The horse’s skeletal musculature is highly physically adapted: in particular, and relative to other species, it has a greater muscle mass relative to body weight, large intramuscular stores of energy substrates (glycogen in particular), a high global mitochondrial volume, a high proportion of fast-twitch muscle fibers with shortening velocities greater than would be expected for an animal of this size. With all these adaptations, a horse’s locomotion is highly efficient.1,2 Some of these muscular adaptations likely reflect functional specialization following selective breeding of animals for elite endurance, sprint and jumping capacities.3 For example, in contrast to most mammals in which 30–40% of bodyweight consists of muscle, and in non-athletic horse breeds (∼42%), skeletal muscle comprises more than half (∼55%) of a mature Thoroughbred’s bodyweight.3 Total muscle blood flow in horses exercising at an intensity when O2 consumption is at a maximum ( Equine muscle is considerably heterogeneous, the diversity reflecting functional specialization and adaptive plasticity which has been studied extensively over the past 40 years. Muscle biopsy in particular has resulted in a greater understanding of the response of this tissue to exercise and training, and much of this work is summarized in previous reviews.5–7 This chapter focuses specifically on new data obtained in the past decade, while assessing earlier studies from a later perspective. Equine muscle physiology research has centered on use of percutaneous needle biopsy (Fig. 6.2), a technique originally described for the M. gluteus medius by Lindholm & Piehl.8 This is the heaviest muscle of the entire pelvic limb,9 it is very active during exercise10,11 and shows considerable adaptation to training.5–7 However, care must be exercised in interpreting data from a single biopsy from this muscle, particularly given its heterogeneity.12 Other locomotory muscles (e.g. semitendinosus, biceps femoris, longissimus lumborum, triceps brachii and cleidocephalicus) can also easily be biopsied using the same technique. Equine muscle biopsy samples for routine histologic are optimally shipped sealed in plastic containers and shipped on ice packs to be processed within 24 h and can thus be interpreted by the receiving laboratory with minimal artifact.13 Samples intended for more specialized analysis, such as genomic or metabolic studies, might require more particular storage and transport conditions. Muscle samples are useful for studies in vivo and in vitro using a range of morphologic, biochemical, physiologic, and molecular techniques. Muscle samples for histochemistry are frozen in isopentane pre-cooled in liquid nitrogen. Samples for biochemistry are immersed directly in liquid nitrogen. Samples for microarray expression are put in RNA stabilizer.14 In addition, a portion of the sample may be directly fixed for electron microscopy, thereby allowing analysis of ultrastructure and measurement of capillary and mitochondrial density.15 Biochemical and physiologic studies can also be made on dissected and skinned single fibers.16,17 Several recent studies in endurance horses have used a minimally traumatic muscle ‘microbiopsy’ technique, which allows collection of enough tissue to evaluate cellular functions in exercised muscle.18,19 Some heritable myopathies can also be diagnosed in horses with a similar minimally invasive method (see Chapter 7).14 Biochemical analysis of homogenized equine muscle samples has enabled the study of broad metabolic responses to exercise and metabolic adaptation to training (see reference5 for a review) through analysis of selected muscle enzyme activities, their substrates, and intermediary metabolites. However, homogenization of samples prevents the differentiation of the various individual fiber types or the study of important morphologic features such as fiber size, capillary density, and myonuclear location. Some of these disadvantages can be overcome by analyzing single fibers biochemically.20 Histochemical evaluation of muscle, combined with image analysis, has provided invaluable information about the contractile and metabolic properties of equine muscles, specifically regarding fiber types, oxidative and glycolytic capacities, fiber sizes, and capillary density. However, subjective visual assessment of qualitative histochemical reactions (Fig. 6.3A,B) has until recently limited the application of these methods.21,22 In recent years, more objective and quantitative histochemical methods have been applied to equine muscle.23 Cellular and molecular diversity within equine muscle has also been addressed via study of myofibrillar and non-contractile proteins by immunohistochemistry,23 gel electrophoresis,24 a combination of both techniques,25,26 enzyme-linked immunosorbent assay,27 and multiple immunofluorescence labeling.22 The past few years have seen the production of a considerable number of monoclonal antibodies that label contractile and non-contractile muscle isoproteins, some of which can be used effectively in immunohistochemical evaluation of horse muscle.28,29 The technique’s specificity provides, among other things, a more objective way to assess muscle fiber type (Fig. 6.3C).22,30 Electrophoretic methods for the quantification of myosin isoforms have also been validated in the horse (Fig. 6.3D),24 and immunoelectrophoresis has enabled the specific identification and relative quantitation of certain muscle-derived proteins.27 Molecular biology and genomic techniques have also been extensively applied to the field of equine muscle physiology.31–38 Northern blotting, reverse transcription followed by polymerase chain reaction, in situ hybridization, and direct sequencing of muscle-specific genes provide the means with which to study the molecular and genetic diversity of muscle proteins at the transcript (mRNA) and cDNA levels; microarray technology enables a more global approach. Furthermore, given that during the early phase of fiber type transformation, isoform-specific mRNA is detectable some time before a change in the specific protein,33 mRNA quantitation is useful for examining exercise and training effects on muscle composition and gene expression.33 Furthermore, the recent sequencing of the equine genome by an international consortium was a major advance that will impact equine genomics in the near future. With this rapid progress, new applications in early performance evaluation and the detection of disease markers become available.38 For example, expression profiling could be used to explore muscle metabolism changes related to exercise, training, pathology and illegal medication in horses.35 Several candidate athletic-performance genes have been identified in the racehorse as principal responsible for fatty acid oxidation, muscle glycogen degradation, increased insulin sensitivity, muscle strength, muscle speed, and transport of lactate across membrane.36,39–42 In addition to cellular and molecular techniques, non-invasive analyzes such as nuclear magnetic resonance and electromyography,43,44 are increasingly utilized to examine the effects of exercise and training on the neuromuscular system. Furthermore, combined electromyography, force plate, and gait analysis have applications for assessing muscle activation patterns during locomotion.10,11,45,46 Data can be examined in terms of whole muscle architecture (the arrangement of muscle fibers within the whole muscle relative to the axis of muscle force generation) when evaluating biomechanically distinct functions.47 Often attributes are described in terms of muscle belly and tendon length, muscle fascicle length, muscle fascicle pennation angle and physiological cross-sectional area.46,48–51 Most skeletal muscles are derived from paraxial mesodermal tissue following its condensation into segmentally-arranged somites. Cells of certain lineages become compartmentalized within each somite as it differentiates; the dorsolateral compartment (known as the myotome) contains two subsets of myogenic precursor cells: the cells of one subset are destined to become the axial musculature, whereas cells of the other subset migrate into the periphery to form the muscles of the body wall and the limbs.52 Myogenic precursor cells differentiate to form myoblasts and these fuse to become discrete populations of myotubes that subsequently fuse into myofibers,53 while α-motor neurons establish neuromuscular junctions. Embryonic myogenesis shares many similarities with the regeneration of mature myofibers following injury, a subject that is covered in detail elsewhere (see Chapter 7). The two primary requirements of locomotor muscle are to support the center of mass and to move the limbs. During steady-state locomotion, metabolic energy is primarily required to support the body weight against gravity. This role is most effectively fulfilled by muscles with short, pennnate fibers, and long tendons, such as those found in the limbs of horses.48,54 Yet locomotion in athletic horses is rarely steady-state: for example, gait is perturbed when accelerating from standing, running uphill or jumping over obstacles. During such activities, net muscle work must increase to raise either the kinetic or potential energy of the center of mass.9 Muscles that are useful for doing work on the system have long, parallel fibers and limited in-series elastic tissue and are, in most cursors, primarily found in the proximal limb.9,55 Thus, functional specialization of muscle architecture is seen in a wide range of animals in which proximal limb muscles are specialized for doing work and more distal limb muscles are specialized for economical generation of large forces.9 Horses and other quadrupeds reveal further functional specialization between the limbs. When compared with proximal thoracic limb muscles, proximal pelvic limb muscles of the horse are larger in volume and have shorter fascicles, indicating that from an evolutionary perspective, pelvic limb muscles have sacrificed the ability to exert force over a wide range of motion, in order to produce large forces.9,55,56 Muscle architecture has also been shown to vary between the same muscles in different breeds of horses. Thus, Quarter Horses inherently possess large strong pelvic limb muscles, with the potential to accelerate their body mass more rapidly than those of Arab horses47 which are optimized to function with maximum economy rather than maximum power output.51 Equine locomotor muscles are mainly located proximally on the appendicular skeleton with their tendon located distally, which has the effect of reducing the weight of the lower limb and decreasing the energy necessary to overcome inertia when the limb swings back and forth. Equine natural gaits typically consists of stretch-shortening cycle exercises, in which lengthening (eccentric) and shortening (concentric) actions of the muscle-tendon complex in the limbs are repeated during each cycle.45,57,58 During contractions, elastic energy is stored in tendons and intramuscular connective tissues during the lengthening (eccentric) phase of the cycle, and then reused and added to the active energy produced in the shortening (concentric) phase. Thus, the total force exerted across a muscle is the sum of active force generated by the contractile machinery and passive forces provided by elastic structures that are arranged either in parallel or in series with the contractile proteins in the muscle-tendon complex.45,46,59 Consequently, movements of the distal limb are mainly passive and result from the release of elastic energy of the digital flexor tendons and suspensory ligament when the limb is unloaded.57 However, while the equine interosseus muscle (suspensory ligament) has undergone a reduction of muscle content during its evolution, it still contains significant muscular components that likely contribute to limb stability and elastic storage of energy during locomotion.49 Thoracic limb protraction is largely a passive process in horses60 and thoracic limb protraction is likened to a catapult mechanism in which energy is stored relatively slowly in elastic tissues (the internal tendon of biceps brachii and lacertus fibrosus) during limb loading, but that is released rapidly when the toe leaves the ground, thereby rapidly protracting the limb. Furthermore, the equine biceps muscle-tendon unit acts as a tuneable spring and the contractile component functions to modulate the energy required to accelerate forelimb protraction at different speeds.60 Tendon springs are particularly important in a cursorial quadruped like the horse, as they facilitate the exchange of kinetic potential and elastic strain energy and reduce the amount of work that muscles must perform in order to move the animal’s limbs and center of mass. This effective utilization of elastic energy explains much of the admirable (superior) locomotor efficiency of horses, which consume rather less metabolic energy than would be expected based on the substantial energetic demands on this animal, particularly at fast gaits.58 Moreover, metabolically-economical force production can also be increased through eccentric muscle contraction, where muscle length increases while active (developing contractile force) due to-externally applied forces. In contrast, movements of the proximal limb result primarily from active muscular contraction and generally, most locomotor muscles are active during the propulsion stage of weight bearing in each limb.11,45,61 Muscle activity, as measured by electromyography, is positively correlated with running speed, with muscles within the same group having significant differences in their activities when a horse exercises at constant speed.11,61,62 Furthermore, different locomotor tasks, such as moving up or down gaits or changing speed, require adjustment of the amount of work that a muscle must perform to raise or lower, or to accelerate or decelerate the animal’s centre of mass.11,61,62 More than 90% of a muscle is made up of myofibers, with the rest consisting of nerves, blood vessels and the fat and connective tissue that separates the individual fibers (endomysium), the fascicles (perimysium), and the whole muscle (epimysium; see Fig. 6.1). The connective tissue merges with both the origin and the insertion tendons of the muscle, as well as with internal tendons in compartmentalized muscles. Blood vessels and nerves course within the perimysium. Capillary arrangement in the skeletal muscle is optimized for oxygen delivery to tissue during exercise; usually several capillaries are located within close to the muscle fiber proximity, sometimes circumferentially but more often running parallel to each fiber. Skeletal myofibers are typically elongated cells (generally believed to be between 30 and 100 mm in length) with tapered ends. Fibers vary from 10 to 100 µm in diameter and are multinucleated (Fig. 6.4A). Nuclei are normally located at the fiber’s periphery in a subsarcolemmal position. Although the cytoplasm contains other organelles found in many cell types, it is largely occupied by the contractile apparatus, which consists of contractile proteins and their supportive structures all grouped together as myofibrils. In longitudinal sections, individual muscle fibers have numerous cross-striations (dark and light bands), orientated perpendicularly to the fiber’s long axis (Fig. 6.4B). Lighter I bands alternate with darker A bands; within the I band there are dense striations called Z disks. The repeating unit between two adjacent Z disks is known as a sarcomere, the unit of muscular contraction. Each sarcomere includes half the I band on each side of the A band (Fig. 6.4C,D). I bands contain only thin filaments (8 nm diameter), whereas A bands contain both thin and thick (15 nm diameter) filaments. Within the A band, the H band is defined as the central area where the thick filaments do not overlap with thin filaments (Fig. 6.4D). The central darker portion of the H band is designated as the M line (see Fig. 6.4D). Transverse section of the sarcomere at the overlapping zone between thick and thin filaments reveals each thick myofilament surrounded by thin myofilaments arranged in a hexagonal lattice (Fig. 6.4E). Muscle contraction occurs when (within each sarcomere), thin myofilaments slide over the thick myofilaments, bringing the adjacent Z disks closer together. Hence, upon contraction, the I band shortens and the H band starts to disappear. Thick filaments contain myosin and other myosin-binding proteins. Sarcomeric myosins have two heads and a long tail and consist of two heavy chains and two pairs of light chains (Fig. 6.5A). The myosin head is the motor domain that contains the ATP-binding site, the actin-binding site and the myofibrillar ATPase enzyme. The major components of the thin filaments are tropomyosin, the troponin complex (consisting of three subunits: troponin C (TnC), troponin T (TnT) and troponin I (TnI)), and two helical filamentous strands of actin (F-actin), made up of polymerized globular actin monomers (G-actin) (Fig. 6.5B). Elongated tropomyosin dimers lie within the major groove of the actin filament, spanning seven actin monomers; each troponin complex is also associated with a seven-actin repeat. The elongated NH2-terminal of TnT extends for a considerable length from each tropomyosin molecule, spanning the gap between adjacent molecules. Mitochondria are located beneath the sarcolemma and between myofibrils (Fig. 6.6, Fig. 6.7). This intimate relationship means that ATP produced during oxidative phosphorylation is readily available for the contractile machinery. Intramuscular substrates, such as glycogen and lipids, are also stored between the myofilaments and under the sarcolemma (see Fig. 6.7). Numerous other proteins, including myoglobin, glycolytic enzymes, and various intermediate filaments, are distributed throughout the cytoplasm. The sarco(endo)plasmic reticulum (SR) of skeletal myofibers is an intracellular membranous system located between the myofibrils (see Fig. 6.6, Fig. 6.7), but which has no physical continuity with the external surface membrane (sarcolemma). Much of its tubular network is orientated longitudinally to the myofibrils. The SR contains, amongst other molecules, a large amount of a Ca2+-ATPase enzyme, the protein calsequestrin, and the calcium release channel (ryanodine receptor or RYR1). At the AI junction of the sarcomere, the SR tubules become confluent and form terminal cisternae orientated perpendicularly to the long axis of the cell (Fig. 6.7D). Two adjacent cisternae are separated by a structure known as the T-tubule, which is a long tubular invagination of the sarcolemma communicating directly with the extracellular space. Together the three structures make up a triad (see Fig. 6.6, Fig. 6.7). The motor end-plate (see Fig. 6.11B) is a specialized region on each fiber where the α-motor neuron interdigitates with the sarcolemma. The postsynaptic membrane of the motor end-plate contains numerous acetylcholine receptors. The remainder of the sarcolemma contains a variety of specific structural proteins, channels, pumps, and hormone receptors. Of note, myofibers have a cytoskeleton of various intermediate filament proteins that link the contractile apparatus with a complex of proteins at the sarcolemma, known as the dystrophin-associated complex (see Fig. 6.14), which itself provides a structural link with the extracellular matrix. Between the sarcolemma and the extracellular matrix is the basal lamina (Fig. 6.7E), which is generally closely apposed to the sarcolemma except where it leaves the sarcolemma to course over the surface of satellite cells (Fig. 6.8). Further information about satellite cells is available elsewhere (Chapter 7). A motor unit consists of an α-motor neuron and the skeletal muscle fibers that it innervates (Fig. 6.9). Since each time an α-motor neuron fires, the entire motor unit contracts, coarser movements are generated in those muscles that have many muscle fibers making up motor units (such as the locomotor muscles) compared with those with few (such as the extrinsic eye muscles). Muscle fibers within one motor unit are generally all of the same histochemical type, but they are normally widely distributed between fibers from other motor units and therefore give rise to the characteristic checkerboard pattern that is apparent with certain histochemical stains (see Fig. 6.3A). Loss of this checkerboard pattern is evident in denervated muscle (Fig. 6.10A,B) where there may be selective loss and atrophy of fibers of one histochemical type (Fig. 6.10A), or in reinnervated muscle following disease or injury, by patterns of fiber grouping (Fig. 6.10C).34,63 Large-diameter α-motor neurons innervate fast-twitch fibers, whereas smaller ones tend to innervate slow-twitch fibers. Proprioception is the term given to the mechanism underlying the self-regulation of posture and movement through stimuli originating in sensory receptors embedded in joints, tendons, muscles, and the labyrinth of the ear. In skeletal muscles and tendons these receptors are known as spindles and Golgi tendon organs and each type has been characterized in the horse.64 Signals derived within these sensory receptors are conveyed via a variety of well-defined reflex pathways that generate specific motor responses. Muscle spindles consist of specialized intrafusal muscle fibers surrounded by a connective tissue capsule and lie parallel to regular muscle fibers (Fig. 6.11A). Sensory nerves (type Ia and type II) terminate on the intrafusal fibers in specialized sensory endings and generate afferent signals that are relayed to the spinal cord via the dorsal horn. Type Ia nerves carry both dynamic (rate of stretch) and static (amount of stretch) afferent signals, whereas type II nerves sense only static muscle length. Motor innervation to the muscle spindle is provided by γ-motor neurons, which regulate the sensitivity of muscle spindles to muscle stretch. Golgi tendon organs are located within the connective tissues of tendons, joint capsules, and muscles. They lie in parallel with the direction of mechanical force that they measure and, from them, afferent type Ib fibers convey proprioceptive signals to the spinal cord. Acetylcholine released from pre-synaptic nerve terminals at neuromuscular junctions (end-plates; Fig. 6.11B) binds to acetylcholine receptors and increases the conductance of the post-junctional membrane to Na+ and K+. The inward movement of Na+ down its concentration gradient predominates, causing a transient depolarization (about 20 mV) in the end-plate, known as the end-plate potential. This depolarization is sufficient to activate sarcolemmal voltage-gated Na+ channels – the channels that are mutated in hyperkalemic periodic paralysis. These Na+ channels open, eliciting propagation of an action potential along the membrane. Following this, and in response to depolarization, voltage-gated K+ channels open, resulting in the downswing of the action potential. The action potential therefore conducts rapidly along the sarcolemma in a wavelike fashion away from the neuromuscular junction. Action potentials are conducted into the interior of muscle fibers via the T-tubules and there they activate voltage-gated channels known as dihydropyridine receptors (DHPR). Unlike in cardiac muscle, very little calcium enters the muscle fiber from the extracellular space (via the DHPR).65 Instead, a mechanical link between DHPR and the SR Ca2+ release channel (ryanodine receptor, RYR1) at the junctional feet of the triads causes release of calsequestrin-bound Ca2+ from the SR’s interior (Fig. 6.12). A positive feedback loop, known as calcium-induced calcium release, is responsible for further activation of RYR1, with the result that the calcium concentration within the cytoplasm increases about 100-fold from a resting concentration of approximately 50 nM.65 The anatomical location of the terminal cisternae results in Ca2+ being released adjacent to the overlapping contractile apparatus. Binding of Ca2+ to high-affinity binding sites on troponin C causes a conformational change to the troponin–tropomyosin complex (see Fig. 6.5B). This results in the exposure of the myosin-binding sites on F-actin and allows the myosin globular head to attach as ATP is hydrolyzed, thus forming the crossbridge. Force generation and shortening of the sarcomere occur as a result of a conformational change of the myosin head. Adenosine diphosphate and inorganic phosphate are displaced by actin, which is followed by dissociation of the actin–myosin bridge due to the binding of ATP to myosin again. The crossbridge cycle continues while the cytoplasmic Ca2+ concentration remains high. Relaxation is achieved when the Ca2+ is resequestered within the SR via the action of the Ca2+– ATPase pumps (see Fig. 6.13 for a summary). The force that is generated in the crossbridge cycle is transmitted via the contractile apparatus to intermediate filament proteins that act to maintain and stabilize the muscle fiber’s shape during contraction. These intermediate filaments provide a structural link first to the sarcolemma and then to the extracellular matrix via a group of proteins known as the dystrophin-associated protein complex (Fig. 6.14, Fig. 6.15).66 Contractile forces are transmitted from each muscle fiber via the extracellular matrix and the connective tissues of tendons, and ultimately to the bones of the skeleton. ATP replenishment in (predominantly) oxidative fibers requires a readily available source of oxygen that is provided by the O2-binding heme protein known as myoglobin. The P50 for equine myoglobin (the PO2 when it is 50% saturated) is about 2.4 mmHg at physiological temperatures and pH,67 and therefore far to the left (lower) than the P50 of equine hemoglobin (23.8 mm/Hg) and close to the PO2 of muscle cells. During exercise, oxygen demand rises dramatically, met by a 20–30 times increased blood flow through the muscle capillary beds.4 Capillary dilation results partly from autonomic control and stretch imposed by the higher blood pressure, but also follows the local production of vasoactive substances that include potassium, adenosine, and nitric oxide. The latter is produced by nitric oxide synthase, found both in the endothelium of the capillaries and bound to the dystrophin-associated protein complex within the contracting muscle fibers themselves (Fig. 6.16). where ADP is adenosine diphosphate and Pi is inorganic phosphate. Within mitochondria, β-oxidation of free fatty acids (FFA), the tricarboxylic acid (TCA) cycle, and oxidative phosphorylation (via the electron transport chain) combine to produce ATP aerobically (Fig. 6.17). During the process, the coenzymes nicotinamide adenine dinucleotide (NAD) and flavin adenine dinucleotide (FAD) are reduced to NADH2 and FADH2, respectively. Subsequently, NADH2 and FADH2 are reoxidized to NAD and FAD via the electron-transport chain in which oxygen acts as the final hydrogen acceptor to form water. Oxygen dissolved within the cytoplasm and bound to myoglobin is rapidly used up and must be replenished; hence oxidative phosphorylation depends on a dense capillary network between muscle fibers (Fig. 6.18). Acetyl-CoA is the substrate for the TCA cycle and its complete oxidation results in the formation of 12 ATP molecules. Acetyl-CoA is derived from pyruvate following anaerobic metabolism of glucose and glycogen within the cytoplasm (glycolysis) (see Fig. 6.17); at submaximal exercise intensities, most pyruvate produced via glycolysis is transported into mitochondria and is converted to acetyl-CoA, which enters the TCA cycle. Thirty-six molecules of ATP are produced in the complete breakdown of glucose via these pathways. However, acetyl-CoA is also derived from the oxidation of fatty acids, following their mobilization from the liver or adipose tissue. Beta-oxidation of FFA is highly efficient, as complete oxidation provides up to 146 molecules of ATP. The enzymes that catalyze these reactions help buffer ATP concentrations at the expense of lowering the cellular concentration of phosphocreatine and free ADP, while increasing the concentrations of creatine, adenosine monophosphate (AMP) and inosine monophosphate (IMP). These reactions occur in active muscles at top speeds, but provide only a small amount of ATP for a few seconds. Deamination of adenosine nucleotides leads to the production of ammonia (NH3), uric acid, and allantoin.68 The second anaerobic pathway involves glycolysis acting independently from the oxidative pathways. Glycolysis requires glucose-6-phosphate as a substrate, which may be derived from the phosphorylation of glucose by hexokinase or by the mobilization of stored intracellular glycogen that is metabolized first to glucose-1-phosphate via glycogenolysis (see Fig. 6.17) and then converted to glucose-6-phosphate. Blood glucose is transported across the sarcolemma by means of specific glucose transporters that include GLUT-1 and GLUT-4 (see Fig. 6.18).69 GLUT-1 is normally located within the sarcolemma and provides basal glucose requirements; however, GLUT-4 receptors translocate to the sarcolemma in vesicles, in response to insulin or the demands of exercise (Fig. 6.19). The glycolytic pathways result in the formation of two pyruvate molecules that in the absence of oxygen are converted to lactate. Aerobic production of ATP is a relatively slow but highly efficient process, while anaerobic pathways produce energy rapidly but relatively inefficiently. Although both pathways are generally active during exercise, the relative contribution within each muscle depends on the nature, intensity level and duration of the activity, the muscle’s fiber-type composition, the availability of oxygen and substrates, and the relative concentrations of intermediary metabolites that may potentially activate or inhibit selected enzymes.68 Hence, at the beginning of low-speed exercise when oxygen is abundant, energy production depends largely on metabolism of glycogen via aerobic pathways.68 Within a few minutes, glucose and FFA concentrations rise in the blood and following 20–30% glycogen depletion, there is a shift towards β-oxidation of FFA70 With higher energy demands, the muscle ATP/ADP ratio decreases, providing a stimulus for energy production via anaerobic mechanisms. The activity of the key regulatory glycolytic enzyme phosphofructokinase increases, causing greater production of pyruvate via glycolysis. The point where the availability of oxygen becomes a limiting factor in oxidative phosphorylation is reflected by partial reoxidation of NADH2, as more and more pyruvate is converted to lactate. As exercise intensity increases, the anaerobic pathway supplies a greater proportion of energy. The point when the increased rate of lactate production can be detected in the plasma is known as the anaerobic threshold. This threshold varies and depends on several factors including the muscle’s fiber-type composition and the level of fitness of the horse. Furthermore, diet plays an additional role; for example, a fat-rich diet promotes oxidative energy production via FFAs,71 thereby increasing the oxidative capacity of muscle72 and sparing glycogen.72 However, other substrates also influence the pathways employed during energy production; for instance, energy production can be steered towards that derived from glucose by the provision of additional glucose during exercise.73 There are important differences in the morphologic, physiologic, and biochemical properties of fibers both within and between muscles. These differences form the basis for the classification of fiber types. Better understanding of the expression patterns of groups of proteins within individual fibers has allowed refinement of fiber-type classification. For example, myofibrillar proteins exist as different isoforms encoded by separate genes that are expressed in a myofiber type-specific and coordinated manner.74 Fiber types can best be differentiated by analyzing the specific myosin heavy chain (MyHC) isoform(s) expressed by each fiber, since MyHC composition closely reflects each fiber’s phenotype.23 Three MyHC isoforms have been characterized in adult equine skeletal muscles at the protein,27 mRNA,31 and cDNA,32 levels; they are designated as types I, IIA, and IIX27 (or IID)29 (henceforth IIX). The differential distribution of these MyHCs defines three pure fiber types containing a single isoform (types I, IIA, and IIX) and two hybrid fiber types coexpressing two isoforms (I+IIA and IIA + IIX (IIAX)) (Fig. 6.20). Hybrid IIAX fibers exist in equine locomotor muscles in significant numbers,29,30 although the coexpression of IIA and IIX MyHCs at the protein level seems not to be reflected by cotranscription of the corresponding genes (Fig. 6.21).33 This suggests that hybrids might represent fibers that are undergoing committed fiber type switching, towards the type corresponding to the mRNA currently being expressed, and that this conversion occurs in equine muscle not subjected to any specific training stimulus.33 Some studies have demonstrated either minimal (less than 0.6%)75 or no expression of the MyHC-IIB isoform in locomotor muscles of the horse,27,31–33 hence the fibers classified as type IIB in earlier studies are now more appropriately classified as type IIX. It remains unclear why the MyHC-IIB gene is only minimally (or not) expressed in various ungulates,32 although it could be related to body size and muscle fiber length. As a muscle’s maximum contraction velocity is proportional to the number of sarcomeres in series76 and the MyHC-IIB isoform exhibits the fastest shortening velocity,74 the expression of this isoform in the very long muscle fibers of the horse would, in theory, result in an extremely high contraction velocity. Nevertheless, some recent studies have demonstrated the expression of this isoform in the skeletal musculature of some other large mammals (pig and llama),77,78 indicating that the expression (or lack) of this isoform is not only a reflection of body size. Certain important differences between the various equine skeletal muscle fiber types are illustrated in Figure 6.22 and summarized in Table 6.1. When studied in combination, these differences allow more objective delineation (Fig. 6.23) and represent the considerable interdependence of contractile, metabolic, and morphologic features. Type I fibers have a MyHC isoform that hydrolyzes ATP slowly, resulting in a slow crossbridge cycle. These fibers have a small cross-sectional area, a high number of capillaries and high oxidative capacity. However, their glycolytic capacity and glycogen content are relatively low. Together, these properties make type I fibers highly efficient and economical in producing slow repetitive movements and sustaining isometric force, but not significant power generators. Table 6.1 Quantitative fiber type features of equine skeletal muscle1 1Values are mean ± SE. Within a row, means with different letters are statistically different (P < 0.05), where a expresses the lowest value and e the highest. In the absence of significant differences between type II fibers, values are presented as pooled means for all type II fibers (n = 157 fibers). OD = optical density. 2Hybrid I+IIA fibers are not considered in this analysis; IIAx = hybrid fibers with a predominant myosin heavy chain type IIA content; IIaX = hybrid fibers with a predominant myosin heavy chain type IIX isoform. 3See Figure 6.22‘s legend for origins and specificities of antibodies. 4Ac-mATPase = myofibrillar ATPase after acid (pH 4.45) pre-incubation; Alk-ATPase = myofibrillar ATPase after alkaline (pH 10.45) pre-incubation; Qu-mATPase = quantitative myofibrillar ATPase activity (pH 7.6). 5SDH = succinate dehydrogenase activity; GPD = glycerol-3-phosphate dehydrogenase activity; PAS = periodic acid–Schiff. 6CSA = cross-sectional area; cap = number of capillaries; nuc = nuclear number. 7SERCA = sarco(endo)plasmic reticulum Ca2+-ATPase; PLB = phospholamban; see 23 for sources and specificities of these antibodies.
Muscle physiology
Responses to exercise and training
Muscular response to exercise
Overview
134 ± 2 mL/min/kg) has been estimated at 226 L/min, which represents approximately 78% of total cardiac output.4 This exercise requires the integration of many different body systems under the control of the nervous system (Fig. 6.1). Metabolites and oxygen reach skeletal muscle fibers via the respiratory, cardiovascular, and hematologic systems; in turn muscle fibers produce energy in the form of adenosine triphosphate (ATP), which, via the contractile machinery, is converted into mechanical work. The structural arrangement of the musculoskeletal system provides the means with which to harness this energy, either moving the horse’s limbs in a characteristic rhythmic pattern for each gait, or enabling diaphragm contraction, which contributes substantially to inspiratory effort.
Methodology
Percutaneous needle biopsy technique
Laboratory methods
Other techniques
Muscle structure and function
Morphology
Development
Gross anatomy and muscle function
Histology
Ultrastructure
General muscle physiology
The motor unit
Muscle proprioception
Electrical and ionic properties of the sarcolemma
Excitation–contraction coupling
Force transmission
Oxygen availability
Energy provision for muscular functions
Aerobic pathways
Anaerobic pathways
Integration of aerobic and anaerobic pathways
Muscle heterogeneity
Muscle fiber types
Fiber type differentiation
Muscle fiber type properties
MUSCLE FIBER TYPES2
I
IIA
IIAx
IIaX
IIX
No. of fibers
51
80
25
25
27
Anti-MyHC monoclonal antibodies3
BA-D5 (OD)
0.60 ± 0.02 b
0.34 ± 0.02 a
SC-71 (OD)
0.30 ± 0.02 a
0.52 ± 0.03 d
0.46 ± 0.02 c
0.34 ± 0.01 b
0.34 ± 0.01 b
BF-35 (OD)
0.47 ± 0.03 c
0.49 ± 0.03 d
0.46 ± 0.03 cd
0.36 ± 0.02 b
0.30 ± 0.02 a
S5-8H2 (OD)
0.44 ± 0.02 d
0.33 ± 0.01 a
0.37 ± 0.02 b
0.41 ± 0.01 c
0.41 ± 0.01 c
Myofibrillar ATPase activity4
Ac-mATPase (OD)
0.77 ± 0.02 d
0.28 ± 0.03 a
0.34 ± 0.03 b
0.47 ± 0.02 c
0.50 ± 0.02 c
Alk-mATPase (OD)
0.27 ± 0.02 a
0.37 ± 0.03 b
0.46 ± 0.04 c
0.52 ± 0.02 d
0.54 ± 0.02 d
Qu-mATPase (OD/min)
0.30 ± 0.02 a
0.39 ± 0.02 b
0.42 ± 0.01 c
0.45 ± 0.01 d
0.51 ± 0.01 e
Metabolic properties5
SDH (OD/min)
0.49 ± 0.02 d
0.46 ± 0.03 c
0.37 ± 0.02 b
0.34 ± 0.02 b
0.24 ± 0.03 a
GPD (OD/min)
0.33 ± 0.02 a
0.36 ± 0.03 b
0.41 ± 0.01 c
0.46 ± 0.01 d
0.47 ± 0.01 d
PAS (OD)
0.34 ± 0.02 a
0.45 ± 0.03 b
Fiber size, capillaries, and myonuclei6
CSA (µm2)
3124 ± 723 a
3339 ± 763 b
4623 ± 807 c
5039 ± 1293 c
7635 ± 2930 d
cap/103 µm2
2.06 ± 0.94 b
1.96 ± 0.57 b
1.60 ± 0.41 ab
1.38 ± 0.21 a
1.23 ± 1.07 a
nuc/103 µm2
2.26 ± 0.65 b
2.32 ± 1.02 b
1.65 ± 0.36 ab
1.44 ± 0.78 a
1.11 ± 0.70 a
Anti-SERCA and PLB antibodies7
CaF2–5D2 (OD)
0.33 ± 0.03 a
0.44 ± 0.03 b
MA3–910 (OD)
0.48 ± 0.02 b
0.38 ± 0.03 a
05–205 (OD)
0.54 ± 0.03 b
0.37 ± 0.02 a
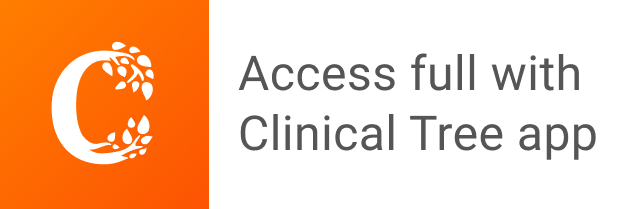