CHAPTER 4 Starches are broken down in the small intestine by pancreatic amylase to maltose. Disaccharides such as maltose, sucrose, and lactose are further digested by the brush-border enzymes maltase, sucrase, and lactase, respectively, into simple sugars. Maltase activity is high in the horse and is found along all regions of the small intestine. Sucrase activity has been shown to be highest in the proximal small intestine, whereas lactase activity is similar throughout the small intestine (Dyer et al., 2002). The monosaccharides glucose, fructose, and galactose are absorbed and transported in blood to tissues for energy metabolism or storage. The glucose and galactose transporter, SLGT-1 (sodium/glucose cotransporter 1), is expressed principally in the proximal small intestine and has a limited capacity for these sugars (Dyer et al., 2002). Therefore, at high levels of grain intake, sugar digestion and absorption may exceed the capacity of the small intestine, and these carbohydrates may pass to the large intestine (Dyer et al., 2002; Potter et al., 1992). This occurrence may contribute to digestive disturbances associated with high grain intake (Dyer et al., 2002). Dietary triglycerides are emulsified by bile salts from the liver and then broken down by lipases from the pancreas. The resulting fatty acids and glycerol are absorbed and transported to sites of storage and metabolism. Although equine diets are relatively low in fat, horses appear to be able to digest most fats efficiently. Digestibility of fat included in the diet is close to 100% at ranges between 15% to 23% dry matter (Kronfeld et al., 2004). This is likely supported by relatively high concentrations of lipase within the pancreas (Lorenzo-Figueras et al., 2007). Some dietary protein may also escape digestion within the small intestine, particularly when associated with cell wall fiber. Therefore, dietary protein in high-fiber feeds may not be available until the large intestine. This becomes important with respect to digestibility of feedstuffs to ensure adequate amino acids are available for absorption in the small intestine. High-quality protein sources will, therefore, be both highly digestible and have optimal amino acid profiles. Absorption of intact amino acids from the equine large intestine is likely small (Bochroder et al., 1994), though the presence of lysine transporters within the colon have been reported (Woodward et al., 2003). Whereas small intestinal digestion results in the absorption of protein as amino acids, ammonia is an important product of the digestion of protein in the large intestine. The absorbed ammonia can be used to synthesize nonessential amino acids in horses, or it can be excreted as urea in the urine. Along with the digestion and absorption of energetic substrates, the absorption of vitamins, minerals, and water necessary for athletic function is a vital function of the digestive tract. Water is absorbed from the large intestine, along with a large portion of the electrolytes (Argenzio et al., 1974a). The large intestine may act as a reservoir for fluid, particularly during dehydration, and is influenced by the amount of soluble fiber in the diet (Warren et al., 1999a). Sodium is absorbed along with glucose and water by SLGT-1, giving justification for the inclusion of glucose with electrolyte-replenishing beverages and supplements (Shirazi-Beechey et al., 2008). Further, salt water offered following a 45-km ride has been shown to assist with recovery of lost body weight (Butudom et al., 2002). Careful attention should be paid not only to the amount of mineral in the diet but also to the ratios of particular minerals (e.g., calcium:phophorus [Ca:P] and zinc:copper [Zn:Cu]) and their sources (organic versus inorganic; presence of interfering substances). Most minerals, including calcium and magnesium, are absorbed within the small intestine, although phosphorus is absorbed within the large intestine. Calcium absorption largely depends on calcium status in the body, and its absorption is regulated by parathyroid hormone and vitamin D. Calcium absorption can be further affected by phosphorus, phytate, and oxalates in the diet. Phosphorus absorption is also affected by the presence of high levels of calcium and oxalates in the diet. Much of the phosphorus in typical feeds is found as phytate phosphorus, which may be less digestible than nonphytate phosphorus. Fat-soluble vitamins are absorbed in the small intestine and may be influenced by the degree of fat absorption (Kronfeld et al., 2004). A considerable amount of B vitamin synthesis occurs in the large intestine (Linerode, 1966), and absorption from the large intestine has been demonstrated for several B vitamins. At rest: Following a meal, the process of digestion and absorption will result in an increase in the circulating levels of several nutrients. The effects of diet and feeding schedule on glucose metabolism have received the most attention from researchers because of the relationship between glucose and insulin. When a horse receives a meal consisting of a concentrate feed (such as corn), blood glucose levels will rise from a prefeeding concentration of approximately 4 to 5.5 millimoles per liter (mmol/L) to a concentration of 6.5 to 7.5 mmol/L within 2 hours of feeding. The extent of the increase in blood glucose concentration will depend on meal size and composition. In human nutrition, the glycemic index (GI) has been used to characterize the extent of the increase in blood glucose that occurs in response to specific foods. The GI is the area under the glucose curve after consumption of a test food expressed as a percentage of the response to a standard amount of a standard food. An alternative term, the glycemic load (GL), accounts for the available carbohydrate in the meal. There has been some interest in applying the GI or GL to equine diets (Kronfeld et al., 2004), but the relevance of a GI or GL to equine athletic performance has not been well defined. In addition, application of the GI or GL to equine diets is difficult, in part due to the inconsistency of the reference feed, differences in consumption rates, and potential interactions among ingredients. For example, a study showed that when fat was added to a grain mix, the GI for the grain mix tended to decrease (Pagan et al., 2000). Other studies have shown that processing can affect the glucose response to a specific feed (Healey et al., 1995; Hoekstra et al., 1999), but in some other studies, processing had no effect on glucose or insulin responses to feeding (Vervuert et al., 2003;2004). However, when a high-roughage meal is consumed, the increase in blood glucose is much smaller than when a grain meal is consumed (Stull and Rodiek, 1988). In humans, it is well recognized that absorbed triglycerides are packaged into chylomicrons for transport to sites of metabolism or storage. The enzyme lipoprotein lipase facilitates the hydrolysis of triglycerides to fatty acids, thus allowing movement into the cell. Once inside the cell, fatty acids may be used to resynthesize triglycerides. The postabsorptive fate of fat in equines is not well understood, as attempts to isolate chylomicrons following a fat load have failed (Watson et al., 1992). Also, it is interesting to note that horses have a relatively slow clearance rate of triglycerides following a load (Moser et al., 1993). This slow clearance occurs despite an apparent increase in lipoprotein lipase activity with increased substrate, such as with hyperlipemia in ponies (Watson et al., 1992). Conversely, it appears that chronic fat feeding affects the metabolism of fat such that flux of triglycerides from blood to muscle is increased (Geelen et al., 1999), likely through the activity of lipoprotein lipase (Geelen et al., 2001). Following a meal, plasma concentrations of amino acids also will increase. Peak levels of most amino acids in the plasma have been shown to occur within 2 hours of feeding, with decreases seen by 4 hours. Most amino acids will return to baseline concentrations within 8 hours (Hackl et al., 2006). It has been suggested that even after a 10-hour period of withholding feed, plasma amino acid concentrations are highly variable, so care should be taken with interpretation of data (Hackl et al., 2006). Similar to glucose, insulin stimulates amino acid uptake by skeletal muscle and other tissues. Unlike triglycerides, the VFA are taken directly into the bloodstream and delivered to tissues without being packaged into chylomicrons or other lipoproteins. VFAs are absorbed from the large intestine quickly, within 30 minutes of being infused directly into the cecum (Glinsky et al., 1976). Lieb (1971) also detected VFAs in the portal blood 30 minutes after infusion into the cecum. However, as peak production rates occur 2 to 8 hours after feeding (Argenzio et al., 1974b), one would expect blood concentrations to peak shortly thereafter. The VFAs are transported in the portal system to the liver, where butyrate and propionate are taken up (Lieb, 1971). Acetate appears to remain in blood for delivery to systemic tissues. Propionate is largely glucogenic, and it has been reported that between 50% and 61% of blood glucose is derived from colonic propionate, depending on diet (Simmons and Ford, 1991). Butyrate is converted to acetate and ultimately acetyl-CoA (coenzyme A), which can then be oxidized via Kreb’s cycle or used for fat synthesis. Acetate is quantitatively the most important VFA. On an all-roughage ration, the molar percentage of VFAs in cecal or colon fluid is about 70% acetate, 17% propionate, 8% butyrate, and 5% others (isobutyrate, isovalerate, and valerate) (Glinsky et al., 1976; Hintz et al., 1971). When horses are fed high-concentrate diets, the proportion of acetate decreases, and the proportion of propionate increases. As expected, dietary composition affects blood VFAs accordingly; Pethick and coworkers (1993) and Doreau et al. (1992) reported higher concentrations of blood acetate with higher-forage diets. During the process of fermentation, some energy is lost and is unavailable to the horse. Nonetheless, fiber fermentation and the VFAs produced can generate significant energy for the horse. Using labeled VFAs, it was estimated that 30% of the horse’s total digestible energy intake was derived from cecal VFA production when fed a high-forage diet (Glinsky et al., 1976). Assuming substantial VFA production within the colon as well, it is assumed that an even greater proportion of the horse’s energy needs would be met by VFAs. Studying arterial-venous differences, Pethick and others (1993) found that acetate accounted for up to 32% of the total substrate oxidation within the hindlimb at rest. Following the decline of blood glucose concentrations, insulin secretion from the pancreas is decreased. In the hours following a meal, low blood glucose concentrations stimulate the release of glucagon, another hormone from the pancreas. Glucagon functions to antagonize the effects of insulin; as insulin promotes the storage of energetic substrates, glucagon acts to mobilize energetic substrates. In the horse, feed deprivation of 54 hours results in significantly elevated glucagon concentrations (Sticker et al., 1996). At the liver, through activation of cyclic adenosine monophosphate (cAMP) and protein kinase A (PKA), glucagon ultimately functions to activate glycogen phosphorylase. This enzyme stimulates glycogenolysis, and glucose is released into the bloodstream. Glucagon also activates hormone-sensitive lipase within adipocytes, promoting the hydrolysis and subsequent release of free fatty acids (FFAs) for mobilization and metabolism. Frank et al. (2002) reported a 16-fold increase in plasma FFA concentration following feed restriction for 36 hours. Prolonged fasting in humans results in the adaptation to utilize ketones, which are fatty acid metabolites, for energy production, especially within the central nervous system. In horses, extreme feed deprivation often results in hyperlipemia, characterized by accumulation of very-low-density lipoproteins (VLDL) and plasma triglyceride concentrations >500 milligram per deciliter (mg/dL), though there is significant variation among horses (Frank et al., 2002). The response to prolonged fasting suggests that lipid metabolism in horses may not be identical to lipid metabolism in humans. It is possible that hormone-sensitive lipase activity in horses is impaired (Watson et al., 1998), particularly if accompanied by insulin resistance (Jeffcott and Field, 1985). Further research will be necessary before fat metabolism in the equine athlete is fully understood. The contribution of various energetic substrates to metabolism at rest appears to largely depend on the diet; however, overall resting metabolism will be primarily supported through aerobic pathways. Recently, glucose tracers and modeling computations were used to compare glucose metabolism in horses. Horses consumed either a diet with the majority of calories coming from starch and sugar or a diet with most calories coming from fat and fiber (Treiber at al., 2008). It was concluded that increased availability of glucose in the starch and sugar type diets resulted in an increased flux of glucose, suggesting increased usage. Several other methods are available to determine substrate contribution to overall energy production. Indirect calorimetry is a method of estimating energy expenditure from the measurement of respiratory gases. The respiratory exchange ratio (RER) is the ratio of carbon dioxide exhaled to the oxygen inhaled, and this closely matches the respiratory quotient (RQ), which refers to the ratio of cellular production of carbon dioxide to oxygen consumption. The RER and the RQ are approximately equal under steady state conditions. On the basis of the chemical structure of carbohydrate and fat, oxidation of each substrate affects the RER such that oxidation of carbohydrate results in an RER of 1.0 and oxidation of fat results in an RER closer to 0.7. The effect of diet on the RQ or the RER has not been well studied in resting horses, but it would seem that there would be greater shift toward carbohydrate metabolism (and, therefore, an RQ closer to 1.0) if horses were fed higher-starch and higher-sugar types of diets. During exercise: With exercise, energetic substrates are mobilized and used to generate adenosine triphosphate (ATP) for skeletal muscle. However, the specific substrates mobilized and utilized for energy production depend on several factors, including the intensity of the exercise, duration of the exercise, fitness of the individual, habitual diet, and feeding state. The main substrates of energy production are carbohydrate and fat. Sources of carbohydrate include blood glucose (from a recent meal or glycogenolysis or gluconeogenesis in the liver) and muscle glycogen. Muscle contraction mediates the movement of GLUT4 to the muscle membrane (independent of insulin) to facilitate glucose uptake into muscle during exercise. Fat sources include FFAs hydrolyzed from adipose tissue, blood triglycerides, or muscle triglyceride stores. Protein can be utilized for energy production, though its overall contribution to energy production is minimal except during endurance types of exercise. In the horse, it is possible that VFAs contribute to energy production during exercise. Pethick et al. (1993) showed that acetate contributes significantly to energy production at the muscle at rest, and Pratt et al. (2005) showed greater clearance of acetate during low-intensity exercise compared with rest, suggesting it was used for energy production. Exercise intensity has the greatest effect on substrate use. In general, as the intensity of the exercise increases, there is a shift toward a greater percentage of energy being derived from carbohydrate sources and less energy derived from fat. This change in major energetic substrate is often referred to as the crossover concept. It should be noted, however, that at all times, there is combined metabolism of fat, carbohydrate, and even protein, just at different contributions. At rest and during low-intensity type of exercise, a majority of the ATP produced will be through fat oxidation. As the intensity of the exercise increases through the 30% to 50% In the horse, the oxidative substrates for energy production also largely depend on exercise intensity. For example, Geor et al. (2000b) showed that muscle glycogen (and lactate) accounted for approximately 30% of the total energy expenditure at 30% of Substrate selection at a given exercise intensity is influenced by hormones, blood flow, and local factors such as the energy status of the cell. The onset of exercise is met with an increase in epinephrine concentration. Epinephrine acts at the adipose tissue and hormone-sensitive lipase to mobilize triglycerides into FFAs and glycerol. Thus, at the start of exercise, particularly low- to moderate-intensity exercise, a rise in FFAs is observed. It has been shown that the contribution of FFAs to energy production during low-intensity exercise is largely dependent on supply. For example, a rise in fatty acid concentrations from the administration of heparin increases fat oxidation and reduces glycogen use during moderate exercise (70% As exercise intensity increases, blood flow is shunted away from adipose tissue and directed more at muscle, thereby decreasing the delivery of FFAs from adipose tissue to muscle. Because of reduced substrate supply, energy status of the cell decreases (that is, ATP is reduced, and AMP and inorganic phosphate are increased). Increases in AMP stimulate one of the two key enzymes regulating carbohydrate metabolism, glycogen phosphorylase (Howlett et al., 1998). The other key enzyme, pyruvate dehydrogenase (PDH), is also upregulated with increasing exercise intensity, largely due to contraction mediated increases in calcium (Howlett et al., 1998). Increases in carbohydrate flux decrease fat oxidation, likely through the limitation of fat transport into the mitochondria. The transport of fat is mediated by carnitine palmotoyl transferase (CPT-1), an enzyme inhibited by malonyl-CoA, which is increased with increased carbohydrate flux (Coyle et al., 1997). Furthermore, if fatty acid availability is increased pharmacologically, such as via infusion of a fat emulsion (Intralipid), fat oxidation by muscle is still hindered at higher intensities (above 65% Thus, it appears that in contrast to the Randle Effect, at higher intensities of exercise, carbohydrate metabolism regulates fat oxidation (Coyle et al., 1997). In the horse, the infusion of glucose (and consequential increase in carbohydrate flux) prior to exercise at 55% As indicated above, although both fat and carbohydrate are utilized in different amounts, depending on exercise intensity and duration, at any given time, there will be a combination of these substrates generating ATP. However, because of the relatively large energetic storage supply of fat (primarily triglycerides stored in adipose), carbohydrate availability, in particular muscle glycogen, is thought to be limiting to exercise. During high-intensity exercise, glycogen will be the primary energetic substrate. However, because the duration of such exercise is relatively short, overall glycogen use is minimal. Studies in Thoroughbreds (in 800-m to 2000-m exercise) and Standardbreds (in 1600-m exercise) have found glycogen depletion rates around 20% to 35% (Harris et al., 1997; Lindholm et al., 1974). During lower-intensity endurance exercise (e.g., 80-km to 160-km rides), there is substantial glycogen depletion (between 50% and 75%), despite a lower rate of use (Snow et al., 1981; Snow et al., 1982). Adequate stores of muscle glycogen are important for both short-term, high-intensity exercise as well as for endurance (low intensity) exercise. It has been shown that reduced glycogen stores (to approximately 30% of resting values) can negatively affect subsequent exercise bouts and impair performance (Lacombe et al., 2001; Lacombe et al., 2003). In humans (Coyle and Coggan, 1984) as well as in horses (Snow et al., 1981), fatigue following endurance exercise (>80-km ride in horses) is associated with a depletion of both liver and muscle glycogen stores. Hypoglycemia is often reported following such exercise (Essen-Gustavsson et al., 1984), where hepatic glucose production cannot meet the increased demands of exercise. It is possible that when the muscle glycogen concentration is reduced, blood glucose uptake into muscle is increased to accommodate metabolism, thereby reducing the amount of glucose available to the brain (Hargreaves, 1997). It is believed that endurance exercise that results in hypoglycemia causes a decline in central nervous system function, or “central fatigue” (Davis et al., 1992; Nybo, 2003) and that maintenance of euglycemia through endogenous supplementation can attenuate fatigue (Davis et al., 1992; Farris et al., 1998). Not only is glucose directly required for adequate cerebral function, but increases in fatty acid concentration with exercise promote the production of serotonin in the brain, which contributes to fatigue (Farris et al., 1998). Hypoglycemia may also play a role in peripheral fatigue, particularly when muscle glycogen is depleted, as carbohydrate intermediates are required to maintain flux through the citric acid cycle. Administration of exogenous glucose is therefore also believed to provide substrate for energy production while preserving muscle glycogen (Coyle and Coggan, 1984; Geor et al., 2000b). In the horse, it is possible that propionate (derived from microbial fermentation in the hindgut) serves as anaplerotic substrate (Kasumov et al., 2007), whereas acetate and butyrate are easily metabolized to acetyl-CoA (Knowles, 1974), though only acetate has been studied as a source of energy for the horse (Pethick et al., 1993; Pratt et al., 2005; Waller et al., 2009a). Therefore, for equine sports such as endurance racing, horses should be fed periodically during the event to maintain adequate substrate levels, through direct absorption of glucose as well as VFA production from microbial fermentation (Harris, 2009). It has been shown that in both humans and horses, exercise conditioning that results in improved fitness causes a move toward reduced glucose flux and increased fat oxidation at a given intensity (Geor et al., 2002). It is likely that increases in oxidative enzyme capacity and reduced catecholamine response to exercise favor this shift in substrate use (Korzeniewski and Zoladz, 2003). Similarly, adaptation to a high-fat diet increases fat oxidation while reducing dependency on carbohydrate metabolism (Pagan et al., 2002; Sloet van Oldruitenborgh-Oosterbaan et al., 2002). The consumption of a diet that provided 29% of the digestible energy as fat reduced carbohydrate utilization during a low-intensity exercise test (at 35% During recovery from exercise: Following exercise, the body works to replenish substrates used during the exercise bout, particularly muscle glycogen. Rapid resynthesis of glycogen is important for the horse, especially for events such as 3-day eventing, where strenuous exercise bouts are performed on consecutive days. Glycogen is formed via the actions of the enzyme glycogen synthase (GS). GS is regulated both allostearically and covalently (Nielsen and Richter, 2003). Depending on substrate availability, namely, glucose-6-phosphate, glycogen synthase is activated. GS is also highly regulated by insulin. With exercise, insulin concentrations decrease, facilitating the mobilization (rather than storage) of energetic substrates. However, because of increased blood flow, it is likely that a significant amount of insulin actually reaches the muscle. In humans and rodents, insulin sensitivity is high following exercise, promoting glycogen synthesis. Furthermore, contraction-mediated increases in glucose uptake (insulin independent) remain high after exercise, providing substrate for glycogen synthesis. GS activity also appears to be affected by the amount of glycogen present such that following exercise, when muscle glycogen concentrations are low, glycogen synthase activity is increased. It is believed that the enzyme may reside within granules of glycogen and is freed upon glycogen usage (Nielsen et al., 2001). Due to increased muscle contraction–stimulated glucose uptake, increased delivery of insulin, and reduced glycogen concentrations, GS is active following exercise. The degree of GS activation is largely dependent on the intensity of the exercise and the extent of glycogen depletion. Studies in humans (Nielsen and Richter, 2003) and horses (Pratt et al., 2007) have found inverse correlations between GS activity and glycogen concentrations. In humans, the replenishment of glycogen following exercise is rapid and depends largely on glucose supply to muscle. It is recommended that athletes consume carbohydrate (1 gram per kilogram body weight [g/kgBW]) immediately after glycogen depleting exercise and then every 2 hours for a total of 6 hours to maximize glycogen resynthesis (Ivy, 1998). With this program, glycogen concentrations are replenished within 4 to 6 hours, and supraphysiologic concentrations of glycogen can be achieved. In horses, however, glycogen replenishment following exercise is much slower. It has been shown that oral administration of carbohydrate (3 g/kgBW over 6 hours) following glycogen-depleting exercise results in only 56% of pre-exercise glycogen concentrations after 6 hours (Geor et al., 2006). However, the venous infusion of glucose (at 0.5 g/kgBW) for 6 hours (for a total of 3 g/kgBW) following glycogen-depleting exercise resulted in more rapid glycogen resynthesis, with glycogen concentrations returning to approximately 75% of baseline by 6 hours (Geor et al., 2006). Despite intravenous (IV) glucose, supraphysiologic concentrations of glycogen are not attained. Similarly, starch consumption (2.8 kg of corn) following glycogen-depleting exercise resulted in muscle glycogen of only 52% of resting concentrations at 24 hours following exercise (Jose-Cunilleras et al., 2006). Interestingly, it has been shown that electrolyte supplementation along with hay and grain feeding results in greater glycogen replenishment than does feeding alone (Waller et al., 2009b). Therefore, it is likely that factors beyond substrate availability influence glycogen resynthesis in the horse. In fact, the provision of acetate following glycogen-depleting exercise, which can be oxidized directly, may preserve any glucose derived from the diet for use in glycogen resynthesis (Waller et al., 2009a). The differences in glycogen metabolism between horses and other mammals (i.e., humans and rodents) may be attributed to differences in glycogen content and insulin sensitivity. For example, muscle glycogen stores in horses range from 500 to 650 mmol glucosyl units per kilogram (units/kg), whereas in humans stores range between 320 and 400 mmol glucosyl units/kg. Further, glycogen itself may be different between species, with proglycogen and macroglycogen being repleted at different rates (Brujer et al., 2006). As indicated above, an increase in insulin sensitivity is observed in most species following glycogen-depleting exercise, which is believed to hasten glycogen resynthesis when carbohydrate is consumed (Holloszy, 2005). In horses, however, no such increase in insulin sensitivity exists following glycogen-depleting exercise to approximately 60% of original stores (Pratt et al., 2007). In fact, insulin sensitivity was lower when assessed 30 minutes following exercise compared with baseline insulin sensitivity (no prior exercise). Furthermore, although GS activity was increased following exercise, it was not to the same extent as observed in other species (Pratt et al., 2007). It is possible that these discrepancies contribute to the relatively slow glycogen resynthesis following exercise that is observed in horses. It should be noted that patients with McArdle’s disease, a disease characterized by high glycogen concentrations in humans, have low insulin sensitivity and GS activity (Nielsen et al., 2002). With high-intensity exercise, blood lactate concentrations in horses can rise dramatically, to levels higher than those seen in other species. Lactic acid is produced during anaerobic metabolism in muscle, and it is transported out of muscle into blood in an attempt to maintain muscle pH. Red blood cells (RBCs) act as a sink for lactate, facilitating its transport out of muscle. The key transporter regulating lactate uptake into RBCs is the monocarboxylate transporter (MCT1). Following exercise, lactate is primarily delivered to the liver, where it can be converted back to glucose via gluconeogenesis or can be oxidized (Brooks et al., 1995). Plasma glucose concentrations also increase with high-intensity exercise. This is, in part, caused by glycogenolysis at the liver and return to baseline within 60 minutes (Gordon et al., 2007). Mobilization of fatty acids from adipose tissue continues to increase following exercise, as evidenced by the increase in glycerol concentrations (Poso et al., 1989). Fatty acids are either oxidized or may be re-esterified into triglycerides (Poso et al., 1989). Low-intensity endurance-type exercise results in substantially lower lactate concentrations because of reduced dependence on anaerobic metabolism to support energy production. Furthermore, blood glucose concentrations tend to not peak, in part because of reduced liver glycogen concentrations to support glycogenolysis (Lindholm et al., 1974). In fact, if no carbohydrate is consumed during or following endurance exercise, the animal may become hypoglycemic (Coyle and Coggan, 1984). Fatty acids will be used for oxidation, whereas glycerol will be an important component of gluconeogenesis. Muscle protein catabolism may also support gluconeogenesis, and increased alanine concentrations have been observed following endurance exercise in the horse (Trottier et al., 2002). Exercise type, climate, and individual horse characteristics will affect the nutrient requirements of each equine athlete. It is impossible to define the requirements of every type of equine athlete, so broad categories of exercise levels are often used. The National Research Council (NRC, 2007) has suggested that mature horses may be grouped into four exercise categories: (1) light, (2) moderate, (3) heavy, and (4) very heavy. Light exercise is described as 1 to 3 hours of imposed exercise each week that consists of mostly walking and trotting. Many horses used for recreational purposes would be included in this category. Moderate exercise is described as 3 to 5 hours of imposed exercise each week that consists of mostly trotting with some cantering and skills work. Horses used for riding lessons, horse shows, ranch work, and possibly polo would fit into this category. This category could also include horses that are beginning training programs for racing or other strenuous events. Horses that compete at the upper levels of strenuous sports such as 3-day eventing, endurance racing, polo, flat racing, steeplechasing, or harness racing would be included in the heavy and very heavy exercise categories. These horses may be exercised for long periods each week or for relatively short periods at a high intensity. In addition, many of these horses are transported frequently or over long distances and are often housed in unfamiliar environments, all factors which may contribute to increased use of some nutrients. The suggested nutrient requirements (NRC, 2007) for a 550-kg horse at each exercise level are shown in Table 4-1. These values should be used as a starting place for individual horses, rather than as absolutes. The following material discusses some of the sources of variation for each nutrient category. TABLE 4–1 Recommended Daily Nutrient Intakes of 550-kg Mature Horses Performing Light, Moderate, Heavy, and Very Heavy Work* *Adapted from NRC (2007). Sodium, potassium, and chlorine recommendations apply to mild climates. In cool or hot climates, sodium, potassium, and chlorine amounts should be decreased or increased, respectively. Energy: Dietary energy requirements can be expressed in a variety of ways. Gross energy is the total amount of energy in a feed. Digestible energy (DE) is determined as the difference between the gross energy that is consumed and the energy excreted in feces. Thus, DE approximates the amount of energy absorbed by the horse. In the United States and several other countries, the dietary energy requirements of horses are commonly expressed in units (either megacalories or megajoules) of DE. However, daily energy requirements may also be expressed in units of net energy. A system that utilizes net energy as the basis of requirements has been developed in France, but it expresses feeding guidelines in relation to the amount of net energy contained in a standard feed (Martin-Rosset et al., 1994; Martin-Rosset and Vermorel, 2004). The French system more accurately accounts for the efficiency of use of different energy sources, but it has not been completely validated for exercising horses, and many horse owners are unfamiliar with it. Therefore, energy requirements in this chapter will be expressed as units of DE, unless otherwise noted. The daily energy requirement of an equine athlete is the sum of the calories needed to maintain the horse at rest and the calories needed to replace the energy used during exercise. The caloric needs for maintenance are variable. The NRC (2007) described three levels of maintenance energy requirements for adult horses: (1) minimum, (2) average, and (3) elevated. The elevated maintenance requirement applies to horses with alert temperaments and above-average levels of voluntary activity, which would include many elite performance horses. Other factors that can affect maintenance energy requirements include climate, housing conditions, and transportation. The caloric debt created by exercise will depend on several factors including the duration of the exercise and the intensity of the exercise. Exercise intensity is often equated with speed, and many studies have shown that energy utilization increases with speed for exercising horses (Eaton et al., 1994; Eaton et al., 1995; Hiraga et al., 1995 Pagan and Hintz, 1986). However, the effect of speed on energy use does not appear to be linear; and horse-related factors such as fitness and gait will affect the relationship (Eaton et al., 1999; Hoyt and Taylor, 1981; Katz et al., 2000). Other factors such as hills, jumps, or ground conditions can also increase energy use at a given speed. The weight of the rider must also be considered. Thus, although speed of travel is an important characteristic of work effort, it may not adequately describe the intensity of a work bout unless other factors are considered as well. In most of the studies cited above, oxygen utilization was measured as a means of estimating energy use, but it is difficult to measure oxygen utilization under most practical conditions. Heart rate (HR) is closely related to oxygen utilization during submaximal exercise (Coenen, 2005; Eaton et al., 1995), and it is relatively easy to monitor heart rate in practical situations. Therefore, HR may provide a relatively simple but comprehensive assessment of exercise intensity (Coenen, 2005; NRC, 2007). The NRC (2007) reported that Coenen had reviewed data from 87 studies to arrive at the following equation relating HR to oxygen utilization in exercising horses: This equation can be used to estimate the liters of oxygen utilized during an exercise bout. When combined with an estimate of the caloric equivalent for each liter of oxygen (approximately 4.86 kcal/L; NRC 2007) the equation can be used to calculate the calories burned during the exercise bout. For example, if a 490-kg horse with a 60-kg rider (combined BW of 550 kg) is exercised at an HR of 90 beats per minute (beats/min) for 30 minutes, it will consume approximately 350 L of oxygen. If each liter of oxygen has an energetic equivalent of 4.86 kilocalories (kcal), then energy use during that exercise bout will be about 1.7 megacalories (Mcal). To arrive at the amount of DE that must be consumed to replace this energy, the efficiency of DE use for exercise must be known. Pagan and Hintz (1986) estimated that the efficiency of DE use for exercise was about 57%. However, a summary of subsequent studies suggests that the efficiency is lower, especially for high-intensity exercise (NRC, 2007). If the efficiency of DE use is 40% for the type of exercise described above, then about 4 Mcal of DE must be added to the daily diet to replace the energy expended in the exercise described above. Few horses perform exactly the same amount of exercise every day, but it is not practical to change the diet every day. Feeding programs can be based on weekly (or even monthly) work averages, especially if the horse is in a steady state of fitness. The NRC (2007) recommendations for DE intakes in exercising horses are based on weekly averages. These broad categories of light, moderate, heavy, and very heavy exercise represent increased DE intakes that are 20%, 40%, 60%, and 90% above maintenance, respectively. The maintenance requirement for horses in light, moderate, and heavy work has been estimated at 33.3 kcal/kgBW/day; however, the maintenance requirement for horses in very heavy work has been estimated at 36.3 kcal/kgBW/day. The higher maintenance requirement was applied to horses in very heavy work because it is anticipated that they will have more voluntary activity, higher lean body mass and higher feed intakes; all factors that can increase the maintenance component. The weekly work loads suggested by the NRC (2007) for each exercise category will not exactly match the work loads of specific performance horses, so it should be expected that actual feeding programs will have to be adjusted to reflect the real work loads on an individual basis. It is relatively easy to evaluate whether a performance horse is receiving an appropriate amount of energy from its diet. If daily energy expenditure exceeds energy intake, then the horse will lose weight. Conversely, if energy intake exceeds energy expenditure, then the horse will gain weight. Skilled horsemen can often detect a small change in a horse’s body weight, but the most reliable method of assessing weight change is to weigh the horse regularly. The most consistent results are obtained if the horse is always weighed at the same time of day relative to feeding and exercise. In human athletics, body composition profiles have been developed for different types of athletes. There is limited information about the ideal body composition for horses engaged in various activities, at least in part because it is difficult to measure body composition in live horses. Ultrasonic measurement of subcutaneous fat over the rump has been used by some researchers to assess body composition in equine athletes. It would be expected that equine athletes would have less body fat compared with sedentary horses. Using ultrasonic equipment to estimate rump fat, Lawrence and coworkers (1992b) reported that competitive endurance horses had a mean body fat of 7.9% (n = 61). For comparison, broodmares in a separate study were reported to have 10% to 15% body fat (Lawrence et al., 1992a). Kearns et al. (2002) reported that high-quality Standardbred race horses had a mean body fat of 7.4% for males (n = 6) and 9.9% for females (n = 8). However, the relationship between rump fat thickness and carcass fat is not particularly strong (Kane et al., 1987; Westervelt et al., 1976), and none of the available equations relating rump fat thickness to body composition were derived with athletic (fit) horses. Therefore, the estimates of total body fat that exist for horses may illustrate qualitative differences in fatness within groups of equine athletes but the specific estimates may not be quantitatively accurate. Body condition scoring has also been used to assess horses. Henneke and coworkers (1983) developed a condition scoring system for broodmares that has been applied to performance horses. This system uses a nine-point scale, where 1 is extremely thin and 9 is extremely fat. Endurance horses tend to have condition scores below 5 (Garlinghouse and Burrill, 1999; Lawrence et al., 1992a). Gallagher et al. (1992) reported a mean condition score of 5 for Thoroughbreds in race training. Similarly, horses used for competitive polo have condition scores of about 5, but horses used for dressage and as show hunters may have condition scores above 6 (Pagan et al., 2009). Phased dietary programs for humans that change energy supply and source with training status and event have been explored (Schroder et al., 2008). Phased feeding programs that manipulate calorie amount and type during training may hold some potential for equine athletes as well, but additional research is needed to define optimal programs for horses performing in different events. Regular monitoring of DE intakes is warranted for equine athletes as they progress through different stages of training and competition. More frequent monitoring should be considered for horses that are less than 4 years old, as these horses will be growing, training, and competing at the same time. As discussed previously, adequate muscle and liver glycogen stores are important for optimal performance. Horses are capable of synthesizing glucose from propionate that is produced by microbial fermentation, but glucose may be obtained more efficiently from dietary sugar and dietary starch. Most feeds are relatively low in simple sugars; therefore, starch is the primary source of absorbable glucose in horse feeds. The optimal level of dietary starch has not been determined. It is possible that the level of dietary starch that is optimal for an endurance horse is different from the amount that is optimal for a race horse. Starch is most effective as an energy source if it is digested in the small intestine and absorbed as glucose. Starch that escapes the small intestine will be fermented to VFA in the large intestine. The amount of ATP produced from absorbed glucose will be greater than the amount of ATP generated from the VFA produced from glucose that reaches the large intestine. Several factors affect the susceptibility of starch to small intestinal digestion, including the size of the meal, the type of starch, and the processing of the starch prior to ingestion. Oat starch is digested more easily than corn starch, and small meals are digested more easily than large meals. In addition, processing of cereal grains has been shown to increase the availability of starch to digestion in the small intestine. Although starch is an important energy source in the diets of most performance horses, dietary fiber and dietary fat are also important. Dietary fibers that are fermented to VFA provide energy, and as noted above, propionate can be used to synthesize glucose. Fats, particularly vegetable oils, are often added to the diets of performance horses. Fats contain more than twice as much DE as starch on an equivalent weight basis. Fats are often used to increase total calorie intake when feed intake is limited. In addition, horses that have been adapted to supplemental fat in the diet appear to have an increased capacity to utilize fat during exercise and to spare carbohydrate use (Dunnett et al., 2002; Pagan et al., 2002). Protein and amino acids: The importance of protein to athletic performance has been pondered for decades, possibly for centuries. Even ancient Greek athletes may have believed that the addition of meat to their diet would improve performance (Hickson and Wolinski, 1989). Today, it is recognized that dietary protein plays an important role in the maintenance of tissue but that it is a relatively minor energy source in comparison with carbohydrate or fat. Most amino acids in the body are found in structural proteins and are, therefore, not readily available for energy production. However, there are some free amino acids in plasma and some labile proteins in the liver that could provide amino acids to fuel energy production. In other species, evidence for exercise-induced protein catabolism comes from the observation of metabolic changes during exercise and from studies using isotope-labeled amino acids. Several studies with horses have documented that plasma alanine concentrations increase with moderate exercise (Essen-Gustavson et al., 1991; Miller-Graber et al., 1991a, b; Miller and Lawrence, 1988). Plasma urea concentrations may also increase in response to long-term exercise (Snow et al., 1982). No studies with labeled amino acids have been performed to examine whether certain amino acids are preferentially catabolized in exercising horses, but it can be inferred from studies with other species that leucine might be used to a greater extent by muscle compared with other essential amino acids. Regular exercise can alter the percentage of lean body mass in humans, and certain types of exercise can increase absolute lean body mass. An increase in absolute lean body mass in horses may be more likely with strength training than with endurance training, but hypertrophy of the longissimus dorsi has been reported in response to aerobic training in horses (D’Angelis et al., 2007). Regular exercise has been reported to enhance nitrogen retention in horses (Freeman et al., 1988; Wickens et al., 2003), which could be related to increased lean body mass. Performance horses will have increased nitrogen losses in sweat, and there may be increased endogenous fecal losses when feed intakes are high. Collectively, these observations support the concept that exercising horses have higher protein requirements compared with sedentary horses. There is general agreement that regular exercise can elevate the protein requirement of horses, but there has been much debate regarding the magnitude of the increase. In 1989, the NRC suggested that horses engaged in intense exercise (such as racing) should receive twice as much dietary crude protein (CP) as would a sedentary horse of the same body weight. This recommendation was arrived at by maintaining the same protein:calorie ratio in the diet of the intensely working horse that was suggested for the sedentary horse. This recommendation was a practical approach to meeting the protein needs of exercising horses, but it was not based on actual estimates of protein needs in exercising horses. In 2007, the NRC substantially reduced the dietary protein recommendations for exercising horses. For example, it is currently recommended that 550-kg horses performing light, moderate, heavy, and very heavy work receive 769 g CP/day, 845 g CP/day, 948 g CP/day, and 1105 g CP/day, respectively; compared with the previous recommendations (NRC, 1989) of 895 g CP/day, 1074 g CP/day, and 1432 g CP/day for light, moderate and intense work, respectively. The current recommendations apply to diets with highly digestible protein sources, whereas previous estimates were based on lower estimates of dietary crude protein digestibility. The 2007 NRC recommendations for crude protein intakes of exercising horses account for the crude protein needed for lean tissue accretion during exercise, the crude protein needed to replace nitrogen losses in sweat, and the crude protein needed for normal maintenance. The 2007 NRC recommendations do not maintain a constant protein:calorie ratio across all levels of work effort (light, moderate, heavy, and very heavy). The additional dietary protein needed for muscle gain was estimated as 0.089 g CP/kgBW, 0.177 g CP/kgBW, 0.266 g CP/kgBW, and 0.354 g CP/kgBW, for light, moderate, heavy, and very heavy exercise, respectively (NRC, 2007). The crude protein needed to replace nitrogen losses in sweat was calculated from estimates of the nitrogen losses in sweat with adjustments for the efficiency of digestible protein use and crude protein digestibility. Sweat losses were estimated at 0.25%, 0.5%, 1%, and 2% of BW per day for light, moderate, heavy, and very heavy exercise, respectively, and the nitrogen content of sweat was estimated as 1.25 g/kg sweat (NRC, 2007). The estimates used by the NRC (2007) to calculate the amount of dietary protein needed to support lean tissue accretion were based on limited experimental data. In addition, sweat losses can be extremely variable depending on environmental conditions. Therefore, the current recommendations probably do not apply to every horse in every situation. However, the current recommendations are consistent with studies that have examined the effect of exercise on nitrogen retention in horses (Freeman et al., 1988; Wickens et al., 2003). Diets providing 12% to 15% of the total energy as protein or 1.2 g to 1.7 g protein/kgBW have been suggested to be adequate for human athletes (ADA, 2000; Rennie and Tipton, 2000); the current NRC recommendations are consistent with these allowances. There are no demonstrated benefits to feeding very high levels of crude protein to exercising horses. Meyer (1987) suggested that digestible protein intakes above 2 g/kgBW per day should be avoided in endurance horses because of effects on water balance and urea and ammonia metabolism. If dietary protein is 79% digestible (NRC, 2007), Meyer’s recommendation would set an upper limit of about 2.5 g CP/kgBW. Exercising horses consuming approximately 3.2 g CP/kgBW per day excreted more urea in sweat and had higher plasma urea concentrations than horses consuming approximately 1.6 g CP/kgBW (Miller et al., 1991a). An increase in post-exercise orotic acid excretion in the horses receiving a high-protein diet was interpreted to suggest that the high-protein diet exceeded the capacity of the urea cycle. It has been suggested that high-protein diets are acidogenic and may affect systemic acid–base balance in exercising horses (Graham-Thiers et al.,1999; 2001). As a dietary energy source, protein is metabolized to net energy less efficiently than is starch or fat; thus, if protein is added to a diet at the expense of another calorie source, there could be a negative effect on net energy intake. If protein replaces starch in the diet of exercising horses, muscle glycogen storage may be reduced (Pagan et al., 1987), although this response has not been observed in all studies (Miller-Graber et al., 1991b). Although high-protein diets are not recommended, extremely-low-protein diets should also be avoided. Low-protein diets may not provide adequate amounts of essential amino acids. In addition, the microbial population in the equine large intestine will require adequate nitrogen for optimal function. In other species, the term “ideal protein” is used to describe a dietary protein with an array of amino acids that closely matches the amino acids required by the animal. It is presumed that horses require the same amino acids in the diet as other monogastrics, but few studies have investigated the amino acid requirements of athletic horses. A summary of available data suggested that a 500-kg horse doing moderate to heavy work needs approximately 34 g of lysine per day (NRC, 2007). The NRC (2007) crude protein requirements for athletic horses are based on a diet containing good-quality protein containing at least 4.3% lysine (as a percentage of the crude protein). If a lower-quality protein is fed, more total protein will be needed to ensure that the lysine requirement is met. Lysine is usually considered the first limiting amino acid, so if lysine needs are met, it is likely that other amino acid requirements will be met as well. However, experimental evidence that verifies this assumption is not available. The incorporation of various amino acids in equine supplements is common. A review of studies evaluating the ergogenic benefits of these supplements can be found later in this chapter. Although not generally considered an ergogenic amino acid, glutamine is an important amino acid for enterocyte and leukocyte metabolism. There is some evidence that glutamine supplementation may help maintain gut health in challenged animals (Sukhotnik et al., 2007; Wu 2009), but studies in horses have not been conducted. Exercise or training may decrease plasma glutamine concentrations in humans, and a decrease in glutamine availability to leukocytes had been suggested as a mechanism for exercise-induced immune suppression. Muscle and plasma glutamine concentrations do not appear to change in response to acute exercise in horses (Beaunoyer et al., 1991; Miller-Graber et al., 1990; Russell et al., 1986), but studies in heavily trained horses have not been conducted. Aerobic capacity is closely related to lean body mass; therefore, the maintenance of lean body tissue is probably important for optimal athletic performance in horses. Diets that do not meet the amino acid needs of the horse may lead to loss of lean body mass; therefore, it is important to provide an adequate amount of high-quality protein in the diets of performance horses. Some human athletes consume high-protein or amino acid supplements, in an attempt to stimulate muscle synthesis or to reduce the effects of exercise-induced muscle damage. It appears that adequate protein intake will help preserve lean body mass in exercising individuals that consume energy-deficient diets (Pikosky et al., 2008). Inadequate feed consumption during rigorous training can result in negative energy balance and weight loss. If consumption of a higher-protein diet during this time would help preserve lean body mass and favor fat loss, then the effects of negative energy balance on the horse might be partially ameliorated. Unfortunately, there have been no studies with horses that test this hypothesis. Studies with human athletes have suggested that the timing of ingestion of a protein or amino acid supplement may affect whether a beneficial effect is observed. Consuming protein during resistance-type exercise has been reported to enhance protein synthesis in humans (Beelen et al., 2008). Consuming protein or amino acid supplements shortly after exercise has been reported to upregulate markers of protein synthesis (Dreyer et al., 2008; Willoughby et al., 2007). Recent literature reviews suggest that muscle soreness and indicators of exercise-induced muscle damage in humans may be reduced when protein or amino acid supplements are consumed before, during, or after exercise, although the results are not always consistent (Howatson and van Someren, 2008; Negro et al., 2008). The effects of administering protein or amino acid supplements to horses in the period immediately before or after exercise may deserve study. It is possible that studies performed in the past did not provide protein or amino acid supplements at the most optimal time.
Nutrition of the performance horse
Equine digestion, absorption, and metabolism
Nutrient metabolism
O2max range, there is an increase in the absolute oxidation of fat, with maximum fat oxidation peaking at approximately 55% to 65%
O2max (maximal oxygen consumption) in humans (Achten et al., 2002). As exercise intensity reaches 65%
O2max and higher, the relative contribution of fat to overall oxidation declines. Carbohydrate oxidation is relatively low (30%–40% of total energy expenditure) during lower-intensity exercise and increases to nearly 100% of energy expenditure with very-high-intensity exercise. Work by Romijn et al. (1993) reported RER values of 0.73, 0.83, and 0.91 at exercise intensities of 25%, 65%, and 85%
O2max, respectively, in humans. Also, using tracers, this group was able to determine the contribution of plasma glucose, plasma FFAs, muscle glycogen, and muscle triglyceride. At the lower intensities of exercise, plasma FFAs likely make up a significant portion of the relative energy expenditure, whereas at the higher intensity of exercise, muscle glycogen is the primary source of energy (Figure 4-1).
O2max, and about 65% of total energy expenditure at 60% of
O2max. As expected, at lower intensities of work, fat oxidation accounts for a larger percentage of relative energy expenditure (∼55%) compared with that at higher intensity (fat oxidation accounting for 25% of energy expenditure). At both exercise intensities, blood glucose contributed 12% of the relative energy expenditure at 30% of
O2max and 7% of energy expenditure at 60%
O2max. It should be noted that there may be differences in substrate utilization among different breeds (Prince et al., 2002) and that other factors such as fitness, age, and gender can contribute to individual differences among horses.
O2max; Costill et al., 1977). The concept that fat supply and metabolism regulates carbohydrate metabolism is termed “the Randle Effect” (Randle et al., 1964). The Randle Effect states that glucose oxidation is impaired in the presence of fatty acids. Specifically, increases in fatty acids were shown to increase acetyl-CoA and citrate, which inhibits pyruvate dehydrogenase (PDH), phosphofructokinase (PFK), and hexokinase activities, ultimately decreasing glucose metabolism (Randle et al., 1964). Thus, at lower intensities of exercise, fat supply to muscle is high, so fat oxidation will be greater than carbohydrate metabolism. It should be noted that in the effort for fat to be oxidized, carbohydrate intermediates are required to maintain the citric acid cycle (“fat burns in a carbohydrate flame”). Pyruvate is a major contributor to maintain the cycle, and amino acids (glutamine and aspartate) play a role in anaplerosis as well. Thus, even with substantial fat stores, carbohydrate availability can limit exercise at lower intensities.
O2max). It has been suggested that transport into mitochondria by CPT-1 is inhibited by lactate (Starritt et al., 2000), potentially contributing to the rationale behind the reduction in fat oxidation at higher intensities. At higher intensities, there will also be a further increase in catecholamine response. In addition to its effects on lipolysis, epinephrine is a significant stimulator of glycogen phosphorylase, the enzyme that stimulates glycogenolysis. Epinephrine affects glycogenolysis to a greater extent than it does lipolysis, contributing to the greater dependency on glycogen at higher exercise intensities.
O2max reduced fat oxidation, which supports this theory (Geor et al., 2000a). In summary, it is likely a combination of reduced fat availability and increased glycolytic flux that result in the increase in carbohydrate oxidation at higher intensities of exercise. Furthermore, with increasing exercise intensity, the recruitment of type II muscle fibers increases compared with the recruitment of type I fibers. Because type II fibers are more glycolytic, it stands to reason that there would be increased rates of glycogen and glucose use for energy production and a lower dependency on fat oxidation at higher intensities of exercise.
O2max) (Pagan et al., 2002). Fat adaptation also resulted in reduced lactate accumulation with a standardized stepwise exercise test (Sloet van Oldruitenborgh-Oosterbaan et al., 2002). Glucose kinetics were investigated in horses adapted to either a diet high in starch and sugar (SS) (SS: 3.3% fat dry matter [DM], 44.7% non-structural carbohydrate [NSC]) compared with a diet high in fat and fiber (FF) (FF: 10.6% fat DM,13.1% NSC DM)(Treiber et al., 2008). This study found increased glucose use during exercise for horses on the SS diet and subsequently lower glucose use during exercise for horses on the FF diet. It was suggested that dietary fat adaptation increases metabolic flexibility and could contribute to the ability to spare muscle glycogen.
Nutrient requirements of performance horses
Stay updated, free articles. Join our Telegram channel
Full access? Get Clinical Tree
Nutrition of the performance horse
Only gold members can continue reading. Log In or Register a > to continue
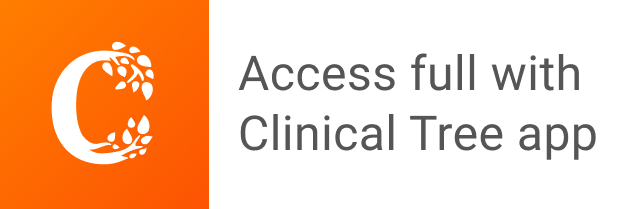