CHAPTER 12 As illustrated throughout this book, the horse has evolved into the supreme athlete, being capable of both high speeds and endurance. Fine neuromuscular coordination, strength, and stamina allow a Thoroughbred to attain speeds in excess of 18 meters per second (m/s) or 65 kilometers per hour (km/h), which can be maintained for about 1 minute with a stride length of up to almost 7.5 m. This feat is possible because the muscular system of the horse has evolved to produce powerful, efficient movement at high speeds. In most mammalian species, muscle mass makes up about 40% to 45% of body weight; however, in the Thoroughbred, muscle mass can comprise up to 55% of body weight (Gunn, 1979). In addition to increased muscle bulk, elite performance is achieved by adaptations of muscle at many different levels: the gross arrangement of muscle groups, the architecture of muscle fibers and connective tissue within muscles, and the highly specialized ultrastructure of the muscle fibers themselves. Our understanding of muscle anatomy in the horse can be traced back through centuries of study of the organization and attachment of muscles and their tendons. The details of the cellular organization of muscle fibers in the horse, however, have only been characterized during the last few decades. On an ultrastructural level, much of our current understanding of the horse still relies on parallels drawn from other animal species. Locomotor muscles in the horse are strategically located proximally on the skeleton, creating a pendulum-like effect that decreases the energy necessary to swing the limb. The arrangement of spindle-shaped muscle cells (muscle fibers) within the muscle also maximizes efficiency and power output during locomotion. In many limb muscles, muscle fibers are arranged in a “pennate” shape whereby they join one or more tendinous insertions at an angle to the direction of force. This maximizes the cross-sectional area and power output of the muscle in relation to the limited space available on the upper limb. Other muscles, strap muscles, maximize their range of movement by a parallel organization of muscle fibers along the direction of force. Several perpendicular tendinous insertions are usually present within this type of muscle, since most muscle fibers are only about 5 to 10 centimeters (cm) in length, with a cross-sectional area of 2000 to 6000 micrometers squared (μm2) (Karlstrom et al., 1994). For efficiency, muscle fibers are grouped within the muscle such that the slower-contracting fibers commonly used for postural support are frequently located deeper in the muscle and the faster-contracting fibers used for higher speeds are located more superficially. The eventual mosaic of contractile fiber types within a muscle will be determined by innate developmental directives, temporal and positional factors, neural innervations, and activation of specific signal transduction pathways. Positional factors dictate that those portions of muscles that are primarily postural have a higher percentage of slow twitch fibers, whereas neural innervations dictate that all fibers supplied by the same nerve branch have the same muscle fiber type. In the equine fetus, the perinatal form of contractile protein within the myosin heavy chain (MHC) is initially expressed. However, differentiation of slow-contracting and fast-contracting muscle fibers becomes well established at 158 days of gestation and evident in newborn foal muscle. Fast twitch fibers may continue to coexpress perinatal MHC in foals up to 10 weeks of age, and many slow twitch fibers may coexpress a cardiac MHC isoform up to 22 weeks of age (Dingboom et al., 1999). Development of subtypes of fast twitch fibers (2a, 2ax, or 2x) occurs in concert with the emergence of thyroid function (Russell et al., 1988). Muscle fiber composition remains plastic well into adulthood, affected by growth and training. Contractile proteins: Myofibrils comprise a highly repeating arrangement of filaments of noncovalently associated proteins connected in series within the length of each muscle fiber (Figure 12-1). The number of myofibrils in a muscle fiber varies with its contractile type and cross-sectional area. Each myofibril is 1 to 3 μm in diameter and has a polygonal shape. The organization of numerous myofibrils in register within a cell gives skeletal muscle fibers a striated appearance under the light microscope. Sarcomere: The repeating unit of myofilaments within the myofibril is referred to as a sarcomere, the fundamental unit of contraction (see Figure 12-1). Filaments of actin (molecular weight 43 kilodaltons [kDa]) traverse the sarcomere at each end to form the Z-line. The width of the Z-line varies from 50 nanometers (nm) in slow-contracting muscle fibers to 65 nm in fast-contracting muscle fibers. Thin myofilaments extend axially from either side of the Z-line and form a hexagonal array around overlapping thick myofilaments. Muscle contractions occur when, within each sarcomere, thin myofilaments slide over the thick myofilaments bringing consecutive Z-lines closer together. This complex arrangement of overlapping filaments permits several distinctive areas to be identified with electron microscopy. The I-band contains the Z-line centrally and extends to include the adjoining area of nonoverlapping thin myofilaments. Centrally, in the sarcomere, the A-band is defined by the full extent of the thick myofilaments. Within the A-band, the H-band is defined by the central area where thick myofilaments do not overlap with thin myofilaments. In the middle of the H-band, a dark line is formed by three to five M-line filaments traversing the sarcomere (see Figure 12-1). Thick myofilaments: The thick myofilaments are bipolar, spindle-shaped structures 1.6 μm in length and 15 nm in diameter. Myosin is the primary protein in the thick myofilament with a molecular weight of 460,000 kDa, and it possesses both structural and enzymatic properties. Myosin is composed of two identical heavy chains (polypeptide chains with an approximate molecular mass of 200 kDa) and two pairs of light chains (polypeptide chains with molecular masses ranging from 16 to 27 kDa) (Figure 12-2, A). The two myosin heavy chains are arranged in a double helix to form a long stable tail at one end. At the opposite end, each heavy chain is folded to form one globular, pear-shaped head. Four myosin light chains are contained within the globular heads (two per head) near the junction of the head and neck domains. The composition of MHCs within sarcomeres varies between individual muscles and between individual muscle cells. Equine skeletal muscle cells may express the following distinct heavy chains: perinatal (or neonatal), slow, fast type 2a, fast type 2x (or 2d) or a hybrid of 2a/2x (Dingboom et al., 1999; Eizema et al., 2005). The speed of contraction of these myosin heavy chain isoforms increases in the order listed above. Thin myofilaments: Thin myofilaments are 1 μm long and 8 nm in diameter, consisting primarily of actin. Actin is a globular protein with a molecular weight of 43 kDa. Two F-actin strands twisted in a double helix form the backbone of the thin myofilament and possess a complementary binding site for the myosin globular head. Actin has identical isoforms in fast-contracting and slow-contracting fibers. The interaction between myosin globular heads and actin is regulated by tropomyosin and troponin (see Figure 12-2, B). Tropomyosin is a two-stranded α-helix with a molecular weight of about 70 kDa, and it lies in the helical grooves formed by actin strands. Troponin is a complex of three noncovalently linked subunits, with molecular weights ranging from 18 to 35 kDa, which attaches at regular intervals to tropomyosin along the thin filament. Each subunit has a distinct physiologic function. The subunit TN-T, the tropomyosin-binding component, attaches the complex to tropomyosin at intervals along the thin myofilaments. The inhibitory subunit (TN-I), when bound to actin, acts together with tropomyosin to inhibit actin–myosin interaction. TN-C is the calcium-binding subunit of troponin, which, in the presence of calcium, can remove the inhibitory effects of TN-I and facilitate actin–myosin binding. Cytoskeleton: Both the organization of myofilaments within sarcomeres and the organization of myofibrils in myofibers are supported by a complex cytoskeletal network of intermediate filaments (Wang and Ramirez-Mitchell, 1983). Intermediate filaments, as well as a number of accessory proteins that form fine filaments, function to (1) maintain the alignment of myofilaments and sarcomeres, (2) attach and maintain alignment of adjacent myofibrils, (3) attach and transfer forces from the sarcomeres of peripheral myofibrils to the sarcolemma, and (4) connect terminal sarcomeres to the sarcolemma at myotendinous junctions. In addition, cytoskeletal proteins serve a vital role in intracellular signaling and in maintaining the shape of organelles such as mitochondria and nuclei. Titin is one of the largest cytoskeletal proteins, which attaches thick myofilaments to Z-lines, and in the I-band region this attachment imparts a passive elasticity to sarcomeres (Maruyama, 1999). An additional cytoskeletal protein, nebulin, forms small filaments that run the length of thin myofilaments, which may regulate the length of thin myofilaments. Both M-protein and creatine kinase are cytoskeletal elements located at the M-line that may serve as structural support for the thick filaments by linking neighboring filaments to each other, in addition to providing a source of ATP from creatine phosphate. A-actinin and vinculin, among other cytoskeletal proteins at myotendinous junctions, attach the thin myofilaments of the last sarcomere to the sarcolemma. At the periphery of myofibrils, adjacent Z-lines within the same sarcomere are connected by intermediate filaments of desmin. Also, intermediate filaments of desmin encircle the circumference of Z-lines and appear to form linkages with Z-lines of adjacent myofibrils to aid in the alignment of sarcomeres in register. Desmin filaments are also anchored to the sarcolemma by a number of adhesion proteins such as vinculin. The cytoskeletal elements that connect the sarcomere to the basement membrane are called costameres. They serve to laterally transmit contractile forces from sarcomeres across the sarcolemma to the basement membrane and potentially to convert mechanical stimuli to alterations in cell signaling and gene expression. To date, the distribution of only one cytoskeletal protein, tubulin, has been studied in the horse (Horak et al., 1991). Sarcolemma and sarcoplasmic reticulum: Muscle basement membrane: The basement membrane surrounds skeletal muscle fibers, is directly linked to the sarcolemma, and plays a critical role in myogenesis, muscle fiber development, regeneration, structure, and function. In addition, it is required for the proper assembly of the neuromuscular and myotendinous junctions. Trans-sarcolemma complexes provide critical mechanical links among the basement membrane, the cytoskeleton, and the myoplasm. Two such sarcolemma protein complexes include (1) the dystrophin–glycoprotein complex and (2) integrins (Michele and Campbell, 2003). The dystrophin–glycoprotein complex likely plays a key role in protecting the sarcolemma from mechanical damage during muscle contraction. It is grouped into three subcomplexes: (1) dystroglycan (α- and β-dystroglycan), (2) the sarcoglycan–sarcospan subcomplex, and (3) the cytoskeletal components dystrophin, syntrophin, and dystrobrevin (Ervasti and Sonnemann, 2008). These proteins are well characterized in other species and their absence causes various forms of muscular dystrophy; however, there are no specific studies of these complexes in horses. Sarcolemma: The sarcolemma maintains the intracellular milieu, actively transports substrates into the muscle cell, serves as a docking location for proteins originating in the basement membrane and cytoskeleton, and also transmits neural excitatory impulses that lead to muscle contraction. Facilitated diffusion of glucose across the sarcolemma occurs via glucose transporters (GLUT). GLUT-1 is constitutively present in the sarcolemma and provides basal amounts of glucose uptake, whereas GLUT-4 is present in the endosomes in the sarcoplasma, which migrate to and then dock and fuse with the sarcolemma when stimulated by insulin and contraction-dependent processes. Long-chain fats are transported across the sarcolemma by fatty acid translocase. The sarcolemma forms tubular invaginations, t-tubules, at regular intervals along its length which traverse perpendicularly across the myofibrils at the junction of the A-bands and I-bands (Figure 12-3). The t-tubule membranes have a lower protein content but similar lipid content as the sarcolemma and contain numerous voltage-gated calcium channels called dihydropyridine receptors (DHPR). The t-tubules serve to transmit electrical impulses into the interior of the muscle fiber, where, through association with the intracellular membranous system, they can almost simultaneously initiate myofibrillar contraction. Neuromuscular junctions: The neuromuscular junction is a specialized region on the sarcolemma, where a motor neuron axon terminal rests. The synaptic cleft is the space between the axon terminal and the postsynaptic sarcolemma (motor end plate), and it is filled with basal lamina containing acetylcholinesterase. Depolarization of the motor neuron results in calcium-dependent exocytosis of the chemical transmitter acetylcholine (ACh) from the small vesicles in the presynaptic axon terminal. The ACh that is released diffuses across the synaptic cleft to bind with acetylcholine receptors (AChRs) in the postsynaptic sarcolemma. Excitation of the myofiber is initiated by the reversible binding of ACh with AChRs. The binding of ACh to AChRs is transient, and its effects are abolished by the diffusion of ACh away from the receptors and its hydrolysis by acetylcholinesterase. The binding of ACh with AChR results in a local depolarization of the postsynaptic membrane caused by the transient increased conductance of sodium. When a threshold is reached, voltage-gated sodium ion channels in the synaptic clefts and along the sarcolemma are activated, and a wave of depolarization extends into the myofiber via contiguous t-tubules. Sarcoplasmic reticulum: The intracellular membranous system of skeletal muscle, or sarcoplasmic reticulum (SR), is physically separate from the sarcolemma and surrounds each myofibril in a highly repetitive pattern. The SR membranes contain a high concentration of calcium ATPase, the protein calsequestrin, and the calcium release channel called the ryanodine receptor (RYR). This system of membranes sequesters calcium in the relaxed muscle fiber, leaving extremely low concentrations in the sarcoplasm surrounding the myofibrils. Over the A-bands and I-bands, the SR runs parallel to the myofibrils. At the A–I junction, the SR tubules change their membrane composition and converge to form terminal cisternae (see Figure 12-3). The terminal cisternae run perpendicular to the myofibril on either side of the t-tubules. The t-tubules, along with the two neighboring terminal cisternae, form a functional association called a triad. A triad occurs twice in each sarcomere. The gap between the terminal cisternae and t-tubules is bridged by a tetragonal arrangement of foot processes called junctional feet. These feet act structurally to maintain the architecture of the triad during contraction and to mediate the coupling of sarcolemmal excitation with the release of calcium for muscle contraction. They do this by maintaining a proximal relationship between the voltage-gated dihydropyridine receptor (DHPR) in the sarcolemma and the RYR located in the terminal cisternae of the sarcoplasm. Excitation–contraction coupling: Excitation–contraction coupling is the transformation of depolarizing events in the sarcolemma into the initiation of mechanical shortening of the myofibrils. The action potential that is propagated into the depths of the myofiber via transverse t tubules triggers the voltage-gated DHPR located within the triads. Activation of the DHPR triggers the release of calcium ions from the terminal cisternae into the sarcoplasm by opening the RYR in the SR membrane. This elevates the calcium ion concentration surrounding the myofilaments in the sarcoplasm from 0.1 micromolar (10-7 moles/L), to more than 10 micromolar (10-5 moles/L). Accessory proteins that regulate RYR function include calmodulin and the FK-506–binding protein. Other organelles: Skeletal muscle fibers contain hundreds to thousands of postmitotic nuclei, which are located directly underneath the sarcolemma. The number of myonuclei per fiber is established at birth. Regeneration of myofibers is dependent on small satellite cells, which are situated between the basement membrane and the sarcolemma. In light microscopy, satellite cell nuclei could be easily confused with myonuclei. A varying complement of smooth and rough endoplasmic reticulum, Golgi apparatuses, and lysosomes is usually found near myonuclei. Numerous proteins, including myoglobin and the enzymes involved in glycolysis, are distributed in the sarcoplasm. Enzymes involved in oxidative metabolism are located within the mitochondrial membranes. Mitochondria in horse muscle are concentrated in subsarcolemmal locations, particularly in association with capillaries. A lesser distribution of mitochondria is found between myofibrils. The volume density of mitochondria varies in different horse muscles and in different breeds from 2% to 24% (Kayar et al., 1988). Equine mitochondria are generally cylindrical in shape but may produce transverse extensions at the level of the I-band. Glycogen granules and, to a varying extent, lipid droplets are also distributed throughout the sarcoplasm between the myofilaments and under the sarcolemma. Glycogen is stored in intracellular granules composed of self-glycosylating protein primer called glycogenin and variable amounts of glucose joined by straight α-1, 4 or branched α-1, 6 glycosyl linkages. This structure is treelike in its branching and allows glycogen granules to be potentially regulated individually and regionally within a cell for metabolism. Glycogen synthesis of new granules is catalyzed by glycogenin. It generates an oligosaccharide primer of 7 to 11 glycosyl units, which serves as a substrate for glycogen synthase. In combination with glycogen-branching enzyme, glycogen synthase forms glycogen granules, which, in the initial stages, have a low molecular weight and a high protein-to-carbohydrate ratio and can precipitate in acid (Brojer et al., 2002). These glycogen granules are termed proglycogen and have a molecular weight of up to approximately 400 kDa. When proglycogen granules grow larger by the addition of glucose residues, they are termed macroglycogen. These macroglycogen granules are acid soluble and range in size from 400 to 10,000 kDa. Studies in horses have revealed that these two fractions of glycogen granules respond differently during times of glycogen catabolism and anabolism (Brojer et al., 2002; Brojer et al., 2006). The development of the muscle biopsy technique brought many new insights into skeletal muscle form and function in the horse (Lindholm and Piehl, 1974; Snow and Guy, 1976). Collected muscle samples were analyzed with a range of physiologic, biochemical, histochemical, and molecular techniques to understand the influences of growth, training, diet, and genetics on equine muscle function. Open surgical techniques provide large superficially located muscle specimens for study, and the percutaneous needle muscle biopsy technique provides insight into a variety of muscles, sample depths, and longitudinal studies of muscle responses to exercise and training. The muscle most frequently examined in the horse by percutaneous biopsy is the gluteus medius, or the middle gluteal muscle. This muscle is easily sampled in the unsedated horse, is very active during exercise at a range of speeds, and demonstrates a metabolic response to exercise and training adaptation. Biopsies of the semimembranosus or the semitendinosus are also used in metabolic and training studies, but these can be more difficult or dangerous to obtain in unsedated horses. Although the quadriceps muscles are the muscle of choice in human studies, in horses, this muscle does not show nearly the same degree of training adaptation (Essen et al., 1980). This may be because the quadriceps in horses serves more of a role in the stay apparatus to lock the patella in place at rest rather than to advance the limb during locomotion. Unless otherwise stated, the metabolic responses and training effects reviewed in this chapter refer to the gluteal or the semitendinosus or membranosus muscles. Percutaneous needle muscle biopsy safely and relatively atraumatically provides specimens satisfactory for histochemical, biochemical, ultrastructural, and molecular studies (Figure 12-4). In addition, repeated sampling can be carried out during and following exercise bouts without any adverse effect on performance. Following removal of the core of the muscle, the tissue is replaced by regeneration of myofibers rather than by fibrous tissue. Samples ranging from 200 to 500 milligrams (mg) are often obtained using the needle technique. If larger samples are required for investigation, an open excision biopsy of the semitendinosus or semimembranosus is often the most expedient choice. Muscle fiber types vary among different muscles in the same horses as well as across horses and breeds (Essen et al., 1980; Snow and Guy, 1980). Standardization of the site of the muscle biopsy is imperative because of the heterogeneous distribution of muscle fiber types both within the depth and along the length of the same muscle. Deeper regions within locomotor muscles have contractile and metabolic characteristics similar to postural muscles (Rivero et al., 1993a). When sampling site and depth are consistent, repeatable results are obtained (Rivero et al., 1993a). For gluteal muscle biopsies, a site along a straight line from the most dorsal point of the tuber coxae to the head of the tail is often used. A specific length along that line is selected within a given study, usually 15 to 17 cm from the dorsal aspect of the tuber coxae and a specific depth is selected, usually 4 to 8 cm in an adult horse. The depth and length need to be reduced for studies of younger animals to reflect the same relative part of the gluteal muscle as examined in adults. Prior to obtaining a biopsy, an area of skin of approximately 2.5 cm2 is shaved, washed, and cleaned with surgical spirits or equivalent. Three milliliters (mL) of local anesthetic is then injected subcutaneously at the site of the proposed incision and into the fascia overlaying the muscle, but not into the muscle itself. An incision about 1 cm long is made through the skin and, where necessary, the fascia. The needle together with the cutting cylinder is then inserted into the muscle. Once within the muscle, the cutting cylinder is partially withdrawn, so that the window is opened up. The window is positioned such that it is upward or sideward and pressed firmly against the muscle to catch a small piece of muscle within the needle. Finally, the cutting cylinder is pushed down to detach the trapped muscle. This can be repeated several times so that between 50 and 250 mg of muscle is obtained. The needle is carefully withdrawn, and the excised muscle is removed with the stylet. Good technique allows a 1-cm to 2.5-cm cylindrical-shaped piece to be obtained. The incision is allowed to heal without suturing or with one nonabsorbable suture if a gap exists (see Figure 12-4). This procedure usually can be carried out without using tranquillizers and with minimal restraint. The author has carried out thousands of biopsies with negligible adverse effects. Rarely, a hematoma may result if a larger blood vessel is accidentally cut during the biopsy. Myosin–atpase differentiation: Histochemical methods based on the sensitivity of myosin ATPase to acid and alkaline preincubation usually identify three contractile fiber types in the gluteal and semitendinosus muscles from horses. Following preincubation at pH 10.4, type I fibers have low myosin ATPase activity, and type II fibers have high activity, whereas this activity is reversed following incubation at pH 4.3. These reactions reflect slow- and fast-contracting fibers, respectively, and therefore are also referred to as slow (type I) and fast (type II) twitch fibers. Type I fibers also have a slower relaxation time and are more fatigue resistant than are type II fibers. Type II fibers can be further divided into the subtypes IIA, IIB, and IIC, according to the lability of myosin ATPase following preincubation at either acidic or alkaline pH (Brooke and Kaiser, 1970) (Figure 12-5). A continuum of staining intensity usually exists between the type IIA and IIB fibers (Linnane et al., 1999) (see Figure 12-5). Type IIC fibers do not reverse staining activity in alkaline as well as acid pH and likely represent fibers containing both slow and fast myosin. They can be found in muscles of very young animals but are rare in mature animals unless the fibers are regenerating after an injury. Immunohistochemical differentiation: Monoclonal antibodies for specific myosin isoforms provide a more accurate means to discern fiber types in equine skeletal muscle (Linnane et al., 1999). MHC fiber types are based on three adult MHC isoforms that define five fiber types: pure type 1, 2a, and 2x fibers and the hybrids (i.e., coexistence of two MHC isoforms) 1+2a and 2a+2x fibers (Pette and Staron, 1997; Pette and Staron, 2000). Thus, a continuum of activity exists in the hybrid fibers. In situ hybridization with RNA probes specific for each MHC isoform show that the majority of fibers express identical mRNA and protein isoform, whereas hybrid fibers present a mismatch between coexpression at the protein level rather than at the mRNA level (Eizema et al., 2005). The nomenclature for contractile fiber types differs between myosin ATPase histochemistry and MHC immunohistochemistry. Type IIB fibers were originally named because their myosin ATPase staining properties resembled those of rodent type IIB fibers. However, MHC immunohistochemistry revealed that equine type IIB fibers do not correspond to 2b MHC found in the fastest-contracting rodent muscle fibers. Further, no complementary deoxyribonucleic acid (cDNA) encoding the 2b gene has been identified in horses. Rather, fibers originally identified as IIB by ATPase stains in horses actually most closely correspond to type 2x or type 2a/x MHC (Linnane et al., 1999). Speed of contraction: Using skinned single muscle fibers from the equine soleus, which contains three fiber types in horses, the maximum velocity ( Metabolic differentiation: In addition to fiber typing solely on the basis of contractile speed, divisions have been made in the horse solely on the basis of metabolic properties or, more commonly, in combination with myosin ATPase activity at pH 9.4. Usually, only oxidative capacity is determined as a metabolic marker, since it is accepted that type I fibers have relatively low glycolytic capacity and all type II fibers have high glycolytic activity. For the assessment of oxidative capacity, fibers are incubated for determination of either succinate dehydrogenase (SDH) or nicotinamide adenine dinucleotide tetrazolium reductase (NADH) diaphorase activity and classed as either having high or low activity (see Figure 12-5). In combination with myosin-ATPase activity at pH 9.4, this allows fibers to be classified into three categories: (1) slow-twitch high-oxidative (ST), (2) fast-twitch high-oxidative (FTH), and (3) fast-twitch low-oxidative (FT) fibers (see Figure 12-5). A fairly clear distinction can be made between high- and low-oxidative fibers in the untrained horse, but as training progresses, differentiation becomes more difficult because there is a continuum of activity similar to that seen for myosin-ATPase activity of type II fibers or hybrid 2a+2x fibers. Because type IIB (2x) fibers show the greatest change in oxidative staining with training, some researchers using a type I, IIA, and IIB classification subdivide the IIB fibers into low- and high-oxidative categories (see Figure 12-5). The oxidative differences shown histochemically also have been supported by biochemical studies on pools of single fibers (Valberg et al., 1988). The histochemical reactions for oxidative capacity only provide a relative difference between fibers in the same sample. Biochemical studies have shown that oxidative markers such as citrate synthase (CS) activity could vary as much as twofold in type I and IIA fibers within a horse, whereas histochemical staining showed a similar high-oxidative staining intensity in type I and IIA fibers. Because of the ability of type IIB fibers to have varying oxidative status depending on training status, it is important to realize that the subdivision of type II fibers using metabolic or contractile properties do not correlate exactly. The properties of type I and type II fibers are outlined in Table 12-1. Other features: The periodic acid Schiff’s (PAS) reaction shows equine muscle to be rich in glycogen and staining may be slightly less in type I than in type II fibers, whereas oil red O-stain for lipid content is relatively low in horses with intracellular lipid concentrated in the most highly oxidative fibers. Capillarization of fibers has been studied using the PAS reaction following glycogen removal with amylase (Karlstrom et al., 1994). Capillary number can then be expressed either in terms of per fiber or per unit area of fiber type. High-oxidative fibers have a greater capillarization than low-oxidative fibers. Not surprisingly, a relationship exists between the capillary supply and the functional and dimensional capacities of the cardiocirculatory system as well as the structural and biochemical properties of the muscle (Kayar et al., 1989) Immunofluorescent and immunohistochemical stains: Detailed identification of structures within myofibers can be accomplished using monoclonal antibodies coupled to fluorescent tags. The location of insulin-sensitive glucose transporters, GLUT4, in equine muscle either within intracellular storage pools and when actively inserted in the sarcolemma has been characterized by this method (Annandale et al., 2004). Furthermore, localization of isoforms of the calcium (Ca2+)–ATPase (SERCA) within equine skeletal muscle has been accomplished by SERCA immunohistochemistry (Eizema et al., 2007; Quiroz-Rothe and Rivero, 2001). Fiber areas: Fiber areas can be determined from histochemical preparations. Care has to be taken in selecting sections for this determination, since uneven muscle fiber contraction may occur within samples collected by needle biopsy. A more accurate indication of fiber size can be obtained by measurement of the minimum diameter, since this is not affected by any oblique orientation of the section. Measurement of fiber areas now can he carried out rapidly by using digitized planimetry and specialized software packages. Muscle biopsy investigations generally have found that fiber areas vary according to type, with the type 1 fibers being the smallest and the type 2x low-oxidative the largest, although this can vary between muscles and even within the depth of a muscle (Table 12-2). Fiber area measurements also depend on the histochemical preparation used, since sections reacted for myosin–ATPase activity result in measurements 10% to 20% less than those reacted for SDH (Snow and Guy, 1981). TABLE 12–2 Mean Cross-Sectional Area and Fiber Type Composition* *Determined from histochemical analysis and activity of the oxidative marker citrate synthase (CS) in pools of fibers of identified fiber type from two Standardbred and two Thoroughbred racehorses. Note the wide variation in oxidative capacity of all fiber types between horses. Ultrastructural differentiation: High-oxidative fibers have many more subsarcolemmal and intermyofibrillar mitochondria than low-oxidative fibers. Although histochemically type I fibers generally appear more highly aerobic than type IIA fibers, an examination of the semitendinosus revealed that the type IIA fibers had slightly higher mean volume density of mitochondria compared with type I fibers (Hoppeler et al., 1983). It also has been shown that horse muscle has a high mitochondrial content, helping explain the exceptionally high Biochemical assays: A more quantitative means to assess metabolic capacity of skeletal muscle is to measure the activity of enzymes or substrates in whole muscle homogenates or on pools of fibers or single fibers of identified type. Frequently used markers of oxidative capacity include assays of citrate synthase (CS) or succinate dehydrogenase (SDH) activity within the citric acid cycle or 3-OH-acyl-CoA dehydrogenase (HAD) in free fatty acid (FFA) oxidation. Glycolytic capacity is often assessed by determining lactate dehydrogenase (LDH) or phosphofructokinase (PFK) activities. Hexokinase (HK) activity is used to evaluate the capacity for phosphorylation of glucose. Type I fibers in untrained horses have higher activities of CS and HAD and lower activity of LDH compared with type II fibers. Type IIB fibers usually have the lowest oxidative and highest glycolytic activities. However, training can markedly influence these activities such that the CS activity in type IIB fibers of a trained racehorse can be greater than the CS activity of a type I fiber in an untrained horse (see Table 12-2) (Valberg and Essen-Gustavsson, 1987). Further assessment of the concentrations of triglycerides, glycogen, glucose-6-phosphate, pyruvate, lactate, and adenine nucleotides can be made by fluorometric or high-performance liquid chromatography (HPLC) assays of rapidly frozen muscle samples. Metabolite analyses on whole muscle must be evaluated with caution as this represents only a mean value for the metabolic responses in different fiber types. In some cases, analysis of single fibers may prove most elucidating (Essen-Gustavsson et al., 1997). Gene transcription: A rapidly developing application for equine muscle biopsies is the use of real-time reverse transcriptase–polymerase chain reaction (RT-PCR) which provides a means to evaluate gene transcription within muscle under varying metabolic stimuli. Gene sequence and transcription of lactate dehydrogenase, pyruvate kinase, myosin heavy chains, GLUT-4, sodium/potassium ATPase, myosin isoforms glycogen synthase, branching, and debranching enzymes have all been evaluated in horses. Microarrays for gene expression studies in horses are currently under development and should provide further insights into genes that are active in the muscle’s acute and chronic responses to exercise and their relationship to performance. Although very difficult to accurately measure, the number of fibers found in a particular muscle can vary considerably between breeds (Gunn, 1979). Animals bred for speed, such as the greyhound and Thoroughbred racehorse, have a greater number of fibers within the semitendinosus compared with “slower” breeds of these species. The difference is present in early life and becomes more pronounced in the mature animal. There is no reason not to suspect that similar differences occur in other key locomotor, if not all, muscles. Difference in fiber number may be largely under genetic control, although the possibility exists for training-induced hyperplasia. The fiber type composition of a muscle is expressed as the percentage of fibers of different types examined in a biopsy (out of at least 250 fibers examined) (see Table 12-2). Varying functional requirements result in muscle fiber type composition differing between muscles. However, the fiber type composition will also vary along the length and depth of a muscle due to compartmentalization. This effect on muscle fiber composition in horses has been studied in the horse in greatest detail in the middle gluteal muscle. The ease of sampling and its importance in locomotion make the middle gluteal the most frequently biopsied muscle in equine exercise physiology studies (see Figure 12-4). The middle gluteal muscle is divided into dorsal and ventral parts by a tendon that runs from the crest of the ilium to the greater trochanter (Bruce et al., 1993). The two compartments have separate points of origin and insertion. The ventral compartment is innervated by the cranial gluteal nerve and the dorsal by the caudal gluteal nerve. The dorsal region is visually considerably darker than the ventral region. Within each compartment, there is generally an increasing proportion of type I fibers with increasing depth, and almost complete disappearance of type IIB fibers occurs as the proportion of high-oxidative fibers increases (Table 12-3). In addition to an increasing proportion of type I and oxidative fibers, a change in fiber size occurs. In superficial sites, type I fibers have the smallest area, with type IIB being the largest, whereas in deeper sites, the predominant type I fibers are the largest. Biochemical variation also occurs through the muscle. Not surprisingly, the deeper within the muscle one goes, the higher are the CS and HAD activities and the lower are the phosphorylase and LDH activities. This diversity within the middle gluteal muscle suggests that the deeper portions have a more postural function, whereas the more superficial portions are recruited with increasing workloads. This nonhomogeneity also has been described in a number of other muscles. TABLE 12–3 *p <0.001; †p <0.01; ‡p <0.05. Adapted from Lopez-Rivero JL, Serrano AL, Diz AM, et al: Variability of muscle fiber composition and fiber sizes in the horse gluteus medius: an enzyme-histochemical and morphometric study, J Anat 181:1, 1992 with permission. Nonuniformity of fiber types within a muscle is an important consideration when trying to compare fiber composition in longitudinal and cross-sectional studies. If a constant site has to be sampled, allowance has to be made for the overall size of the muscle. When comparing results in a foal with those in an adult, for example, sampling depth at the same site should be approximately 2.5 cm in the foal and approximately 5 cm in the adult. In addition, frequent sampling from the same animal also requires confidence that a similar site can be sampled. During biopsy of the middle gluteal muscle in the adult horse, most workers have adopted a site 10 to 15 cm caudodorsal from the tuber coxae and at an angle of 45 degrees at a depth of 5 to 10 cm in the adult (see Figure 12-4). Samples taken at a uniform depth and within about a 5-cm radius of this point give reproducible results with slight variation in fiber types (Snow, 1983). Although this difference may require caution when fiber typing is done in an attempt to evaluate performance potential, a relatively small difference does allow repeated sampling in short time frames, for example, in recovery studies following bouts of exercise. At a similar site, no difference exists between samples taken from the right or left middle gluteal of normal muscle. The standardized fiber type composition of the gluteal muscle varies between breeds and between individual horses and is impacted by training (Table 12-4 and Table 12-5). In addition, gender also has a significant effect on fiber composition. A higher proportion of type I fibers is found in the gluteal muscle of Andalusian stallions versus mares but this is not so in Arabians (Rivero et al., 1995). Large-scale studies of Standardbreds and Thoroughbreds have found no difference between stallions and mares in the proportion of type I fibers, but stallions have a higher ratio of type IIA to type IIB fibers beginning at 1 year of age (Roneus, 1993; Roneus et al., 1991; Roneus et al., 1992). In general, the higher the composition of type IIB fibers in a muscle biopsy, the lower is the oxidative capacity in muscle and the more is the lactate that is produced with maximal exertion such as racing (Figure 12-6 and Figure 12-7). TABLE 12–4 Fiber Composition in the Middle Gluteal of Different Breeds of Horses Adapted, with permission, from Snow DH: Skeletal muscle adaptations: a review. In Snow DH, Persson SGB, Rose RJ, editors: Equine exercise physiology, Cambridge, UK, 1983, Granta Editions, p 160.
Muscle anatomy, physiology, and adaptations to exercise and training
Muscle structure and function
Embryology
Muscle ultrastructure
Equine muscle composition: Response to exercise and adaptations with training
Percutaneous muscle biopsy
Fiber types
max) of shortening was determined (Rome et al., 1990). In contrast to small animals, where a three- to fivefold difference in
max exists between fiber types, the horse has a 10-fold difference, the type IIB fibers shortening more rapidly than would be expected from normal scaling.
max was found primarily to be associated with fiber type rather than diameter or force generation. This higher
max for type IIB fibers indicates that there is a sacrifice of efficiency for increased mechanical power, since power is higher in fibers with a high
max than in those with a lower
max.
O2max (Hoppeler et al., 1987). Type I fibers have an abundant supply of lipid droplets, whereas fewer are seen in the type II high-oxidative fibers, with low-oxidative fibers not surprisingly having negligible amounts. Glycogen is found as discrete granules throughout the sarcoplasm. With respect to the contractile properties, type II fibers, which have a greater requirement for rapid Ca2+ turnover, have a more prominent sarcoplasmic reticulum. Z- and M-band width also can vary between types. In some species, fiber typing has been carried out on the basis of these ultrastructural differences.
Fiber type populations within muscles
Stay updated, free articles. Join our Telegram channel
Full access? Get Clinical Tree
Muscle anatomy, physiology, and adaptations to exercise and training
Only gold members can continue reading. Log In or Register a > to continue
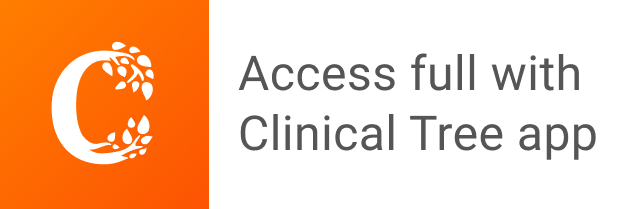