g/dl (6 g/liter) and 0.2 g/dl (2 g/liter) for dog and cat plasma, respectively (Briend-Marchal et al., 2005). Although the internal scales on most refractometers limit the measurement of protein to a minimum of 2.5 g/dl (25 g/liter), it has been shown that a close correlation to total protein determined by the biuret method can be obtained to concentrations as low as 0.6 g/dl (6 g/liter), allowing the use of the method to estimate the protein content in most body fluid samples (George and O’Neill, 2001). b. Fibrinogen Refractometry can be used to determine the concentration of fibrinogen in plasma. This large protein (340 kDa) constitutes about 5% of the total plasma protein, and its concentration can be estimated from the difference in protein content before and after heat treatment of plasma at 56°C for 3 minutes, which causes fibrinogen to precipitate. A refractometer is used to determine the protein concentrations before and after heating with the fibrinogen being estimated from the difference between the two readings. Fibrinogen has also been estimated by measurement of the height of fibrin clot in microhematocrit tubes or the assessment of the weight or the protein content of fibrin clots (Davey et al., 1972). Monitoring the fibrin formation spectrophotometrically via enzymic action of thrombin or the snake venom, batroxobin, on fibrinogen allows the assay to be automated (Messmore et al., 1997; Oosting and Hoffmann, 1997) and has been used to monitor canine fibrinogen (Mischke et al., 2005). Thrombin time, as described in Chapter 10 on hemostasis, is also used to estimate fibrinogen concentration. The total protein content of serum is made up of a large number of individual proteins, and diagnostic information can be obtained by detecting changes in the component proteins or in different fractions of proteins. It has been suggested (Anderson and Anderson, 2002) that virtually all diseases affect the proteins found in serum and that diagnosis would be advanced by using proteomics methods (Section V.C.4) to monitor change in many serum proteins at the same time. This is likely to be in the distant future for routine applications in veterinary clinical pathology laboratories. Nevertheless, considerable valuable diagnostic information can be obtained using current methods to fractionate serum to determine the concentration of its major proteins or groups of proteins. Most of these methods require the initial determination of the total serum protein concentration, which is then used in calculation of the protein content of different fractions. In its simplest form, the globulin fraction can be estimated if the total protein and albumin concentrations are known. The globulin concentration is the difference between the total protein and albumin concentrations. For quantitative estimation of the subdivided globulin fractions (α-, β-, or γ-globulins), the percentage of each fraction in a serum sample can be determined by electrophoresis and the concentration of each fraction calculated from the total protein by proportion. The weak bonds that hold together the secondary, tertiary, and quaternary structures of proteins can be disrupted by a variety of changes in the aqueous environment leading to reduced solubility. Because of the different amino acid composition of proteins, alterations in the environment have differential effects on individual proteins, and salt fractionation of serum protein exploits this property. The addition of salts to serum increases the ionic concentration causing the flocculation and precipitation of the globulins (particularly γ-globulins), whereas albumin is more resistant to increased ionic charge and remains in solution. Precipitation with ammonium sulphate is a widespread technique used in the purification of serum (and other) proteins. For diagnostic test use in animals, the most common application of salt precipitation is in assessment of the transfer of antibody (γ-globulins) from colostrum to the serum of the neonate (Weaver et al., 2000). The optimal concentrations of sodium sulphite (Pfeiffer and Mcguire, 1977) or zinc sulphate (McEwan et al., 1970) have been determined, which, when added to a serum sample, will only precipitate the γ-globulin fraction. Thus, serum from calves or foals in which passive transfer of immunoglobulin has occurred will show an increase in turbidity, whereas a sample from a neonatal animal that has not absorbed colostral antibody will remain clear. The simplicity of these methods has allowed their use as point-of-care methods. A recent reevaluation of the use of sodium sulphite found that a concentration of 18% (w/v) provided the optimal diagnostic value. With zinc sulphate as the precipitant, a similar diagnostic value was found, but it was affected by hemolysis and the test solution was not stable when exposed to atmospheric carbon dioxide (Tyler et al., 1999). An alternative approach to assessment of antibody transfer, which also causes the precipitation of the γ-globulin fraction, is the glutaraldehyde coagulation test (Sandholm and Kivisto, 1975). Glutaraldehyde reacts with free amino groups on proteins causing cross-links to form between protein chains and, if sufficient numbers are produced, will cause aggregation of the proteins and visible precipitate formation. γ-Globulins have the highest proportion of the basic amino acids (lysine, arginine), which have free amino groups available for reaction with glutaraldehyde. Therefore when mixed with glutaraldehyde at a predetermined concentration, a serum sample with a high γ-globulin concentration will form cross-links and precipitate formation will be visible, whereas a sample with low γ-globulin will not produce a precipitate. However, fibrinogen can also form cross-links with glutaraldehyde (Liberg et al., 1975) and can cause interference with the test when it is used for antibody detection in plasma, especially in cases of hyperfibrinogenemia. Indeed, though the glutaraldehyde test was deemed to have poor sensitivity and specificity for detection of failure of passive transfer in calves (Tyler et al., 1996), it was found to be a useful screening test able to distinguish between acute and chronic disease in horses (Brink et al., 2005) because of this reaction with fibrinogen in plasma. The seromucoid fraction of serum is a group of highly soluble glycoproteins that have the ability to remain in solution in the presence of perchloric acid while other proteins are precipitated. Methods have been developed to estimate this “acid soluble glycoprotein” fraction by addition of perchloric acid to aliquots of serum (Nagahata et al., 1989). As most of the glycoproteins in the seromucoid (acid soluble glycoprotein) fraction are APP, this was an early means for monitoring the acute phase reaction. Albumin has the highest concentration of any of the individual serum proteins, and valuable diagnostic information can be obtained by measurement of its concentration. Various dyes have been found that, after binding to albumin, change their absorbance and are used as a means to measure the protein in a spectrophotometer, in automatic analyzers, or in dry chemistry systems. The dyes used most widely for this procedure are bromocresol green (BCG) and bromocresol purple (BCP). BCG has become the favored dye to utilize in dye-binding assays for albumin (Keay and Doxey, 1983), although BCP has been recommended for equine serum albumin (Blackmore and Henley, 1983). Accuracy of the dye-binding methods is generally acceptable within the albumin concentration reference ranges found in animals. However, the accuracy decreases outside the reference ranges and may be unacceptable at very low or very high albumin concentrations. With heparinized canine plasma, it is possible that interference from fibrinogen can lead to overestimation of the albumin concentration when using BCG (Stokol et al., 2001). Once the albumin and total protein concentrations have been determined, the globulin fraction can be calculated by subtraction of the albumin from the total protein concentrations. The albumin:globulin ratio can then be calculated (albumin concentration/globulin concentration). This provides a means of assessing the relative contribution of the albumin or the globulins to the total serum protein, which complements the analysis of either analyte alone (Section VII.B). Electrophoresis is the method of choice for analytical separation of protein. Serum protein electrophoresis (SPE) is currently regarded as the standard of reference for fractionation of serum protein. Serum rather than plasma is used as the sample for electrophoretic separation because it reduces the complexity of interpretation by the removal of fibrinogen. Many modifications have been made to the basic principles of electrophoresis since the separation of protein in an electric field was first pioneered by Tiselius (Tiselius, 1937). Many of these methods have been applied to serum proteins, but only a few have been employed in clinical biochemistry. A major difference between methods is the nature of the support material for the protein separation. From the mid-20th century, the cellulose acetate membrane was utilized for this purpose for SPE (Kohn, 1957). Toward the end of the century, electrophoresis on agarose gel was introduced and has become more popular in diagnostic laboratories. In contrast, biochemical research laboratories almost universally use polyacrylamide gel as the separation medium. Major advances have been made in the ability to separate protein with the introduction of two-dimensional electrophoresis (2DE) and associated proteomic techniques. Whereas SPE on agarose can separate serum into seven or eight fractions, it is claimed that proteomic methodologies can separate and also identify several hundred proteins simultaneously. Although these new methods have not been validated for use in domestic animal clinical biochemistry, it is valuable to be aware of the possibilities that could be available by application of these methods. The principle for all protein electrophoresis is based on the movement of charged protein molecules in an electric field. In original studies in the early 20th century, electrophoresis was carried out in solution. This “free electrophoresis” was subsequently replaced by methods in which the proteins are separated in the matrix of a support medium in which the charged proteins and buffer ions are still able to move. The use of support medium has the benefit of reducing interfering problems and allows greater reproducibility. The choice of support can have direct consequences on the separation obtained during electrophoresis. As well as the nature of the support media, the migration of proteins depends on their size and charge, the pH and ionic composition of the buffer, and the strength of the electric field. The charge on the protein is dependent on the balance of acidic and basic amino acids in its primary structure and varies with pH. Thus, at a neutral pH, protein with a high proportion of acidic amino acids will have an overall negative charge, whereas a protein with a preponderance of basic amino acids will have an overall positive charge. SPE is usually performed at a basic pH (pH 8.6) so that most protein will have a negative charge. Molecules with a negative charge move toward the anode (positive electrode) when an electric current is passed through the solution. A side effect of agarose electrophoresis is that electroen-dosmosis occurs because of impurities in the agarose, causing the migration of the more basic (γ-globulins) proteins to the cathode (negative electrode). Interestingly, one of the most recent innovations in electrophoretic separation has been to revert to electrophoresis in the absence of support media, but in this case using a very narrow bore capillary column. This “capillary zone electrophoresis” has the benefit of providing more rapid and reproducible analyses for individual samples, but the equipment is more specialized than that for SPE. These methods have been applied to canine serum protein fractionation (Martinez-Subiela et al., 2002b) with results analogous to conventional SPE, though hemolysis and lipemia were found to cause interference in the β and α regions, respectively. Specific proteins such as haptoglobin can also be measured using capillary zone electrophoresis (Pirlot et al., 1999). For several decades, cellulose acetate was the method used in diagnostic laboratories for SPE, but the easier use, greater reproducibility, and commercial availability of agarose gels specifically produced for SPE have meant that use of the latter method is now more common. Agarose is a polysaccharide-based material derived from seaweed. When used for electrophoresis at a concentration of ∼1% (w/v), the agarose forms a gel through which serum protein can move relatively freely. The introduction of plastic backed previously prepared gels meant that the handling, staining, and quantification of results could be easily undertaken. Typically in an SPE run, serum samples are diluted 1:5 in buffer, and 5μl are placed close to the center of the gel, slightly on the cathodal side; after the sample has diffused into the agarose, a voltage is applied across the agarose and the proteins are allowed to separate. The mobility of the proteins is based on a mix of their charge at the pH of the buffer (usually pH 8.6) and the size of the protein. Albumin has a high negative charge under these conditions and is also relatively small, so it is one of the most mobile proteins in moving toward the anode. At the other extreme the immunoglobulins (especially γ-globulins) have the least negative charge and are affected by electroendosmosis and migrate toward the cathode. The α– and β-globulins have intermediate mobility between albumin and the γ-globulins. Agarose SPE allows the subdivision of these groups, and α1, α2, β1, β2, γ1, and γ2 fractions of proteins may be observed (Trumel et al., 1996), though this can vary between samples and between species. Following electrophoresis the proteins are fixed in the gel and visualized by staining with a stain such as amido black. Usually 10 samples will be run on one agarose gel with each sample in a different “track.” After staining and clearing the gel, the proportion of proteins in each fraction can be estimated by densitometry. In most instruments a computer-generated printout will provide a graphical representation of the absorbance readings from the densitometer and will also calculate the percentage of protein per fraction. This allows the calculation of the protein content of each fraction based on the total serum protein concentration. Examples of agarose SPE and densitometer scans of the major domestic animal species are illustrated in Figures 5.2 and 5-3. FIGURE 5-2 Agarose gel serum protein electrophoresis showing the separation of normal serum protein from healthy animals. Samples are from (a) sheep, (b) cow, (c) pig, (d) dog, (e) cat, and (f) horse. A recently introduced modification to the commercially available agarose gel allows high-resolution electrophoresis (HRE) to be employed for SPE. This method has been applied to canine serum and was able to localize specific serum proteins within the different subfractions. Thus, haptoglobin and α2-macroglobulin were identified in the α2 fraction, β-lipoprotein and complement C3 were located in the β1 region, and transferrin and IgM were located in the β2 region (Abate et al., 2000). The use of HRE may become more widespread as it is as user-friendly as conventional agarose electrophoresis. FIGURE 5-3 Electrophoretogram of agarose gel serum protein electrophoresis of serum from healthy animals. The most widely used support medium for protein electrophoresis outside the diagnostic laboratory is polyacrylamide gel (PAGE). The use of this medium brings a further factor to the electrophoretic separation of protein. During the polymerization of acrylamide to form the gel used in electrophoresis, the proportion of cross-links between polymer chains can be controlled, and the gel forms a molecular sieve that slows the migration of proteins depending on size. In its original version of discontinuous polyacrylamide gel electrophoresis (Davis, 1964), a strategic use of different buffer systems in the gel, in the sample, and in running buffer caused the proteins in the sample to focus into a sharp band before entering the gel. Once in the gel, separation was based on a balance of mass and charge on the protein. The most widely used modification of this system is to pretreat the proteins by heating in a solution of detergent (sodium dodecyl sulphate, SDS) and a reducing agent such as β-mercaptoethanol. These have the effect of separating any subunits held together by disulphide bonds and coating all the proteins with negative charge so that separation, with the same detergent also in the gel and buffers, is based on size alone as all protein will move to the anode because of their negative charge. This is the SDS-PAGE system introduced by Laemmli (Laemmli, 1970). Separation of serum protein on SDS-PAGE increases the complexity for interpretation of the electrophoretogram. The proteins are no longer grouped in the familiar globulin regions but are in a series of bands defined by relative molecular mass (Mr). The treatment and breakdown of complex proteins into their component subunits complicate interpretation. The high abundance of just a few of the proteins, such as albumin and the immunoglobulins, causes further difficulty in interpretation. Added to this are the more technically demanding methods required for SDS-PAGE such that this method is largely confined to the research laboratory. Nevertheless, separation of serum protein by SDS-PAGE has revealed disease-related changes in protein bands (Fagliari et al., 1998; Kiral et al., 2004), but there has not been a widespread application of the method in diagnostic biochemistry. A further separation technique for electrophoretic fractionation of protein mixtures, introduced in the 1970s (Righetti and Drysdale, 1971), is isoelectric focusing (IEF). This technique, which can be performed in agarose or polyacrylamide gels, differs from other forms of electrophoresis by separating the proteins solely on the basis of their charge. The presence of special reagents, called ampholytes, in the buffer creates a pH gradient once an electric voltage is set up across the gel. Proteins in the gel move because of their relative charge, but once they reach their isoelectric point (pI) on the pH gradient, they become stationary, as they now have zero charge. Thus, an acidic protein with a negative charge will move toward the anode, but as it moves down the pH gradient the protein becomes less charged until it reaches the point where it has no net charge and it is “focused” at their pI. This method has a high resolution and can separate protein isoforms that have only slight charge differences caused, for instance, by glycosylation or phosphorylation of proteins. This greatly increases the potential number of bands that can be seen on IEF gels, but the method has not been adopted by diagnostic clinical biochemistry laboratories for separation of serum proteins, possibly because of this great complexity. However, IEF has been used in examination of enzyme isoforms (Eckersall and Nash, 1983) and can be used to identify microheterogeneity in specific serum proteins, for instance, being able to differentiate multiple forms of AGP that are caused by different degrees of glycosylation (Itoh et al., 1993a; Yoshida et al., 1997). Protein analysis is currently going through rapid evolution that could impact the veterinary diagnostic laboratory in the not-too-distant future and is being driven by advances in proteomics. Technological developments in different disciplines have converged to produce an approach to the separation, identification, and quantification of individual proteins within a complex mixture. The objective of a proteomic investigation is to be able to identify all proteins in a tissue or fluid and to detect even small changes taking place in its composition. Although this goal is still beyond the reach of all but the best-funded research laboratories, it is probable that proteomic techniques will eventually be used in diagnosis of disease. Analysis of serum or plasma protein will be at the forefront of these advances. It has been suggested that the human plasma proteome could be used to detect virtually all pathological processes because every diseased tissue is in contact with the circulation and interchanges material with plasma (Anderson and Anderson, 2002). As many as 1175 distinct gene products have been reported in human plasma by a combination of methods (Anderson et al., 2004), whereas 289 proteins have been directly detected. However, only 117 of these have been registered in the Untied States by the Food and Drug Administration under the Clinical Laboratory Improvement Amendment for use in diagnostic investigation of plasma (Anderson and Anderson, 2002). Investigation of the diagnostic potential of animal serum or plasma proteomes is at a much earlier stage, but it has the potential to yield many novel diagnostic applications. a. Two-Dimension Gel Electrophoresis The new science of proteomics (James, 1997) initially developed from methods in which the electrophoretic techniques of IEF and SDS-PAGE were combined into two-dimensional electrophoresis (2DE) (O’Farrell, 1975). Combination of these methods leads to a protein map, where a protein mixture is separated horizontally by charge (IEF) and vertically by molecular mass (SDS-PAGE) yielding a two-dimensional map with each protein present as a single spot. An innovation that meant that these protein maps were much more reproducible was the introduction of immobilized pH gradients for use in the IEF step (Gorg et al., 2000, 2004). In 2DE, the protein sample is subjected to IEF in a gel strip containing the immobilized pH gradient, and then the strip with the focused protein is placed on the top of an SDS-PAGE gel. After electrophoresis, the separated proteins are stained, using either Coomassie blue or the more sensitive silver or fluorescent stains. The amount of data generated by a 2DE gel can be vast, and a computer program is required to handle the analysis. FIGURE 5-4 Two-dimensional electrophoresis of serum protein from (a) horse, (b) cow postcolostrum, (c) cow precolostrum, (d) sheep, (e) cat, and (f) dog. Labeled proteins are 1: albumin, 2: transferrin, 3: IgG heavy chain, and 4: IgG light chain. Gels were run on an IPG gradient from pH 4-10 (nonlinear) and then on SDS-PAGE. Gels courtesy of Ingrid Miller, Institute of Medical Chemistry, Department of Natural Sciences, University of Veterinary Medicine, Vienna, Austria. The serum proteomes of a number of domestic animals are illustrated in Figure 5.4. It is noticeable that albumin is the most abundant protein in adult serum and that the IgG spots are missing in serum from a precolostral calf (Fig. 5.4c). The serum proteomes of cattle and horse have been more fully determined with 30 and 50 proteins identified, respectively (Miller et al., 2004; Wait et al., 2002). Identification of protein spots following 2DE was originally performed with antibody detection, specific stains (e.g., for lipoprotein), or by comparison to the proteins of other species. A further advance that greatly facilitated proteomic research was the use of mass spectrometry to identify protein spots on gels. b. Mass Spectrometry for Protein Identification Mass spectrometry has been used for many years in investigations to measure the mass of molecules to a high degree of accuracy, but for a long time it was restricted to low-molecular-weight compounds. In the 1980s and 1990s, methods were introduced to determine the mass of larger molecules such as peptides and smaller protein. This was achieved with the introduction of electro spray ionization (ESI) (Fenn et al., 1989) and matrix-assisted laser desorption/ionization (MALDI) (Karas and Hillenkamp, 1988). These methods are central to alternative approaches to identify the protein on 2DE gels and have accelerated the development of proteomics. Mass spectrometry actually measures the mass-to-charge ratio (m/z) of ions under vacuum. To perform this process, a means of generating the necessary ions, a mass analyzer and a detector are required (Patterson and Aebersold, 1995). In proteomic investigation, the most widespread approach is the MALDI time-of-flight (TOF) mass spectrometer, which is used in combination with database searches to identify the protein in a specific spot on 2DE protein maps. Protein identification by MALDI-TOF mass spectrometry is based on some prior knowledge of the amino acid sequences of likely proteins to be identified or of equivalent proteins in other species. It is dependent on enzymic cleavage of the protein into shorter peptides, the size of which is defined by their component amino acids. Before mass spectrometry, the protein spot to be identified is cut out of the polyacrylamide gel and subjected to hydrolysis by a specific protease, usually trypsin. The site of action of trypsin is at the peptide bond of the basic amino acids lysine and arginine. The trypsin digest products of any proteins whose primary structure is held on international protein databases such as UniProt (www.ebi.uniprot.org) or NCBI (www.ncbi.nlm.nih.gov) can be predicted from the position along the protein sequence of the arginine or lysine residues. The tryptic digest product of any protein whose primary structure can be derived from genetic (DNA) databases can also be determined. These tryptic digest “fingerprints” are characteristic of each protein and identify protein spots after 2DE. The trypsin-digested sample is mixed with a matrix compound and dried on a metal slide, which is inserted into the mass spectrometer. Bombardment of the slide by a laser results in the ionization of the peptides and application of a high voltage causes the ions to travel rapidly to the detector with smaller ions having a greater velocity. Thus, small peptides have a shorter time of flight than larger peptides, and from this the mass of each peptide is determined to a high degree of accuracy. The data generated by the MALDI-TOF are thus the mass of each of the peptides produced by the trypsin digest of the protein excised from the 2DE gel. Identification of the protein is completed by comparison of the masses of all the peptides produced by trypsin digest to the protein and gene databases. This is especially useful in species where the whole genome has been sequenced such as human or mouse. There are means to identify proteins by this peptide fingerprint approach even where the genome has not been determined (Wait et al., 2002), though a number of genomes of the domestic species (cow, dog, chicken) are close to being fully sequenced, which will simplify the proteomic investigation of samples from these species. More advanced mass spectrometry using ESI in tandem MS with quadruple instrumentation can directly determine sequences of peptides, but these methods are more time consuming. An advantage of MALDI-TOF is that it can be used in robotic systems where computer-controlled workstations can excise multiple spots from a 2DE gel and automatically perform the trypsin digest, transfer the hydrolysate to the mass spectrometer, and identify the protein as a probability score of the most likely candidate protein. c. Non-Gel-Based Proteomic Analysis When first developed, proteomics combined protein separation on 2DE and mass spectrometry. However, for use in diagnostic investigations, 2DE is an expensive, time-consuming, and difficult technique to reproduce precisely. It is likely to remain a research tool unless major advances are made in the robustness of the methodology. Interest is shifting to non-gel-based approaches to proteomics in which alternatives to 2DE are used for protein and peptide separation but with mass spectrometry still being used for identification. These methods have a greater potential for automation, throughput, precision, and accuracy, which may in the future allow their use in disease diagnosis. One such approach is surface enhanced laser desorption ionization mass spectrometry (SELDI-MS). In this method, a sample such as serum is preincubated with a “protein chip,” which has one of a variety of surfaces to which proteins bind with differing affinity. These surfaces are designed to bind with protein by ion-exchange, hydrophobic, or metal chelate interaction. After washing away unbound protein, the protein chip is placed in the SELDI-MS instrument and subjected to mass spectrometry. The system is optimized to identify biomarkers for disease by contrasting samples from healthy and diseased animals. This approach can identify peptide or protein peaks in the mass spectrogram that have potential as biomarkers and has been used to identify biomarkers for ovarian cancer and other diseases in humans (Petricoin et al., 2002). A drawback of the current SELDI-TOF system is that identification of the protein biomarkers requires further investigation by traditional protein biochemistry methods. Another approach to non-gel-based proteomics is nano liquid chromatography coupled to mass spectrometry. Native proteins are in general too large for mass spectrometry, so before separation the sample is treated with trypsin, producing shorter peptides. The peptides are separated by high-pressure liquid chromatography (HPLC) with the output coupled to an ESI mass spectrometer (Gaskell, 1997; Mehlis and Kertscher, 1997). The results can be plotted with elution volume from the HPLC against the mass/charge (m/z ratio) of the peptide and the size of the peptides compared to protein and gene databases. An interesting finding from a number of proteomic investigations using gel and nongel approaches designed to identify cancer biomarkers has been that many of the identified candidate biomarkers have already been identified with many of them being APP (Diamandis and van der Merwe, 2005). It will be fascinating over the next few years to see if these advanced techniques can earn a role in the veterinary diagnostic laboratory. Serum protein is composed of many different individual proteins with current electrophoretic fractionation in routine diagnostic use (SPE) only providing a guide to the disease-related changes affecting serum proteins as only a small number of protein fractions can be consistently characterized. Undoubtedly the ideal diagnostic approach would be to monitor the changes in concentration of most if not all serum proteins, and this is the ultimate objective of proteomic investigation. However, until the technology advances sufficiently, the only means to measure changes in individual serum proteins is to use assays that directly measure the specific proteins. In recent years, there has been increasing success in identifying proteins with sufficient diagnostic value to develop suitable assays to perform routine analysis. Some serum proteins can be measured by methods where physical, chemical, or biological activities can be exploited (Sections V.A.2.b and V.B.2). However, most serum proteins are measured by immunoassay requiring a specific antibody raised against the target serum protein. Though there is cross reactivity between species for a number of serum proteins, it is advisable to use species-specific antiserum or to thoroughly validate assays developed with antisera to species other than the one under investigation. Immunoassays have become an established weapon in the arsenal of the clinical biochemistry laboratory, especially where the exquisite specificity of antibody can be harnessed for diagnostic procedures. Antibodies for use in immunoassays for serum proteins can be polyclonal or monoclonal. They are usually raised against the proteins purified from serum, though a recombinant protein may be produced if the gene sequence is known. There are several ways in which antibodies can be incorporated in immunoassays to provide qualitative or quantitative data with the choice of method being dependent on several factors. The range of analyte concentration, time taken to run an assay, and ease of automation are among the considerations taken into account when setting up an immunoassay for a specific serum protein. a. Radial Immunodiffusion One of the simplest methods for measurement of specific serum protein is radial immunodiffusion (RID). This method requires polyclonal antibody. The method is based on the precipitation in agarose gel of antigen-antibody complexes, and this does not occur with monoclonal antibodies as more than one binding site on the antigen is required for complex formation. The RID plates are prepared with agarose gel containing antibodies to the protein antigen at an optimized concentration. Sample is placed in a well in the agarose and allowed to diffuse for 24 to 48 hours. A precipitin ring forms because of the antibody-antigen reaction, the diameter of which is dependent on the analyte concentration. The concentration of the protein in the sample is determined by comparison to standards. Radial immunodiffusion assays have been used to measure immunoglobulin and complement in serum and can distinguish between the different classes of antibody (see Chapter 6), and RID assays for acute phase proteins have also been developed (Ohwada and Tamura, 1995; Tamura et al., 1989). b. Immunoturbidimetry Immunoturbidimetry (IT) and the related method of immunonephelometry also make use of the formation of antigen-antibody complexes, but in solution rather than in agarose gel. With the correct balance of antigen and antibody, the formation of antigen-antibody complexes can be followed in a spectrophotometer as flocculation occurs and absorbance increases. As the reaction takes as little as a few minutes, this is the method of choice for automation of analysis, but it is only suitable for protein concentrations above 0.5 to 1.0 mg/dl (5 to 10 mg/liter). This method is widely used in human clinical biochemistry for determination of protein such as CRP, but availability of suitable reagents has held back applications in veterinary medicine. However, IT methods for canine CRP (Eckersall et al., 1991) and feline AGP (Bence et al., 2005) have been described. Although commercial kits for human CRP based on IT have been validated for use in serum from some animal species (Kjelgaard-Hansen et al., 2003), care has to be taken in their use, especially as antiserum batches may have differing cross-reactivities with animal protein so that batch-to-batch variation may occur. Immunonephelometry is a related method where reflected rather than absorbed light is measured, which aids in reducing interference. Another modification of the IT test is to make use of antibody-coated latex particles, which can make assays more sensitive as well as stabilizing the antibodies. A method using latex particles coated with antibody to human serum amyloid A has been validated to detect this protein in horses (Jacobsen et al., 2005a; Stoneham et al., 2001). c. Enzyme-, Luminescent-, and Fluorescent-Linked Immunosorbent Assays Enzyme-linked immunosorbent assay (ELISA) are a common format for many immunoassays and are used to detect or measure a wide variety of serum analytes including steroid and protein hormones, drug residues, immunoglobulins, and pathogen antigens. They can be performed in a number of formats with antigen or antibody absorbed onto the plastic surface of microtiter plate wells and with primary or secondary antibody being conjugated to a variety of labels to allow sensitive detection. Labels that have been used include enzymes such as horseradish peroxidase or alkaline phosphatase, whereas more recent developments have replaced enzyme labeling with fluorescent or luminescent labels (Parra et al., 2005a, 2005b). These labels extend the sensitivity and reproducibility of assays. Most immunoassays for specific serum proteins are based on ELISA formats and include assays for canine CRP, porcine and bovine Hp and a cross-species SAA immunoassay that can be used in most species as the antibody shows cross-species specificity for SAA (Eckersall et al., 1989; Eckersall et al., 2001; Hiss et al., 2003; Sheffield et al., 1993; Yule et al., 1997). Immunoassays based on ELISA or related formats have been developed for low-abundance proteins found in serum that are used as biomarkers for disease in particular tissues. Thus, assays have been developed for biomarkers such as troponin I (Spratt et al., 2005) as a cardiac biomarker and trypsin-like immunoreactivity as a biomarker for pancreatic disease and intestinal malabsorption (Fetz et al., 2004; Steiner et al., 2000; Williams and Batt, 1988). d. Immunochromatography Attempts have been made to produce immunoassays in formats that can be used in practice or on a farm as point-of-care assays. Latex agglutination has been used in tests in which visible agglutination can be observed and has been used in assays for IgG for confirmation of transfer of antibody from colostrum. A more recent innovation has been the development of immunochromatography in which application of a sample to a test slide leads to diffusion of a sample and reagent along a membrane and appearance of a colored line for a positive result. A test method based on this principle for canine CRP has been produced and was assessed for identification of animals with an acute phase response (McGrotty et al., 2004). This technology should be able to produce rapid, in practice, testing for proteins, particularly when there is a large difference in concentration between health and disease states. Apart from albumin and fibrinogen (see Sections V.A and V.B), assays for a number of other serum proteins have been developed based on their chemical, physical, or biological activity. These generally have the advantage that they can be performed on automated biochemical analyzers, do not need the instrumentation required for the more sensitive immunoassays, and are usually applicable in all species. Haptoglobin, an acute phase protein, can be measured by making use of its high affinity for hemoglobin and subsequent preservation of the peroxidase activity of this protein at low pH. Interference by albumin in this assay was eliminated by use of a novel reagent, which also incorporated the chromogen (Eckersall et al., 1999). Ceruloplasmin, a copper-containing acute phase protein, can be estimated by measuring its endogenous oxidase activity (Ceron and Martinez-Subiela, 2004). Methods have been described for measuring the protease inhibitors, α1-antitrypsin and α1-antichymotrypsin (Conner et al., 1988a, 1988b, 1989), based on the specificity of their action, though these assays have not been automated. An impediment to the greater use of specific protein assays is the lack of primary reference standards for calibration, quality control material, and the availability of quality assurance schemes. None of these is presently available from commercial sources. However, the European Union has funded a project to establish reference material for bovine and porcine serum proteins and to establish a quality assurance scheme, but at the time of the project too few laboratories were running specific protein assays to enable a scheme to be viable (Skinner, 2001). As more interest in the use of specific assays expands, this could be reactivated. Laboratories running the tests routinely should prepare their own material for internal quality control. Although 289 proteins have been reported in human plasma (Anderson and Anderson, 2002), only 70 assays have been validated to the extent of reporting reference intervals. Of these, only about 10 are currently employed for diagnostic testing in domestic animals. Table 5.3 gives an overview of serum proteins, but as proteins are under genetic control, variations occur between individuals and especially between species. Biochemical and pathophysiological features of albumin and several globulins that are being used for diagnosis of disease are described next, grouped by their function(s). Albumin is the major single protein found in serum and constitutes 35% to 50% of the total serum protein. Bovine serum albumin, when synthesized and secreted by the hepatocytes, is a nonglycosylated protein of 583 amino acids with a molecular weight of 66.4 kDa and a pI of pH 5.6 (accession number P02769, UniProt database at www.ebi.uniprot.org). Based on X-ray crystallographic studies on human serum albumin, the structure is a heart-shaped protein with three homologous domains (Fig. 5.5) (Nakajou et al., 2003) containing 67% of the protein as α-helix with no β-sheet (Curry et al., 1998). A notable feature of the primary structure of albumin is that there is an odd number of cysteine residues (35 in total) so that, after the formation of 14 cysteine-cysteine internal disulfide bonds, there is a free cysteine residue, which is important for certain functions of the protein.
I. INTRODUCTION
II. CLASSIFICATION OF PROTEINS
A. Structural Classification
B. Chemical Classification
C. Physical Classification
III. METABOLISM OF PROTEINS
A. General
B. Synthesis of Proteins
C. Catabolism of Proteins
1. Turnover of Proteins
2. Urea Cycle
and COO– with part of the cycle taking place in the cytoplasm and part in the mitochondria. Abbreviations: C-P-Syn, carbomyl phosphate synthase; OCT, ornithine citrulline transferase; ArgS-Syn, argininosuccinate synthase; ArgSase, argininosuccinase; ARG, arginase.
IV. PLASMA PROTEINS
A. Sites of Synthesis
B. Functions of the Plasma Proteins
C. Factors Influencing the Plasma Proteins
1. Age
2. Hormones, Pregnancy, and Lactation
D. Handling and Identification of Proteins
V. METHODOLOGY
A. Total Protein
1. Chemical Methods
2. Physical Methods
B. Fractionation of the Serum Proteins
1. Salt, Acid, and Glutaraldehyde Fractionation
2. Dye Binding and the Albumin:Globulin Ratio
C. Electrophoretic Fractionation of the Serum Proteins
1. Principle of Electrophoresis
2. Cellulose Acetate and Agarose Electrophoresis
3. Polyacrylamide Gel Electrophoresis and Isoelectric Focusing
4. Proteomics
D. Specific Protein Analysis
1. Immunoassays for Serum Proteins
2. Biochemical Assays
3. Quality Assurance and Quality Control
VI. NORMAL PLASMA AND SERUM PROTEINS
A. Albumin
1. Biochemistry
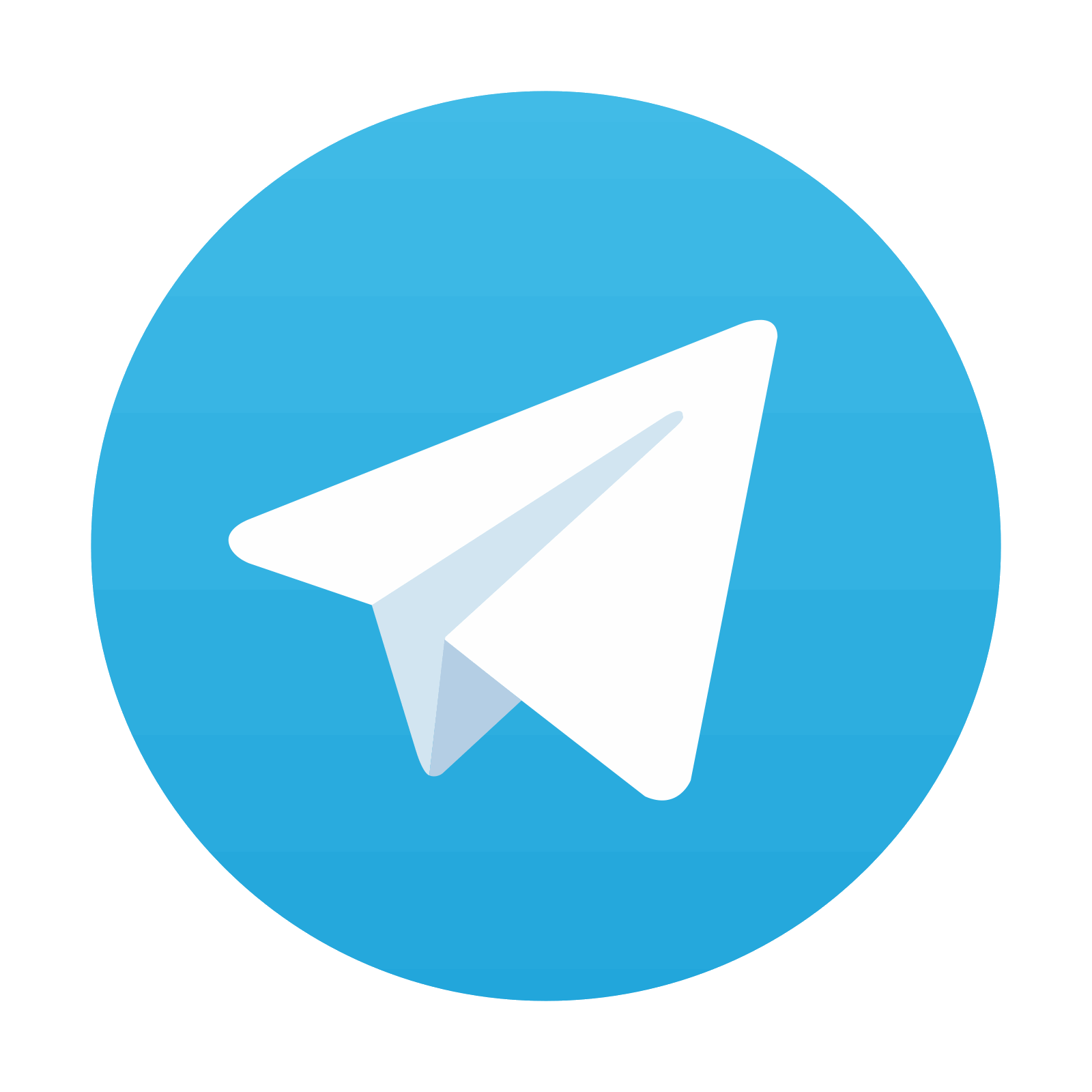
Stay updated, free articles. Join our Telegram channel
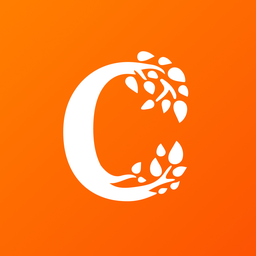
Full access? Get Clinical Tree
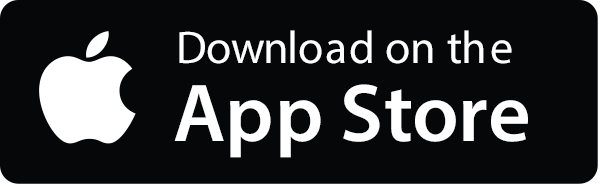
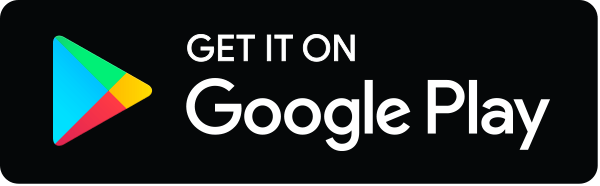