Chapter 18 Pituitary Function I. HYPOTHALAMUS-PITUITARY SYSTEM B. Regulation of Pituitary Functions II. ANTERIOR LOBE AND INTERMEDIATE LOBE A. Proopiomelanocortin-Derived Peptides IV. ASSESSMENT OF PITUITARY FUNCTION The hypothalamic-pituitary system is a preeminent example of the integration of neural and endocrine control. It consists of three major systems: (1) a neuroendocrine system connected to an endocrine system by a portal circulation, (2) a neurosecretory pathway, and (3) a direct neural regulation of endocrine secretion (Fig. 18-1). The neuroendocrine system involves clusters of peptide- and monoamine-secreting cells in the anterior and midportion of the ventral hypothalamus. Their products reach the median eminence by axonal transport. From there they are released into the capillary vessels of the hypothalamic-pituitary portal system and transported to the pituitary to regulate the secretion of hormones from the anterior lobe (AL) of the adenohypophysis (Amar and Weiss, 2003). FIGURE 18-1 Schematic representation of the relationship between the hypothalamus and the pituitary in a generalized mammal. The hypothalamus exerts control over the anterior lobe (AL) by releasing and inhibiting factors. These hormones reach the AL cells via capillaries of the pituitary portal system. The neural lobe (NL) of the pituitary is a downward projection of the hypothalamus. The intermediate lobe (IL) is under direct neurotransmitter control. In the intermediate lobe (IL) the secretory activity is regulated via direct neuronal inhibitory and stimulatory influences (Saland, 2001). In amphibians (Vazquez-Martinez et al., 2003) and reptiles (Dores et al., 1987), the IL plays an important role in adaptation to background color. The function of IL cells in mammals has not been fully established, but they may play a role in opioid-regulated functions. The hypothalamus and pituitary control vital functions such as growth, reproduction, lactation, basal metabolism, stress response, parameters of immune function, and the state of hydration. Understanding of the complicated functional relationship of the hypothalamus to the pituitary requires an appreciation of the anatomical relationships. Hypophysiotropic neurohormones are produced in several areas of the hypothalamus. For example, in an immunofluorescence study of hypothalami of dogs, the majority of cell bodies with immunoreactivity to corticotropin-releasing hormone (CRH) was found in the region of the periventricular and paraventricular nuclei, but they were also found in the supraoptic and suprachiasmatic area as well as craniodorsal to the mammillary bodies (Stolp et al., 1987). The cell bodies of the neurohormone-producing neurons that project to the median eminence are in part intermingled with cell bodies that also synthesize these neurohormones but project to other brain areas. The majority of the neurons that project to the median eminence are found in the preoptic and suprachiasmatic region of the hypothalamus. Axons containing the same neurohormone may have synaptic contacts that enable regulation of cellular function between these neurons. The neurohormone-producing cells receive a complex neural input from a variety of chemical messengers, such as neurotransmitters and other neurohormones. Not only the neurohormones CRH and arginine vasopressin (AVP), but in general the combination of a neurohormone and another chemical messenger, may colocalize within a single neuron. The neurons of the neurohypophyseal system represent a more anatomically distinct entity, with cell bodies located in the paraventricular and supraoptic hypothalamic nuclei (Sawchenko and Swanson, 1983). However, within these areas there are also neurons producing a variety of other neuropeptides. The major neurotransmitter systems for intercellular communication within the central nervous system consist of monoamines and peptides. These chemical messengers regulate the biosynthesis and release of the hypophysiotropic neurohormones. Through a network of axodendritic and axoaxonic contacts, these neurons are connected to the neurohormone-producing cells. In addition, many monoamines and peptides are found within the hypophysiotropic hormone-producing cells, where they are released together with the neurohormones into the portal system and modify the effect of hypothalamic hormones on the pituitary. The biogenic amine neurotransmitters, known to play a regulating/modulating role in the hypothalamic-pituitary system, include catecholamines (dopamine, noradrenalin, and adrenalin), indolamines (serotonin, melatonin), acetylcholine, γ-aminobutyric acid (GABA), and histamine. Neuropharmacological agents can be used to alter neurotransmitter effects and, as a consequence, hypothalamic and pituitary hormone release. Many of the peptides with potential effects on hypophysiotropic hormone release are widely distributed in hypothalamic and extrahypothalamic areas of the brain. They include, among many others, peptides common to the gastrointestinal tract, such as gastrin, cholecystokinin, and pancreatic polypeptide as well as bombesin, angiotensin II, galanin, substance P, neurotensin, enkephalins, neuropeptide Y, natriuretic peptide, the vasoactive intestinal peptide (VIP), and the peptide histidine isoleucine (PHI). The last three peptides may, through vasoconstriction and vasodilatation activities, play an important role in the control of the portal blood flow. The releasing and inhibiting hormones are stored in nerve terminals in the median eminence, where their concentrations are 10 to 100 times as great as elsewhere in the hypothalamus. The uniquely organized capillary plexus (Halasz, 1994; Page, 1986) of the median eminence is in close proximity to nerve terminals of the hypophysiotropic neurons. In contrast to other brain regions, the blood-brain barrier in the area of the median eminence is incomplete, permitting protein and peptide hormones as well as other charged particles to move to the intercapillary spaces and the nerve terminals contained therein. These terminals respond to humoral and neuronal stimuli by secreting releasing and inhibiting factors into the portal system. The portal capillaries coalesce into a series of vessels that descend through the pituitary stalk and form a second capillary plexus that surrounds the AL cells (Fig. 18-1). Inferior hypophyseal arteries supply the neurohypophysis. From the primary plexus of the neural lobe (NL), blood flows not only to the systemic circulation but also to the AL and the hypothalamus. There is evidence for some degree of circulatory flow within the pituitary, that is, from the AL to the NL, from there to the infundibulum, and then back to the AL. The primary capillary plexus of the NL appears to be well positioned in the minicirculatory system, controlling all of the afferent vascular events and many of the efferent vascular events in the pituitary (Page, 1986). FIGURE 18-2 Schematic illustration of median sections through mammalian and avian pituitaries. Key The vascularization of the intermediate lobe is closely linked to that of the neurohypophyis. In spite of the rich blood supply of the NL, the IL is a poorly vascularized structure. In horses the IL is supplied by a vast capillary or sinusoidal plexus, which connects that in the AL with that in the NL (Okada et al. 1997). During embryogenesis the adenohypophysis develops from Rathke’s pouch, which arises from the primitive roof of the mouth in contact with the base of the brain. Rathke’s pouch subsequently separates by constriction from the oral cavity. The anterior wall thickens and forms the anterior lobe of the adenohypophysis. This largest portion of the adenohypophysis remains separated from the intermediate lobe by the hypophyseal cleft, which is the residual lumen of Rathke’s pouch. In several species (Fig. 18-2), the adenohypophysis also extends into a pars tuberalis that forms a cuff or collar around the proximal neurohypophysis and may even envelop part of the median eminence (Batten and Ingleton, 1987; Hullinger, 1993). In the embryonic development, the anterior lobe undergoes major cellular proliferation and differentiation (Dubois et al. 1997). Totipotent pituitary stem cells give rise to two main cell lineages, the acidophilic (mammasomatotropic, somatotropic, lactotropic) and the basophilic (corticotropic, thyrotropic, and gonadotropic) differentiated pituitary cell types. Determination of AL cell-type lineages results from a temporally regulated cascade of homeodomain transcription factors that are being dissected by genetic and molecular approaches at a rapid pace (Treier et al. 1998). Although most pituitary developmental information has been acquired from murine models, histological and pathogenetic observations in others mammals and human subjects have corroborated these developmental mechanisms. The transcription factor Rpx (Rathke’s pouch homeobox) is the earliest known specific marker for the adenohypophyseal primordium. Rathke’s pouch expresses several transcription factors of the LIM homeodomain family, including Lhx3 and Lhx4 (Sheng et al. 1997). Lhx3 is one of the earliest markers for cells that are destined to form the AL and the IL and is required for Pit-1 expression (Sheng et al. 1996). The homeodomain transcription factor Ptx1 behaves as a universal pituitary regulator and activates transcription of α-GSU (the α-subunit of gonadotroph hormones) and POMC (Drouin et al. 1998; Lamonerie et al., 1996). Transcription factors Tpit (T box transcription factor) (Lamolet et al., 2001) and NeuroD1 (Lamolet et al., 2004) appear to be a prerequisite for POMC expression and determine the development ofthe corticomelanotrope cell lineage. TSH and gonadotropin (LH and FSH)-expressing cells share the common α-GSU expression under developmental control of GATA-2. Prop-1, the prophet of Pit-1, stimulates the Pit-1 gene. Pit-1 (or POU1F1), a POU-homeodomain transcription factor, determines the development and appropriate temporal and spatial expression of cells committed to GH, PRL, and TSH. Corticotroph cell commitment, although occurring earliest during fetal development, is independent of Pit-1-determined cell lineages. Mutations arising within these transcription factors may result in isolated or combined pituitary hormone failure syndromes. German shepherd dogs with pituitary dwarfism have a combined deficiency of GH, TSH, and PRL together with impaired release of gonadotropins, whereas ACTH secretion is intact (Kooistra et al., 2000c). The transcription factors Lhx4 (van Oost et al., 2002), Prop-1 (Lantinga-van Leeuwen et al., 2000a), Pit-1 (Lantinga-van Leeuwen et al., 2000b), and the LIF receptor gene (Hanson et al., 2006b) have been excluded as candidates for pituitary dwarfism in German shepherds and the cause remains to be elucidated. The posterior wall of Rathke’s pouch is closely apposed to the neural tissue of the NL, thereby forming the intermediate lobe (IL), which is well developed in most mammals, but not in humans and birds (Fig. 18.2). In humans only during fetal life is a distinct IL found (Amar and Weiss, 2003), whereas there is some debate about the view that in adults “invading” cells of the posterior lobe are homologous with the IL cells of lower vertebrates (McNicol, 1986). Unlike reptiles and mammals, birds have no IL (Batten and Ingleton, 1987). Nevertheless the typical “IL hormone” MSH is present in birds, having been found in the adenohypophysis, where it coexists with ACTH in cells named corticomelanotrophs (Iturriza et al., 1986). TABLE 18-1 Molecular Weights of Adenohypophyseal Hormones and Staining Properties of Adenohypophyseal Cells The pituitary stalk (infundibulum) and the neurohypophysis (posterior lobe, neural lobe) develop from the basal outgrowth of the diencephalon in connection with the development of Rathke’s pouch. The cells of the diencephalic outgrowth later develop into glial cells (pituicytes), whereas nerve fibers from the supraoptic and paraventricular nuclei grow into the NL. The peptide hormones secreted by the AL can be divided into three categories: (1) the somatomammotropic hormones growth hormone (GH) and prolactin (PRL); (2) the glycoprotein hormones thyrotropin (TSH), follicle-stimulating hormone (FSH), and luteinizing hormone (LH); and (3) the corticomelanotropins, which are all derived from the precursor molecule proopiomelanocortin (POMC), and the corticomelanotropins include adrenocorticotropin (ACTH), α-melanotropin (α-MSH), β-endorphin (β-END), and β-lipotropin (β-LPH). Identification of the adenohypophyseal cells has been developed by histochemical staining techniques, immunohistochemical methods, and ultrastructural studies. Staining and immune reactions depend on the chemical nature of the hormones that are stored in granules within the cytoplasm. A general identification of adenohypophyseal cells (Batten and Ingleton, 1987) is given in Table 18-1, although it must be realized that the various cell types do not always give exactly the same reaction in different animal species. Having undergone a number of modifications over the years to improve sensitivity and specificity, immunohistochemistry remains the single most often used method in the identification of normal and diseased AL cell types. Apart from staining for the full spectrum of hormones (GH, PRL, ACTH, FSH, LH, TSH, and α-subunit), special stains investigate the diseased AL cell, such as staining with MIB-1, a cell proliferation marker that recognizes the KI-67 antigen, or demonstration of the protein p53, the product of a tumor suppressor gene, as parameters for aggressive behavior of cells. Development of sophisticated tools of molecular biology during the 1990s has enabled researchers to study chromosomal defects, genetic abnormalities such as specific mutations, the presence of oncogenes or of tumor suppressor genes, cell membrane receptors, signal transductions mechanisms, and intracellular changes that affect hormone synthesis and release. Pituitary cell cultures (normal and diseased, e.g., murine AtT-20 corticotrope cell line) allow various in vitro studies to be undertaken. In situ hybridization demonstrates specific messenger RNAs (mRNAs) at the subcellular level, which allows the reliable assessment of gene expression. In situ hybridization can be used to demonstrate the mRNA of receptors, growth factors, transcription factors, genes, and other regulatory peptides. Whereas immunocytochemistry provides information regarding cell hormone content, in situ hybridization confirms the presence of ongoing hormone synthesis. Transmission electron microscopy allows the study of AL cells at the ultrastructural level. Applications of immunohistochemical methods at the ultrastructural level have also contributed to the rapid progress in the field. Ultrastructural studies of AL cells demonstrate the presence of distinct varieties of secretory granules and typical organization of cytoplasmic organelles for peptide synthesis and release. Characteristics such as the form, size, and location of the various organelles and the size and shape of the cells and their nuclei allow identification of adenohypophyseal cells at the ultrastructural level (Batten and Ingleton, 1987; Mikami, 1986). The distribution of the various secretory cells of the AL is not random, different hormone-secreting cells preferentially accumulate at different sites in the AL. This topological organization of the AL is well known in the human and may also be true for domestic animals. The gland comprises a central “mucoid” wedge containing thyrotropes and corticotropes and lateral wings containing somatotropes and lactotropes. The gonadotropes are distributed diffusely throughout the gland. Also, the numerical contribution of each AL cell type is not the same. In the pituitary anterior lobe of humans the distribution is corticotrope (10% to 15%), thyrotrope (5%), somatotrope (50%), lactotrope (10% to 30%), and gonadotrope (15% to 20%). The concept of one pituitary cell making one hormone is an oversimplification. Immunoelectron microscopy has shown that one hormone can be produced by the same or different cell populations. In some instances, multiple hormones are produced by the same cell population and have even been visualized within the same secretory granule. The majority of gonadotropes and somatomammotropes is multihormonal cells that contain LH and FSH or GH and PRL, respectively. Also, other combinations such as gonadotroph hormones and ACTH or TSH and ACTH have been described. In addition to the functionally distinctive AL cell types noted above, cells termed “stellate” or “folliculostellate” also occur within the normal pituitary. Folliculostellate cells lack secretory granules as well as hormone secretion and are characterized by processes that insinuate themselves between the AL cells. The physiological role of folliculostellate cells is uncertain. They are thought to be supportive in nature and synthesize a variety of chemical messengers, intrapituitary growth factors, and cytokines that exert local paracrine effects on cell function and proliferation (Denef, 1994). The predominant IL cell in mammals is the melanotrope, a cell with immunoreactivity for α-MSH, which is sparse in the AL (Halmi and Krieger, 1983). In some species, including the dog (Halmi et al., 1981) and the horse (Amann et al., 1987), the IL is cytologically heterogeneous. ACTH-containing cells (B cells) have been found to be dispersed among the predominant melanotropes (A cells). In the dog, the immunoreactive ACTH content of the IL even exceeds that in the AL (Halmi et al., 1981). In ferrets, as in dogs and cats, the melanotropic cell was the most abundant cell type of the IL (Schoemaker et al., 2004). In agreement with the previously mentioned direct neural regulation of IL secretory activity, the presence of neural elements was demonstrated in the bovine IL (Boyd, 1987). Evidence is accumulating that the cells of the pars intermedia are also involved in the biosynthesis and release of a yet unknown prolactin-releasing factor. The blood supply of the IL is poor, and therefore the peptides released from the IL are thought to mainly act by diffusion in a paracrine manner. The NL contains axonal nerve fibers, often swollen by being packed with neurosecretory granules. These nerve fibers and the glial cells (pituicytes) have synaptoid contacts. The pituicytes play an intermediary role in the regulation of the release of vasopressin and oxytocin (Rosso et al., 2004). For the regulation of each of the five major adenohypophyseal hormone systems (ACTH, LH and FSH, TSH, GH, and PRL), there is a feedback (closed-loop) system. AL hormone and hypophysiotropic hormone secretions are suppressed by the products of target endocrine glands such as the thyroid, adrenals, and gonads. Apart from this long-loop feedback, some hormones (e.g., PRL) regulate their own secretion directly by acting on the hypothalamus (short-loop feedback). On this powerful feedback control with primarily blood-borne signals, other signals are superimposed. These may originate within the central nervous system (open loop) and can be mediated through neurotransmitters and hypophysiotropic hormones. Thus, influences are exerted that represent the environment (temperature, light-dark), stress (pain, fear), and intrinsic rhythmicity. These regulatory factors influence peptide synthesis or release in adenohypophyseal cells, where each of the steps in hormone synthesis and ultimate secretion represents a potential control point in the regulation of circulating hormone concentrations. The main hypothalamic neurohormones may stimulate or inhibit the release of a single hormone, or it may affect several hormone-producing cells (Fig. 18.3). The predominant influence of the hypothalamic hormones on the pituitary is stimulatory, and these peptides are known as releasing hormones (Guillemin, 2005). Interference with the integrity of the hypothalamic-pituitary connections results in decreased secretion of pituitary hormones. The exception is PRL, the secretion of which is increased when hypothalamic influence is removed. As the complexity of the peptide structures of the hypophysiotropic hormones increases, species variation in sequence may occur. Whereas the structures of TRH, GnRH, and somatostatin (SS) (3, 10, and 14 amino acids, respectively) are identical for mammals, those of GHRH and CRH (44 and 41 amino acids) exhibit species specificity. The one nonpeptide hypophysiotropic hormone is dopamine. In addition to its major role as a neurotransmitter, dopamine is the most important inhibitor of PRL release. FIGURE 18-3 Hypophysiotropic regulation of the secretion of pituitary hormones. Solid lines denote hormones whose structures have been determined. Dashed lines indicate a factor whose identity is still unknown. The developments in recombinant DNA technology have enabled an increased knowledge on the genes encoding pituitary hormones and the regulatory elements involved in gene transcription. In short, the main elements regulating the gene transcription will be mentioned (Fig. 18.4). Eukaryotic genes encoding peptide hormones consist of a promoter (regulatory) unit and a transcription unit encoding the primary transcript that after appropriate processing will form the messenger RNA (mRNA). FIGURE 18-4 Schematic representation of potential control points in pituitary hormone synthesis and secretion. The promoter unit is the upstream part (5′) of the gene. The promoter has specific DNA sequences (response elements) permitting the binding of transcription factors that enhance or inhibit gene expression by changes in the stability of the RNA polymerase-TATA box complex at the constitutive promoter. Also, with a higher distance to the gene-specific enhancer elements, or locus control regions as seen with GH expression (Ho et al., 2006), may regulate gene transcription. Tissue-specific silencers and enhancers that are mandatory for gene expression regulate the highly specialized hormone production. Methylation patterns of the promoter may also strongly influence the overall expression level (Newell-Price, 2003). Tissue-specific intracellular transcription factors play a crucial role in the regulation, whether a gene comes to expression. Specific binding of neurohormones from the hypothalamus to membrane receptors of individual AL cells will result in changes in intracellular second messenger concentrations or activity, a process called signal transduction. Using microarray technology important signaling pathways for pituitary hormone expression can be revealed (Ma et al., 2005). Activation of these pathways results in differences in phosphorylation of transcription factors that may bind to a response element (RE) in the promoter unit. Steroid hormones, thyroid hormone, and retinoids will, after binding to specific cytoplasmic or nuclear localized receptors, induce receptor binding to specific areas of the promoter. For example, a glucocorticoid response element (GRE) in the promoter of the gene encoding proopiomelanocortin binds the glucocorticoid-receptor complex resulting in inhibition of gene transcription (negative GRE). Transcription is thus regulated by responses to extracellular signals, which may also be derived by components of the extracellular matrix (ECM) (Paez-Pereda et al., 2005). After transcription has been initiated, a primary transcript is made containing an RNA copy of the entire transcription unit, the heteronuclear RNA (hnRNA). After excision of the intron areas, a process called splicing, a cap formation at the 5′ end and the addition of a poly(A) tail at the 3′ end a mature mRNA is formed. Through alternative splicing reactions, length variants of the mRNA may ultimately result in variation of the coding sequence (see Section II.C.1), changes in mRNA stability as found for the insulin-like growth factors (IGFs), or by differences in exon coupling even completely different peptides can be obtained from a single gene as for instance in the gene encoding calcitonin. The process of peptide synthesis occurs principally on the rough endoplasmic reticulum (RER). Messenger RNA (mRNA), encoded by nuclear DNA, passes to cytosolic ribosomes, whereby sequential processing of transfer RNAs (tRNA), with their attached amino acids, the translation process of mRNA to a peptide starts (Fig. 18.4). The beginning of the growing peptide forms a specific signal peptide that facilitates the attachment of the translation complex to the RER and enables the passage into the lumen of the RER. The signal sequence of the preprohormone is cleaved, and the remaining prohormone undergoes several modifications as disulfide formation and glycosylation. The peptide passes along the RER lumen into the Golgi complex, where peptides are packaged and released into the cytoplasm as membrane-bound granules. During storage of these granules, the prohormone is further processed by specific proteolytic cleavage, C-terminal amidation, or N-terminal carboxylation. Characteristic for proteolytic cleavage sites are pairs of the basic amino acids arginine and lysine. The granules are stored until the hormone is released by exocytosis. This process involves fusion of the granule membrane with the cell membrane. The corticotropic cells of the AL and the melanotropic cells of the IL are both able to synthesize proopiomelanocortin (POMC), the common prohormone for ACTH, α-MSH, β-lipotropin, and a family of β-endorphin-related peptides (Fig. 18-5). FIGURE 18-5 Schematic representation of preproopiomelanocortin (horizontal bar), with four domains: the signal peptide, which is cleaved after entrance to the lumen of the RER; the N-terminal peptide (N-POC), containing the (pro) γ-MSH sequences and the joining peptide (JP); the ACTH domain from which MSH and corticotropin-like intermediate lobe peptide (CLIP) is generated; and the β-lipotropin (β-LPH) domain, including the endorphin (END) family of peptides and a metenkephalin sequence (Enk). Pairs of basic amino acid residues are indicated with vertical lines, representing potential sites of proteolytic cleavage. In the AL major cleavage products are N-POC, ACTH, and β-LPH. In the IL the major products are N-POC, γ-MSH, α-MSH, and β-endorphin. FIGURE 18-6 Comparison of the ACTH sequences from various species using the one-letter code for amino acids. Identical amino acids are given by a single line (-). The asterisk (*) means an identical sequence for all species. The shaded box indicates the two pairs of basic amino acids prone to proteolytic cleavage. The insert shows a dendrogram indicating the mutual relationship. a. Gene Expression The gene encoding POMC contains three exon areas. Exon 1 encodes a 5’ untranslated region (5′-UTR), and exon 2 encodes the signal peptide and the N-terminal part of the POMC prohormone. The majority of the prohormone, including γ-MSH, is encoded by exon 3. After the splicing process, an mRNA of approximately 1150 nucleotides is formed in the pituitary of most species, including the dog (Mol et al., 1991). In many peripheral tissues, very low expression of shorter POMC mRNA sequences are found, whereas in nonpituitary tumors, an mRNA of 1350 nucleotides can be found (Newell-Price, 2003). The presence of an unmethylated CpG island in the POMC promoter is a prerequisite for gene expression, whereas methylation completely blocks gene expression (Newell-Price, 2003). These methylation patterns are set during early development. Synergistic stimulation of gene expression requires binding of yet partially characterized transcription factors in the distal and central part of the POMC promoter. The expression of the POMC gene in corticotropes and melanotropes is regulated by the same promoter elements as shown by experiments with transgenic mice (Tremblay et al., 1988). Three important response elements act within the central promoter region. The pituitary homeobox 1 (Ptx1) protein, which is widely expressed in the pituitary, acts in synergy with the corticotrope/melanotrope-specific factors NeuroD1 (Newell-Price, 2003) or Tpit (Quentien et al., 2006) to stimulate POMC gene expression. Binding of corticotropin-releasing hormone (CRH) stimulates POMC expression in the PD through stimulation of cAMP within the cell (Jacobson and Drouin, 1994). CRH acts synergistically with the leukemia-inhibitory factor (LIF) through binding of the cAMP response element binding protein (CREB) at the response element sites for NUR77 and STAT1-3 (Mynard et al., 2004). Nur77 is an orphan nuclear receptor that antagonizes the negative feedback of glucocorticoids on POMC expression (Martens et al., 2005). POMC expression in the IL melanotrope is also stimulated by cAMP. In vitro experiments in various species point to a possibility that CRH activates this signal transduction system. However, chronic infusion of CRH stimulates the POMC mRNA concentrations in the AL but inhibits mRNA concentration in the IL (Hollt and Haarmann, 1984). IL POMC expression is also regulated by stimulation with serotonin and norepinephrine or GABAergic and dopaminergic inhibition (Saland, 2001). Glucocorticoids inhibit AL POMC expression but have no effect on the IL expression of POMC. Extrapituitary POMC expression is found in many tissues (DeBold et al., 1988), also in skin tissue of various species including the dog (Kidney et al., 2004). b. (Pro)hormone POMC prohormone sequences are known for a variety of species and share a common structure (Fig. 18.5). Among species a high sequence homology is found between the N-terminal site (N-POC) of the prohormone (including γ-MSH), the ACTH region, and the β-endorphin region. In contrast, the regions between γ-MSH and ACTH and the first part of β-lipotropin are rather heterogeneous (Mol et al., 1991; Numa and Imura, 1985). Species differences in the sequence of ACTH occur only in the N-terminal part (Fig. 18.6), whereas the ACTH(1-24) sequence, necessary for full biological activity, is identical among mammals. The specificity of immunoassays to measure ACTH concentrations is achieved by directing antibodies toward the ACTH (13-18) epitope, which is absent in MSH and corticotropin-like intermediate lobe peptide (CLIP). These antibodies do, however, cross-react with intact POMC and may result in apparent elevated ACTH concentrations (Goossens et al., 1995). Two-sided assays are more sensitive to variation in the carboxy-terminal part and show insufficient cross-reactivity among the species. The proteolytic enzymes PC1/PC3 and PC2 are involved in the processing of prohormones in neuroendocrine cells. They cleave the precursor at pairs of basic amino acids, resulting in the formation of biologically active hormones. The PC1/PC3 enzyme present in the AL cleaves POMC to ACTH and β-LPH. PC2 generates the ACTH(1-13) fragment in both the AL and IL. The combination of both enzymes is present in the melanotropes of the IL. In the hypothalamus, these enzymes determine also the availability of POMC-derived melanocortins, which play an essential role in the regulation of energy balance (Bertile and Raclot, 2006; Helwig et al., 2006). In the melanotrope α-MSH is formed by C-terminal amidation and N-terminal acetylation of ACTH(1-13). Various degrees of acetylation of MSH result in the storage of desacetyl-, monoacetyl-, and diacetyl-α MSH in secretory granules. The canine IL contains predominantly the monoacetyl-α MSH (Young et al., 1992). c. Secretion by the AL ACTH is released in frequent pulses, as demonstrated in the pituitary venous effluent of the horse (Alexander et al., 1994; Redekopp et al., 1986a, 1986b). By measurements in peripheral blood (Kemppainen and Sartin, 1984), the episodic secretion of ACTH in dogs was documented, with an average of nine peaks per 24-hour period. Many factors influence the secretion of ACTH by the AL (Table 18-2). A number of these factors modulatethe release of CRH and AVP, which are considered to be thepredominant stimulating neurohormones in vivo (Antoni, 1986; Keller-Wood and Dallman, 1984). The relative contribution of CRH and AVP to ACTH release varies among species and circumstances. In dogs (van Wijk et al., 1994) and pigs (Minton and Parsons, 1993), both exogenously administered CRH and LVP induce comparably high plasma ACTH concentrations. In sheep, AVP is the predominant ACTH-releasing factor. In horses, AVP is the immediate stimulus for ACTH release, and even ACTH micropulses appear to be regulated by AVP set point (Alexander et al., 1996). On the other hand, CRH secretion and pituitary responsiveness to CRH rise when cortisol falls, suggesting that in horses a major role for CRH is to fix the cortisol set point (Alexander et al., 1996). In the rat, the stimulation of ACTH release by CRH and AVP is synergistic, meaning that the response to CRH + AVP is greater than the sum of the reactions to CRH and AVP separately (Buckingham, 1987). The basal release of ACTH is regulated by the occupancy of type I or mineralocorticoid receptors (MR) in the hippocampus (Jacobson and Sapolsky, 1991). Decreases in brain MR binding capacity of the dog during aging results in enhanced basal activity of the hypothalamus-pituitary-adrenal axis (Rothuizen et al., 1993). In sheep, basal ACTH release is inhibited by active immunization against AVP (Guillaume et al., 1992a) but not by immunization against AVP (Guillaume et al., 1992b). Exercise and insulin-induced hypoglycemia stimulate ACTH release. In the horse, exercise-induced ACTH release and mild insulin-induced hypoglycemia are accompanied by increased AVP concentration in pituitary venous blood without changes in CRH concentrations (Alexander et al., 1991). However, when hypoglycemia becomes severe, CRH is released, which augments the ACTH response (Alexander et al., 1996, 1997). In sheep, mild hypoglycemia is accompanied by increases in AVP and CRH concentrations in portal blood, but in severe hypoglycemia the AVP secretion is relatively much higher than the CRH release (Caraty et al., 1990). AVP also regulates the ACTH response on hypoglycemia in the neonatal rat (Muret et al., 1992). Hypotension induced by nitroprusside (Kemppainen and Sartin, 1987) or by hemorrhage (Lilly et al., 1983) causes ACTH release in the dog, together with large increases in plasma AVP derived from the NL (Raff et al., 1989). Selective neurohypophysectomy results in greatly attenuated ACTH and AVP responses to hypotension and angiotensin II, whereas the ACTH response to CRH injection remains unchanged. By substitution with adequate AVP infusion, the ACTH response to hypotension can be restored (Raff et al., 1992). In sheep, chronic absence of ovarian hormones after ovariectomy reduces the ACTH response to hypotension also, but not to CRH, AVP, or hypoglycemia (Pecins-Thompson and Keller-Wood, 1994). In the dog, the release of ACTH is stimulated by β-adrenergic agonists (isoproterenol), dopaminergic antagonists (haloperidol), and serotoninergic agonists (quipazine maleate). TRH and GnRH stimulate the release of cortisol, probably by stimulating ACTH release (Stolp et al., 1982). In the horse, the α2-adrenergic agonist clonidine lowers ACTH secretion primarily by reducing the secretion of AVP and possibly CRH (Alexander and Irvine, 2000). Although direct stimulatory effects of catecholamine on the in vitro release of ACTH from ovine pituitary cells have been found, central stimulation of predominantly noradrenergic, but also adrenergic, pathways evokes the highest ACTH response (Liu et al., 1991). Endogenous opiates (metenkephalin, dynorphin, and β-endorphin) inhibit the release of ACTH in humans (Besser et al., 1987). Conflicting results have been reported on the effect of metenkephalin in the rat, but β-endorphin and dynorphin may exert tonic inhibition of CRH release (Plotsky, 1986). The metenkephalin agonist DAMME stimulates the release of ACTH in the dog (Meij et al., 1990). Endogenous corticosteroids inhibit ACTH release predominantly at hypothalamic sites. Synthetic steroids such as dexamethasone may act primarily at the pituitary level (de Kloet et al., 1974). In a review on corticosteroid-mediated feedback, Keller-Wood and Dallman (1984) suggested three different time schedules: a fast feedback that acts on the corticotropic cell but may not be related to nuclear receptor binding, an intermediate feedback that probably acts by inhibition of CRH release, and a slow feedback that acts by a decrease in mRNA encoding POMC in the pituitary gland. The delay in inhibiting ACTH production may be caused by the high stability of the mRNA encoding POMC and not by the absence of direct inhibition of the transcription. Dexamethasone inhibits gene transcription in vivo within 30 min in the rat (Fremeau et al., 1986). In the dog, the intermediate-delayed feedback is determined by the mean change in corticosteroid concentration over time (Keller-Wood, 1989). Acute lowering of plasma cortisol in the horse by inhibition of synthesis in the adrenal gland resulted in an increased ratio of ACTH:CRH in pituitary venous blood before CRH concentrations started to rise (Alexander et al., 1993, 1996), indicating that the first effect is the opposite of the fast feedback and is mediated by increased sensitivity of the corticotrope. d. Secretion by the IL The release of POMC-derived peptides by the IL is under direct neural control. The rat and the mouse have been the mammals in which most studies on the regulation and processing of POMC in the IL have been carried out thus far. In the rat, the release of POMC-derived peptides is regulated predominantly via tonic dopaminergic inhibition and β-adrenergic stimulation (Berkenbosch et al., 1981; Tilders et al., 1985), although GABAergic innervation of the IL has also been demonstrated (Oertel et al., 1982). In addition, Proulx-Ferland et al., (1982) demonstrated that CRH is a potent stimulator of α-MSH secretion by the IL. In line with the absence of a glucocorticoid receptor in the IL (Antakly et al., 1985), the α-MSH response to CRH could not be suppressed by dexamethasone administration. The expression of the glucocorticoid receptor in the IL is suppressed by dopamine (Antakly et al., 1987), whereas the CRH receptor content of the rat IL is stimulated by dopamine (Shiver et al., 1992). In the dog, in vitro studies (Mol et al., 1987) and in vivo and immunohistochemical observations (Middleton et al., 1987b) have revealed the IL to be resistant to glucocorticoid suppression. There is also evidence from in vivo studies that dopaminergic pathways play a regulatory role in canine IL function (Kemppainen and Sartin, 1986). However, in other respects the situation in the dog is different from that in the rat with regard not only to the heterogeneous cytology (see Section I.A.5) but also to some of the regulation characteristics. Despite the fact that CRH-immunoreactive fibers have been identified in the canine neurointermediate lobe (Stolp et al., 1987) and although in vitro CRH stimulates ACTH release from the neurointermediate lobe (Mol et al., 1987), there is no convincing evidence that CRH can stimulate release of ACTH from the IL in vivo (Kemppainen and Sartin, 1986, 1987; Middleton et al., 1987a), whereas no (Kemppainen and Sartin, 1986) or a very small (Rijnberk et al., 1987) α-MSH response to CRH stimulation has been observed. Kooistra et al., (1997a) showed that α-MSH is secreted in a pulsatile manner in the dog. In contrast, with a significant increase of plasma α-MSH concentrations after administration of the dopamine antagonist haloperidol, even after pretreatment with dexamethasone to inhibit the contribution of the AL, there were small increases in the plasma concentrations of ACTH and cortisol, which suggests that the canine IL contributes to circulating ACTH concentrations (Kooistra et al., 1997a). The cat has an actively secreting IL, which is reflected in high plasma concentrations of α-MSH and β-endorphin, POMC-derived peptides secreted predominantly by melanotropes (Peterson et al., 1994). In the cat as well as in the dog, no stimulation of α-MSH occurs after CRH administration (Willemse and Mol, 1994). However, cats undergoing handling and skin testing without anesthesia show significant increases in plasma α-MSH concentrations (Willemse et al., 1993). In vitro experiments revealed the sensitivity of feline IL MSH release to dopaminergic inhibition (Willemse and Mol, 1994). In fetal and newborn lamb and in adult sheep (Newman et al., 1987), the administration of a dopamine-receptor antagonist results in α-MSH release. Elimination of the inhibitory hypothalamic control in sheep by hypothalamus-pituitary disconnection results in increased α-MSH release (Clarke et al., 1986). The dopamine inhibition of ACTH secretion in the hyperadrenocorticoid horse (Wilson et al., 1982) suggests that in the normal horse the IL is under dopaminergic control. In the rabbit the dopaminergic control of the IL is absent (Schimchowitsch et al., 1986). e. Action The predominant action of ACTH is stimulation of steroidogenesis and corticosteroid release from the adrenals (see Chapter 19 on adrenal function). ACTH also exerts a growth-stimulating effect on the adrenal cortex. Moreover, non-ACTH portions of POMC—that is, N-terminal POMC peptides—are involved in adrenocortical growth (Lowry et al., 1987). In pharmacological dosages, ACTH may promote lipolysis in fat cells and amino acid uptake in muscle. The role of intact ACTH produced in hypothalamic neurons projecting to higher brain centers remains to be elucidated. Distribution patterns have been reported for the cat (Rao et al., 1986). The C-terminal fragment of ACTH (18-39), called corticotropin-like intermediate lobe peptide (CLIP), has been found a potent stimulator of in vitro adrenal DNA synthesis (Wulffraat et al., 1987). Nowadays five different receptors for the melanocortins have been cloned with variable affinities for ACTH(1-39), α-MSH, and γ-MSH and related peptides. The MC2 receptor is unique because only ACTH(1-39) activates this receptor. The receptor is present in the adrenal cortex of many species and is also shown in adipocytes of mice. The other receptors, MC1R and MC3R, have been found to be expressed throughout the body, whereas MC4R is only found within many brain regions and MC5R only in peripheral tissues. Through these five receptors, the melanocortins play a role in many biological functions such as skin physiology, pain and nerve regeneration, behavior, obesity and energy metabolism, and inflammatory and immune processes (Getting, 2006). The melanocortin system plays a role in regulating feeding behavior and energy balance (Bertile and Raclot, 2006). In the dog a single mutation in the MC1R gene results in a premature stop codon and is associated with the yellow coat color in Labrador retrievers (Everts et al., 2000). f. Disease Lesions at the hypothalamic and pituitary level may result in altered synthesis and release of POMC-derived peptides. There have been no reports of the occurrence of isolated ACTH deficiency in domestic animals. There are a few reports of dogs with tumorous (supra)hypophyseal lesions, with indirect evidence for multiple adenohypophyseal and neurohypophyseal deficits (Eigenmann et al., 1983b; Rijnberk, 1971), and one dog reported to have secondary hypoadrenocorticism without information about other pituitary functions (Peterson et al., 1992). In contrast to the few descriptions of ACTH deficiency, pituitary-dependent hyperadrenocorticism is a common disorder in the dog (Hanson et al., 2005; Meij et al., 2002; Peterson, 1987) and rare in the cat (Meij et al., 2001). The adenomas producing the ACTH excess may originate in the AL or IL (Peterson et al., 1986). Cats with pituitary-dependent hyperadrenocorticism usually have concurrent insulin-resistant diabetes mellitus. Various pituitary neoplasm’s have been described in cats such as AL and IL corticotropic adenomas (Meij et al., 2001), corticotropic and somatotropic adenoma (double adenoma) (Meij et al., 2004), and melanotropic adenoma (Meij et al., 2005). Dogs with pituitary-dependent hyperadrenocorticism had significantly lower CRH concentrations in cerebrospinal fluid compared to control dogs indicating that the excessive ACTH secretion is not caused by chronic hyperstimulation with CRH (Van Wijk et al., 1992). The ACTH secretion appeared also to be less sensitive to stimulation with CRH than with LVP (van Wijk et al., 1994). The expansion of pituitary corticotroph adenomas in dogs is correlated with insensitivity to glucocorticoid feedback (Kooistra et al., 1997b) and plasma concentrations of ACTH precursors (Bosje et al., 2002). Evidence for a genetic involvement in tumorigenesis was found in a family of Dandie Dinmont terriers (Scholten-Sloof et al., 1992). In the horse, the disease originates primarily in the IL (Heinrichs et al., 1990; Orth et al., 1982; van der Kolk et al., 2004; Wilson et al., 1982). In agreement with the characteristics described earlier for the secretion of POMC-derived peptides by the IL, ACTH release by tumors of IL origin in both the dog and the horse tends to be strongly resistant to suppression by dexamethasone (Orth et al., 1982; Peterson et al., 1986). In dogs, the highest plasma α-MSH concentrations are found in individuals with dexamethasone-resistant ACTH secretion (Meij et al., 1997b). This suggests an IL origin of the disease, although there is evidence that the pituitary lesions do not always maintain the characteristics of the lobe of origin (Rijnberk et al., 1988b). A dog with diabetes insipidus has been described, in which the pituitary tumor released primarily biologically inactive POMC-derived peptides (Goossens et al., 1995). In contrast with the equine IL tumors, these tumors in dogs respond poorly to administration of dopamine agonists in terms of diminished ACTH secretion (Rijnberk et al., 1988b). A dog has been described that had dexamethasone-resistant hyperadrenocorticism and elevated ACTH secretion resulting from multiple endocrine neoplasia—that is, a pituitary corticotropic tumor, bilateral adrenocortical tumors, and pheochromocytoma (Thuroczy et al., 1998). For details on clinical manifestations, laboratory findings, diagnostics, and treatment of these diseases, including iatrogenic hypoadrenocorticism resulting from corticosteroid therapy, refer to textbooks by Feldman and Nelson (2004) and Rijnberk (1996) and Chapter 19 on adrenocortical function in this volume. g. Tests Basal levels of circulating POMC-derived peptides are measured for diagnostic purposes in situations of suspected hypo- as well as hypersecretion. ACTH values below or just within the reference range (Table 18-3) may be found in cases of hypothalamus-pituitary disease, as well as in situations where endogenous or exogenous glucocorticoid excess suppresses hormone synthesis in the corticotropic cells. This makes the measurement of basal ACTH levels a useful tool in the differentiation between pituitary-dependent hyperadrenocorticism and hyperadrenocorticism resulting from adrenocortical tumor (Feldman, 1983; Peterson, 1986). In pituitary-dependent hyperadrenocorticism, ACTH values exceeding the reference range may be found, but there is considerable overlap (Meij et al., 1997a). Basal MSH concentrations have been found to be elevated in horses (Orth et al., 1982) and dogs (Meij et al., 1997b; Peterson et al., 1986; Rijnberk et al., 1987) with pituitary-dependent hyperadrenocorticism of IL origin. In the horse, basal plasma ACTH concentration predicts a pituitary adenoma of the IL with 100% sensitivity (van der Kolk et al., 1995). TABLE 18-3 Basal Concentrations of POMC-Derived Peptides in Plasma of Healthy Animals a a Most of the values have been converted to SI units, employing: 1 ng ACTH/liter = 0.22 pmol/l; 1ng α -MSH/liter = 0.60 pmol/l; 1ng β -END/liter = 0.29 pmol/l. Of the dynamic tests, the dexamethasone suppression tests (see Chapter 19 on adrenocortical function) are still the best for the diagnosis and differential diagnosis of excessive ACTH and glucocorticoid secretion. In animals suspected of having pituitary-adrenocortical insufficiency, the secretory capacity for POMC-derived peptides can be measured by provocative testing. CRH and AVP are also used in the differentiation between pituitary-dependent hyperadrenocorticism and hyperadrenocorticism resulting from adrenocortical tumor. The chronically suppressed corticotropic cells are presumed to be unresponsive to these stimuli. However, Meijer et al., (1978) found considerable overlap in plasma cortisol value in the AVP test, whereas much less is claimed to occur in the CRH test (Peterson, 1986). It has been demonstrated that LVP stimulates cortisol release by adrenal tumors in a direct way (van Wijk et al., 1994). It has been shown that dogs with pituitary-dependent hyperadrenocorticism usually remain responsive to single CRH administration (Rijnberk et al., 1987) or combined hypophysiotropic stimulation in spite of persisting hypercortisolism and neoplastic transformation of the corticotropes (Meij et al., 1997a). The cells of the pituitary lesions were found to be less responsive to CRH in vitro than were normal corticotropes (Mol et al., -1987) and less sensitive to inhibition by glucocorticoids (van Wijk et al., 1998). As far as the possibility for manipulation of canine IL function is concerned, of the substances tested only the dopamine agonist haloperidol (Kemppainen and Sartin, 1986; Rijnberk et al., 1987) caused significant increases in circulating α-MSH. More detailed information pertaining to some of these tests is presented in Section IV and in Table 18-4. a. Gene Expression and Biosynthesis Both β-lipotropin and β-endorphin are derived from the precursor protein POMC. The gene expression of POMC has been described in Section II. A.1. β-Lipotropin β-LPH) consists of the 91 C-terminal amino acids of the POMC precursor. It is synthesized in the corticotropic cells of both the AL and IL. The C-terminal sequence (36-91) is remarkably similar in human, porcine, and ovine pituitaries, whereas the N-terminal sequence (1-36) is rather heterogeneous. β-MSH (sequence 37-58) appears to be an extraction artifact and plays no physiological role. By proteolytic cleavage at amino acid 61, γ-LPH (β-LPH[1-61]) and a family of endorphins are formed. Through specific deletion of C-terminal amino acids and N-terminal acetylation, a variety of β-END-related peptides are formed. The three main compounds are β-END(61-91), γ-END(61-77), and α-END(61-76). The corticotropic cells of the AL synthesize approximately equal or higher concentrations of β-LPH than of β-END(1-31) in most species. The equine AL, however, contains primarily β-END(1-31) and N-acetyl-β-END(1-27) (Millington et al., 1992). The corticotropic/melanotropic cells of the IL produce relatively more β-END and related peptides. In the IL of the normal dog, the ratio β-LPH/β-END is less than 0.1 (Krieger, 1983). In the dog, β-END(1-27) is the most abundant form in the IL (Young and Kemppainen, 1994). b Secretion The stimuli that induce ACTH or α-MSH release from either the AL or IL cells cause also a release of the β-LPH-related peptides from the same cell. The cellular subset of specific proteolytic, amidating, and acetylating enzymes present in the secretory vesicles defines the ultimate composition of POMC-derived peptides that are secreted into the blood. The secretion of the IL is mainly under control of multiple neurotransmitters (Saland, 2001). In fearful dogs, only marginal increases in plasma β-END were measured after a gunshot test (Hydbring-Sandberg et al., 2004). In horses, a critical exercise threshold exists before plasma β-END concentrations increase (Mehl et al., 2000). c Action The main action of β-LPH is to mobilize fat from adipose tissues, as demonstrated in the rabbit. Its biological function in humans and other species has not been fully elucidated. Brain tissue can break down β-LPH to form β-END-related peptides. However, it is questionable whether these pituitary peptides are involved in brain function; in conscious sheep, hemorrhagic stress elevates β-END concentrations in plasma but not in cerebrospinal fluid (Smith et al., 1986). β-END is an endogenous opiate with a potent morphinomimetic action. It is also produced in the hypothalamus where the entire POMC precursor is present. Brain and pituitary endorphin are probably part of separate systems. The role of β-END and other opioid peptides in the secretion of pituitary hormones has been studied with long-acting analogues, opiates, and opioid receptor antagonists (Estienne and Barb, 2005; Molina, 2006). d. Disease/Tests Hypersecretion of β-END is associated with the ACTH hypersecretion of IL tumors in both equine and canine pituitary-dependent hyperadrenocorticism (Orth et al., 1982; Peterson et al., 1986; Rijnberk et al., 1987). As the regulation of the secretion of β-END is similar to that of other POMC-derived peptides from the AL and IL, testing procedures can be applied as mentioned in the section on ACTH and α-MSH. Elevation of plasma β-END has been documented in horses following running and shipping (Li and Chen, 1987) and in sheep during electroimmobilization and shearing procedures (Jephcott et al., 1987). Treadmill exercise of Thoroughbred horses induces a variable response in plasma β-END concentrations (Art et al., 1994). Elevated plasma β-END concentrations are found in dogs with congestive heart failure (Himura et al., 1994) and are further elevated after naloxone treatment. The gonadotropes and thyrotropes synthesize the hormones LH, FSH, and TSH, each of which consists of two different peptide chains, termed the α– and β-subunits. The amino acid sequence of the α-subunit is identical for LH, FSH, and TSH within a species, as it is for placental chorionic gonadotropin. The β-subunits have unique structures and determine the hormone specificity. Carbohydrate substituents account for 10% to 20% of the molecular weights of these hormones. a. Gene Expression The gene encoding the α-glycoprotein subunit (αGSU) varies between 8 and 16.5 kb among the species and contains four exons. The α-subunit comes to expression in a variety of cells such as thyrotropes, gonadotropes, and syncytiotrophoblasts. The expression in gonadotropes and thyrotropes is in part differently regulated. In the developing pituitary αGSU is one of the first hormones to be detected and regulated by the transcription factors Pitx1, Lhx3, SF-1,Otx1 (Brown and McNeilly, 1999; Cohen and Radovick, 2002; Jorgensen et al., 2004), and FOXL2 (Ellsworth et al., 2006). In thyrotropes, however, expression of the TSHβ subunit precedes the expression of the αGSU. Apart from commonly used elements the promoter of the αGSU also contains response elements specifically used by gonadotropes, thyrotropes, or throphoblasts (Jorgensen et al., 2004). Also in the horse, this tissue-specific regulation of αGSU expression is documented (Farmerie et al., 1997). The canine αGSU has been coexpressed in a baculovirus expression system together with the canine TSHβ gene (Yang et al., 2000). The steroidogenic factor-1 (SF-1) has been shown in sheep to be essential for αGSU expression (Baratta et al., 2003). FIGURE 18-7 Sequence comparison of the-subunit for glycoprotein hormones (LH, FSH, and TSH). See the legend for Figure 18-6. b. (Pro)hormone Translation of the mRNA results in the formation of a precursor peptide with a molecular weight of approximately 13,500. The α-subunit has a high degree of homology among species (Fig. 18-7). The human sequence contains 92 amino acids, rather than the 96 as found in all other species studied so far. The shorter human sequence is due to a deletion of 12 nucleotides at the beginning of exon 3. After cleavage of the signal peptide, five disulfide bridges are formed. Two asparagine residues are prone to N-glycosylation, but there is also a putative O-glycosylation site (Fig. 18-7). The α-subunit is produced in excess of the β-subunit that determines the hormone specificity. The β-subunit formation is rate limiting in the formation of hetero αβ dimer. The thyrotropic cells of the anterior pituitary produce thyroid-stimulating hormone (TSH), which stimulates both the synthesis and secretion of thyroid hormone. a. Gene Expression The rat, human, and canine TSH gene consists of three exons, whereas the mouse gene has five exons. Its expression is restricted to pituitary thyrotropes. For the development of thyrotropes, and also gonadotropes, the same transcription factors are necessary as described for αGSU expressing cells. For the development of specific thyrotropes, the transcription factor Pit-1 is essential (Dasen and Rosenfeld, 2001). Expression of the TSHβ gene is stimulated by the transcription factors Pit-1 and GATA2, whereas TSHβ gene expression is suppressed by the active thyroid hormone T (Nakano et al., 2004; Shupnik, 2000). In contrast with the suppression of TSHβ synthesis by T, which is produced within the pituitary by selective deiodination of T by type 2 deiodinase (D2), TRH plays a dominant role in the stimulation of TSH synthesis (Nikrodhanond et al., 2006). The feline TSH has also been cloned and brought to expression (Rayalam et al., 2006). b. (Pro)hormone The TSHβ chain consists of 118 amino acids and forms six intrachain disulfide bonds (Fig. 18-8). There are no free cysteine residues, consistent with the fact that beta subunits form a heterodimer with the αGSU noncovalently. The TSHβ subunit has an N-glycosylation site at asparagine 23 and has a molecular weight of 18,000 after appropriate glycosylation. The α-subunit is produced in excess of the β-subunit, which determines the hormone specificity, and its formation is rate limiting in the formation of the α–β dimer. c. Secretion The release of TSH is mainly regulated by stimulation by the hypothalamic thyrotropin-releasing hormone (TRH) and a strong negative feedback by thyroid hormone (Pazos-Moura et al., 2003) at the pituitary and hypothalamic level. The prohormone thyroxine (T4) must therefore be converted locally to the active 3,3′,5-triiodothyronine (T3) by type 2 iodothyronine deiodinase (D2) before binding to the nuclear thyroid hormone receptor of the thyrotrope (Christoffolete et al., 2006). Superimposed on this regulatory system, hypothalamic factors (somatostatin, dopamine) may inhibit TSH synthesis and release. Intrapituitary growth factors can also stimulate (EGF) or inhibit (Neuromedin B) TSH release (Pazos-Moura et al., 2003). Evidence has been presented on an ultra-short-loop feedback control by TSH through pituitary folliculostellate cells (Prummel et al., 2004). FIGURE 18-8 Sequence comparison of the β-subunit of TSH. See the legend for Figure 18-6. In the dog, TRH stimulates plasma TSH concentrations. Single administration of TRH results in slightly higher plasma TSH concentrations than measured after combined stimulation with four releasing hormones (Meij et al., 1996b). In euthyroid dogs, cTSH release is relatively stable with hardly any pulses as measured during a 6-h pulse study, whereas in hypothyroid dogs, an increased basal concentration coincides with higher pulse frequencies (Kooistra et al., 2000b). d. Action TSH stimulates both synthesis and secretion of thyroid hormones from the thyroid gland. After receptor binding, TSH stimulates via the Gs alpha protein the production of cAMP, which acts as an intracellular second messenger. In addition, intracellular Ca2+ may modulate the biological effect of TSH via the phosphoinositol pathway. As a result of receptor activation T4, and to a much lesser extent T3, is secreted into the blood. Prolonged stimulation of the thyroid with TSH results not only in hypersecretion of thyroid hormone but also in enlargement of the thyroid gland. Genetic analysis of DNA from hyperplastic or adenomatous thyroid nodules in cats has revealed that somatic mutations in the TSH-receptor or in the Gs alpha protein are the cause of feline thyroid hyperplasia (Peeters et al., 2002; Watson et al., 2005). Apart from the thyroid, TSH receptors have been found in brain and pituitary where they may have a function in feedback mechanisms, and in lymphocytes, thymus, testis, kidney, adipose tissue, and bone (Davies et al., 2005). e. Disease/Tests Since 1995, sensitive TSH assays have become available for the dog (Williams et al., 1996). Since then numerous reports have been published on the usefulness of cTSH measurements in the diagnosis of canine hypothyroidism. Low total plasma T4 concentrations may be caused by decreased binding to plasma TBG—such as seen after long-term glucocorticoid excess in Cushing’s syndrome or interference of T4 binding to TBG by, for instance, NSAIDs or antiepileptic drugs—and they are therefore not conclusive for the diagnosis of hypothyroidism. Unfortunately some 25% to 40% of dogs with proven hypothyroidism will have plasma cTSH concentrations within the reference range for healthy dogs (Boretti and Reusch, 2004; Peterson et al., 1997). In dogs with nonthyroidal disease also low serum concentrations of total T4 are seen with serum TSH concentrations that remain within the reference range (Kantrowitz et al., 2001). In a recent study, quantitative measurement of thyroidal 99 mTcO4– showed no overlap between dogs with primary hypothyroidism and nonthyroidal illness (Diaz Espineira et al., 2006) and may together with a thyroid biopsy be the ultimate proof of primary hypothyroidism. Secondary hypothyroidism caused by a pituitary tumor (Rijnberk, 1971) or panhypopituitarism caused by a suprasellar tumor (Eigenmann et al., 1983b) is rare. German shepherd dogs with dwarfism have a secondary hypothyroidism, which can be demonstrated by a blunted TSH response on TRH in a combined pituitary function test (Kooistra et al., 2000c). For further details, refer to Chapter 20 on thyroid function. Chemical induction of hypothyroidism in horses using the antithyroid drug PTU results in a steady increase of plasma TSH concentration and an exaggerated response to TRH administration (Breuhaus, 2002). FIGURE 18-9 Sequence comparison of the β-subunit of LH. See the legend for Figure 18-6. Luteinizing hormone (LH), which is identical to the interstitial-cell-stimulating hormone, and follicle-stimulating hormone (FSH) are produced by the gonadotropic cells of the AL. a. Gene Expression In the gonadotropes αGSU appears to be the lead protein before the expression of the β-subunits, which determine the hormone specificity (Pope et al., 2006). Next the β-subunits arise in the bihormonal gonadotropes. During development of the pituitary, transcription factors Pitx, Hesx1, Lhx3, SF-1, and Otx1 regulate gonadotropin subunit expression (Brown and McNeilly, 1999). Although LH and FSH are produced within the same gonadotrope, its gene expression and secretion pattern are different. In primates, multiple copies of LHβ-related genes have been identified, the chorionic gonadotropins (CG), which are expressed in the placenta for the maintenance of early pregnancy. Apart from primates, only in equids has this placental production of CG been well described (Jorgensen et al., 2004). In the horse, placental eCGβ is derived from the same gene that encodes pituitary LHβ (Saneyoshi et al., 2001). For pituitary LHβ expression, three highly conserved response elements have been found in the promoter region that bind the early growth response protein (Egr-1), the orphan nuclear factor SF-1, and the homeodomain protein Pitx1 (Jorgensen et al., 2004; Melamed et al., 2006). For the regulation of basal FSHβ gene expression Lhx3 plays a prominent role, whereas a direct effect of SF-1 on FSHβ expression is not demonstrated in the ovine pituitary gland (Baratta et al., 2003). The LHβ promoter is sensitive to stimulation by GnRH via Egr-1 and inhibition by androgens. The activated androgen receptor (AR) binds SF-1 to block communication via Pitx1 (Jorgensen et al., 2004). The AR, however, increases FSHβ gene promoter activity, as also documented for the progesterone receptor (PR) and glucocorticoid receptor (GR) (Thackray et al., 2006). Estrogens activate the LHβ promoter (Melamed et al., 2006). Inhibin, activin, and follistatin, originally found as hormones from the gonads but they may also be produced within the pituitary, are important regulators of LH and FSH synthesis and release. Activin stimulates FSHβ gene expression and mRNA stability, whereas inhibin and follistatin inhibit FSHβ gene expression and accelerate the degradation of FSHβ mRNA (Gregory and Kaiser, 2004). As activin stimulates and inhibin inhibits expression of GnRH receptors, an indirect effect on LHβ expression and release also can be found. b. (Pro)hormone After cleavage of the signal peptide, LHβ proteins are formed of 121 amino acids (Fig. 18-9). Exception is the horse sequence, which shows a highly variant C-terminal part that is also some 14 to 28 amino acids longer. The β-subunit contains six intrachain disulfide bridges. In humans, LHβ has one unique N-linked glycosylation at asparagine 30. In the horse, asparagine 13 is glycosylated and in addition 12 O-glycosylation sites have been found (Bousfield et al., 2001). The percentage glycosylation is related to increased plasma half-life and thus bioavailability of the hormones. The FSHβ chain consists of 111 amino acids, six intrachain disulfide bridges, and two N-linked glycosylation sites at asparagine 7 and 24. The N-linked oligosaccharide chains are critical for bioactivity. Deglycosylated FSH may act as a potent FSH antagonist (Fares, 2006). The sequence of canine FSH shows the lowest homology (approximately 80%) with the sequences of other species (Fig. 18-10), which may cause limitations to the use of heterologous immunoassays for proper FSH measurements in the dog. FIGURE 18-10 Sequence comparison of the β-subunit of FSH. See the legend for Figure 18-6. c Secretion As with other pituitary hormones, the gonadotropins are released in a pulsatile fashion, stimulated by pulses of the hypothalamic gonadotropin-releasing hormone GnRH. The release of GnRH in seasonal breeders is tightly regulated by the length of the photoperiod and the subsequent release of melatonin by the pineal gland (Thiery et al., 2002). Pulsatile GnRH secretion has been demonstrated in sheep (Clarke and Cummins, 1982) and the horse (Irvine and Alexander, 1994). GnRH can be used for estrus induction and prevention in the dog (Gobello, 2007). In contrast to single administration of GnRH, continuous administration of GnRH agonists reversibly suppresses gonadotropin secretion because of down-regulation/desensitization of GnRH receptors. Effect of dose and duration of treatment with a GnRH-agonist resulting in a switch from stimulated LH and FSH release toward down-regulation has been reported recently for the dog (Concannon et al., 2006). In the dog, LH and FSH pulses coincide during different phases of the estrus cycle although the longer duration of FSH pulses may be due to the difference in plasma half-life. During progression from early to late anestrus basal plasma FSH increase without a concomitant increase in basal LH concentrations (Kooistra and Okkens, 2001a; Okkens and Kooistra, 2006). During the early follicular phase, the LH secretion has frequent increases of short duration (Kooistra et al., 1999). The preovulatory FSH in female dogs may start a few hours earlier or may coincide with the LH surge, which is associated with the highest plasma estradiol-17β concentrations and the start of increasing plasma progesterone concentrations (de Gier et al., 2006). During the mid- to late-luteal phase, basal LH concentrations in pregnant bitches were higher with lower peak frequency and height in comparison to nonpregnant bitches. Plasma FSH concentrations were higher during pregnancy (Onclin et al., 2002). Chronic administration of the synthetic progestin MPA has no effect on plasma LH concentrations, but, like in pregnancy, increased plasma FSH concentrations are found (Beijerink et al., 2006). Administration of dopamine-agonists to shorten the anestrus interval results in a rapid rise in basal FSH concentration (Okkens and Kooistra, 2006), whereas the serotonin antagonist metergoline, which lowers also plasma prolactin concentrations, does not affect the LH and FSH secretion patterns in the dog (Beijerink et al., 2004). Low plasma estrogen concentrations inhibit the GnRH and LH release through a negative feedback system, whereas high concentrations of estrogens may exert a positive feedback by stimulating GnRH release. Kiss1 neurons in the periventricular nucleus are thought to be involved in the estrogen and progesterone-induced LH surge (Smith et al., 2006). In the male, LH stimulates the synthesis and release of testosterone in the Leydig cells of the testis, which may in turn exert a negative feedback on LH secretion. This feedback may depend on aromatization of testosterone to estradiol in the brain. In the male dog, both testosterone and estradiol are the major inhibitors of LH and FSH release (Winter et al., 1982). Finally, FSH can also be regulated by more specific stimulation through activin or by prevention of activin binding and thus inhibition of downstream signaling by inhibin and follistatin (Gregory and Kaiser, 2004; Gregory et al., 2005). Other members of the TGFβ family such as the bone morphogenic protein-6, BMP-7, and BMP-15 can also exert an effect on FSH secretion. d. Action FSH stimulates in the female ovary the folliculogenesis and ripening of the ovaries to the antral follicle stage. LH stimulates changes in the ovarian follicle resulting in ovulation and maintenance of the corpus luteum (Hunter et al., 2004). LH stimulates the production of the androgen precursor androstenedione that is converted, by FSH stimulation, to estradiol-17β and during the luteal phase progesterone secretion. The LH effects are mediated by LHR containing granulosa cells through the release of EGF-like growth factors (Conti et al., 2006). FSH is essential for Sertoli cell proliferation and maintenance of sperm quality in the male testis. LH interacts predominantly with Leydig cells and stimulates the production of testosterone. e. Disease/Tests LH and the LHR are also involved in the pathogenesis of adrenal tumors in ferrets, which develop after castration-induced high plasma LH concentrations (Schoemaker et al., 2002b). In the adrenal carcinomas that secrete predominantly adrenal androgens, the presence of LHR mRNA and protein has been demonstrated. The LHR is expressed constitutively in urogenital tissues and adrenal glands in rodents, but they need functional maturation at a post translational level (Apaja et al., 2005). Dogs have also LHR and FSHR in the lower urinary tract (Ponglowhapan et al., 2006). In long-term spayed bitches, relative lower plasma LH and FSH concentrations were found in dogs with urinary incontinence in comparison with continent dogs (Reichler et al., 2005). Growth hormone (GH) and prolactin (PRL) together with the placental lactogen show homology in amino acid composition and some biological activities. Therefore, they may group together as a family of somatolactotropic hormones. There is increasing evidence that these hormones evolved from a single ancestral gene (Seo, 1985; Wallis, 1984). The somatotropic cells, producing GH, are the most abundant cells of the anterior lobe. a. Gene Expression In primates, multiplication of the GH gene has resulted in a cluster of five GH-related genes. The GH-N gene is expressed in the pituitary and the other four, consisting of the chorionic somatomammotropins (so-called placental lactogens) and a variant gene (GH-V), are expressed in the placenta during pregnancy. In most nonprimates, a single gene encodes GH, but a family of PRL-like genes (also including placental lactogens) is present. In sheep and goats in some individuals, there are two GH-like genes from which one may be expressed in the placenta. In red deer, two gene sequences encoding GH were found, but this is shown to be related to allelic polymorphism (Wallis et al., 2006). The GH-producing cells arise from a lineage of TSH-, GH-, and PRL-producing pituitary cells by the action of transcription factor Pit-1 that is under the control of the prophet of Pit-1 (Prop1). The final maturation of GH-producing cells is under the control of transcription factors Pitx1, Zn-15, Lhx3, and Pit1 (Mullis, 2005). Pit1 and GH expression are already found at the 8- to 16-cell stage in bovine embryos (Joudrey et al., 2003). For the activation of the pituitary GH gene, a 5′-remote locus control region in humans 14.5 kb upstream of the GH-N promoter plays an important role (Ho et al., 2006). This site contains also three Pit1 binding sites (Shewchuk et al., 2002). The pituitary somatotroph differentiation is further stimulated by glucocorticoids and thyroid hormone (Porter, 2005). GH mRNA expression is stimulated by GHRH and ghrelin by inducing cAMP production and subsequently Pit1 mRNA expression (Garcia et al., 2001; Theill and Karin, 1993; Yan et al., 2004). Somatostatin (SS) inhibits in general GH release although in porcine somatotropes SS can also stimulate GH release depending on the dose used. It appeared that SS receptors SST1 and SST2 mediate the inhibitory effects whereas SST5 mediates a stimulatory effect of somatostatin (Luque et al., 2006). The rat GH promoter contains a positive thyroid hormone response element (TRE) and a negative TRE (nTRE) (Sanchez-Pacheco and Aranda, 2003). GH expression is stimulated by the positive TRE. In the absence of T binding the unliganded thyroid hormone receptor (TR) may induce GH expression in nonpituitary cells. In severely hypothyroid dogs elevated plasma GH concentrations have been found, which may be related to this mechanism (Lee et al., 2001). Gonadal steroids modulate also GH production. 17β-Estradiol may reduce the inhibitory effect of SS on GH production, whereas testosterone treatment increases GH mRNA concentrations in adulthood (Chowen et al., 2004). b. Extrapituitary GH Expression In dogs, endogenous progesterone and exogenous progestins may induce considerable rises in plasma GH concentrations, resulting in acromegalic changes and insulin resistance with the possibility of development of frank diabetes mellitus (Eigenmann et al., 1983a; Selman et al., 1994a). GH excess has only been found to occur in intact female dogs during the progesterone-dominated phase of the sexual cycle or in dogs treated with progestins (Eigenmann and Rijnberk, 1981). Selman et al., showed the autonomous character of progestin-induced GH secretion (Selman et al., 1991) and found the canine mammary gland to be the source of plasma GH after progestin treatment (Selman et al., 1994b). The mammary origin was confirmed by an arterial-venous gradient across the mammary gland, a rapid decrease and normalization of plasma GH concentrations after complete mammectomy (Selman et al., 1994b), and the presence of GH mRNA in the canine mammary gland as measured by RT-PCR (Mol et al., 1995b). From the 100% sequence identity it is concluded that a single gene encodes pituitary and mammary GH in the dog, in agreement with the fact that only one canine GH gene is found in the published canine genome sequence. The dog is not unique in expressing GH in the mammary gland as it is demonstrated also in cats with progestin-induced fibroadenomatous changes of the mammary gland (Mol et al., 1995b), and GH mRNA has been demonstrated in the normal and neoplastic human mammary gland as well (Mol et al., 1995a; Mol et al., 1996). The presence of a putative binding site for the progesterone receptor in the GH promoter of human, rat, mouse, and dog makes it likely that progestin-induced GH expression is a direct effect of activated PRs on the GH gene promoter (Lantinga-van Leeuwen et al., 2002). FIGURE 18-11 Sequence comparison of GH. See the legend for Figure 18-6. Apart from the mammary gland, extrapituitary GH expression seems to be widespread throughout the body. GH functions already as an early local embryonic growth, and differentiation factor, including the neural tube, before pituitary GH expression is detectable (Sanders and Harvey, 2004). Furthermore, GH expression is found in the postnatal rat lung (Beyea et al., 2005), chicken testis (Harvey et al., 2004) and canine lymphomas (Lantinga van Leeuwen et al., 2000), insulinomas (Robben et al., 2002), and growth plate or osteosarcomas (Kirpensteijn et al., 2002). c. (Pro)hormone GH is a single-chain polypeptide. It contains two intrachain disulfide bridges and has an apparent molecular weight of 22,000. In humans, a small fraction of circulating GH has a molecular weight of 20 kDa because of alternative splicing of the pituitary GH gene. The metabolic effects are comparable to the normal 22 kDa form (Hayakawa et al., 2004). The amino acid sequence of GH belongs to the best-known sequences of pituitary hormones among species (Fig. 18-11). The sequences of canine and porcine GH are identical and very similar to the horse. The canine and porcine sequence is suggested to be identical to the GH sequence for the ancestral placental mammal (Forsyth and Wallis, 2002). In cattle, a polymorphism in the GH gene exists, resulting in four GH variants that can arise from two possible N-terminal amino acids (phenylalanine or alanine) and two amino acids in position 127 (leucine or valine). The transmission of the trait of high milk production was greater for homozygous leucine-127 in Holstein cows and valine-127 in Jersey cows (Lucy et al., 1993). GH exhibits also heterogeneity because of posttranscriptional processing that may vary in binding to the plasma GH binding protein (GHBP) or biological effects (De Palo et al., 2006). The glycine in the third α-helix of bovine GH (G118) and human GH (G120) is crucial for biological functioning of GH. Substitution of this glycine results in a molecule without growth-promoting activity that inhibits the actions of GH in vitro and in vivo (Kopchick, 2003). d. Secretion The release of GH by the pituitary is regulated by a variety of factors (Fig. 18-12 and Table 18-5). The integration of all these factors results in a pulsatile release of GH. In the female dog, plasma GH pulse patterns are dependent on the estrus cycle. In the early luteal phase, elevated basal GH concentrations are associated with a low pulse frequency of 2 peaks/12 h, whereas at anestrus lower basal GH concentrations are found with an enhanced pulse frequency of 5 peaks/12 h (Kooistra et al., 2000a). The higher basal concentration and diminished pulsatility in early luteal phase may be caused by a direct or indirect inhibition of pituitary GH release by GH secretion from the mammary glands stimulated by progesterone. FIGURE 18-12 Regulation of pituitary GH release, peripheral actions, and feedback. Pituitary somatotropes are not only stimulated by the GH-releasing hormone (GHRH), which was first isolated from human pancreas islet tumors in 1982 and next found in the hypothalamus, but also by the more recently discovered GH-releasing hormone ghrelin, which was originally isolated from rat and human gut. In the pituitary, two GHRH-R isoforms exist generated by alternative splicing. The predominant short form activates somatotropes through the adenylate cyclase/cAMP/PKA pathway. The pituitary contains also two splice variants of a distinct seven-transmembrane helix, G protein coupled receptor for ghrelin, called the GH secretagogue receptor (GHS-R). Only the long type 1a isoform activates pituitary somatotropes via the phospholipase C/IP/PKC pathway. The expression of both receptors is down-regulated by exposure to GHRH as well as ghrelin in porcine pituitary cell cultures (Luque et al., 2004). The release of GH is generally inhibited by soma-tostatin (SS). In the pituitary all five SS receptors (SST1-5) are expressed. In porcine pituitary cells low SS concentrations may, however, also stimulate GH release. It has been found that the inhibitory effects are mediated by SST1 and SST2 receptors, whereas SST5 mediates the stimulatory effect of SS on GH release in vitro (Luque et al., 2006). In young beagle dogs, 13 to 17 months of age, ghrelin was shown to a more potent GH secretagogue in comparison to GHRH. With aging, 7 to 12 year of age, the GH response to ghrelin was significantly lower, whereas only a moderate decrease in the sensitivity toward GHRH was noticed and GHRH appeared to be at higher age a more potent stimulator of GH release (Bhatti et al., 2006b). In contrast to humans, in the dog ghrelin has hardly any stimulatory effect on ACTH and prolactin release (Bhatti et al., 2006b; van der Lely et al., 2004). Plasma concentrations of acylated ghrelin, which is the biologically active form, are higher after fasting and lower after food intake, suggesting a role in feeding control and energy homeostasis also in the dog (Bhatti et al., 2006c, 2006d). No clear relation was found of plasma GH and ghrelin in the study by Bhatti et al., in agreement with a previous study in which also no close relation was found between plasma GH and ghrelin concentrations during the juvenile period in dogs (Yokoyama et al., 2005).
I. HYPOTHALAMUS-PITUITARY SYSTEM
A. Anatomical Considerations
1. Hypothalamus
2. Neurotransmitter Systems
3. Vascular System
, anterior lobe;
, intermediate lobe;
, neural lobe. Redrawn from Batten and Ingleton (1987).
4. Pituitary Organogenesis and Ontogenesis
5. Cells of the Anterior Lobe (AL)
6. Cells of the Intermediate Lobe (IL)
7. Cells of the Neural Lobe (NL)
B. Regulation of Pituitary Functions
1. Hypophysiotropic Hormones
2. Regulation of Gene Expression
3. Prohormone Biosynthesis, Processing, and Release
II. ANTERIOR LOBE AND INTERMEDIATE LOBE
A. Proopiomelanocortin-Derived Peptides
1. ACTH/α-MSH
2. β-Endorphin/β-Lipotropin
B. Glycoprotein Hormones
1. α-Subunit
2. TSH
3. LH and FSH
C. Somatomammotropic Hormones
1. GH
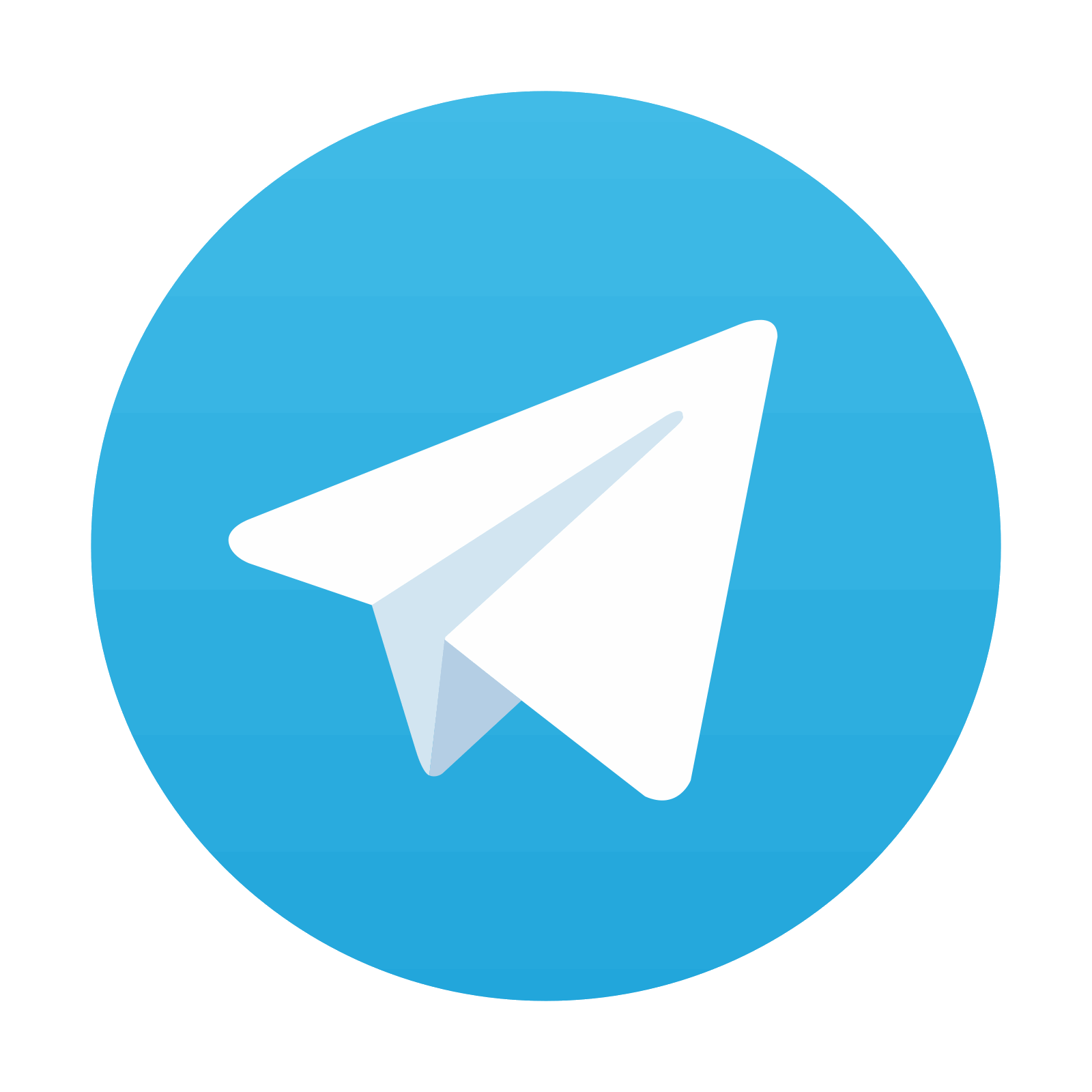
Stay updated, free articles. Join our Telegram channel
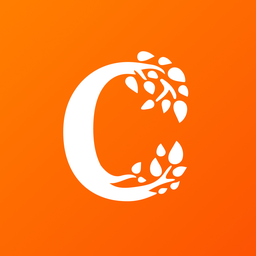
Full access? Get Clinical Tree
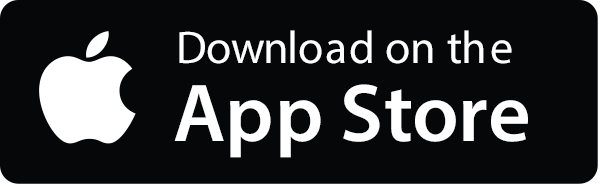
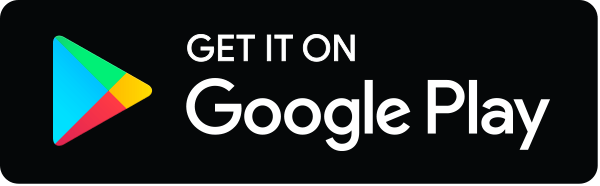