Chapter 103 The left lung of dogs and cats is divided into cranial and caudal lobes (Figure 103-1, A). The left cranial lung lobe is divided into a cranial and caudal portion but shares a common lobar bronchus. The right lung is divided into four distinct lobes: cranial, middle, caudal, and accessory (Figure 103-1, B). The accessory lobe passes dorsal to the caudal vena cava and is located medial to the plica vena cava. The trachea divides into two principle bronchi, which in turn subdivide into lobar bronchi that supply each lung lobe (Figure 103-2). Within each lung lobe, lobar bronchi divide into segmental bronchi that supply bronchopulmonary segments. Dichotomous branching of the airway continues through subsegmental bronchi, terminal bronchioles, and respiratory bronchioles. Respiratory bronchioles give rise to alveolar ducts, alveolar sacs, and pulmonary alveoli. Ventilation is the process of moving air in and out of the alveoli. It requires input from the brain respiratory centers, spinal cord, peripheral nerves, and the respiratory muscles and the presence of negative pressure in the pleural spaces, which allows coupling between the lung and the thoracic wall. Normal resting ventilation is accomplished primarily by the diaphragm during inspiration and elastic recoil during expiration.102 During inspiration, the diaphragm contracts, pulling the caudal lung surface with it. Expiration is passive, with elastic recoil of the lungs returning them to their pre-inspiration position. During exercise or when ventilatory demand is increased, external intercostal, sternocleidomastoid, scalenus, and ventral serratus muscles are used to pull the ribs in a rostral direction and pull the lungs outward. Contraction of internal intercostal muscles and abdominal rectus muscles aids during expiration.95 During inspiration, ventilation has to overcome tissue elastance, alveolar surface tension, and airway resistance.102 Elastance is the degree to which the lung can return to its dimensions (recoil) after removal of the distending forces of inspiration. It is measured in unit volume change per unit of decreased pressure. Compliance is a measure of lung distensibility and is the reciprocal of elastance; it is measured as change in lung volume per unit pressure change. Pulmonary compliance can be estimated from pressure-volume loops obtained during the respiratory cycle and is equivalent to the slope of the pressure-volume curve (ΔV/ΔP) at any particular point. The steeper the slope of a pressure-volume curve, the greater the compliance. Normally, lung compliance is lowest at high and low lung volumes; thus, more energy is required to expand the lungs by the same volume at those points. Lung compliance can be modified by fibrosis or pulmonary edema. Alveolar surface tension is generated at the air–liquid interface in the alveoli. Normally, it is low because of surfactant produced by alveolar type II cells. Surfactant indirectly increases lung compliance by lowering surface tension and reduces the amount of work needed to inflate the lungs. It also prevents collapse of small alveoli, and thus atelectasis, by permitting variation in surface tension with change in alveolar size. Airway resistance must also be overcome during inspiration and expiration. Contributors to inspiratory airway resistance during normal, quiet respiration include the nares (79% of total resistance during inspiration), larynx (6%), and small airways (15%).64,65,95 Expiratory resistance is 74% nasal, 3% laryngeal, and 23% small airways. Alveolar ventilation is precisely controlled to match metabolic need; therefore, arterial oxygen pressure (PaO2) and arterial carbon dioxide pressure (PaCO2) vary minimally with physical activity. Ventilation is controlled by a respiratory center in the medulla that generates breathing rhythm and regulates tidal volume.99,102 Chemical and neural reflexes adjust ventilation to the needs of the patient, with chemoreceptor reflexes being the most important regulators. Changes in PaO2, PaCO2, and pH stimulate central and peripheral chemoreceptors, with the greatest response to changes in carbon dioxide. A small increase in PaCO2 causes a substantial increase in ventilation, which returns PaCO2 to original levels. A normal dog or cat moves approximately 10 mL of air per kg per inspiration to have adequate ventilation. A portion of that ventilation is alveolar, which is critical for gas exchange, and the remainder is dead space, which is important for other functions such as thermoregulation. Alveolar ventilation determines the amount of CO2 in arterial blood: when PaCO2 is increased, hypoventilation is present; conversely, when PaCO2 is decreased, hyperventilation is present. Animals can change the ratio of dead space to alveolar ventilation by changing breathing patterns. Because panting dogs have a normal PaCO2, they are not hyperventilating, even though total ventilation increases dramatically, because PaCO2 is only altered by alveolar ventilation. Dead space itself can be significantly increased when alveoli are ventilated but not perfused (e.g., pulmonary thromboembolism).19 Diffusion of gas across the blood–gas interface follows Fick law, which states that rate of transfer of a gas through a sheet of tissue is proportional to surface area available for diffusion, diffusion coefficient of the gas, and difference in gas partial pressure between the two sides and inversely proportional to the tissue thickness (distance the gas must travel). At the blood–gas interface, the lungs have a surface area of 50 to 100 m2 and a 0.3-µm thickness, which is ideal for diffusion. The diffusion coefficient of CO2 is 20 times greater than that of oxygen; CO2 therefore diffuses more rapidly.100 Normally, this difference is inconsequential; however, oxygen delivery can become critical if the area for diffusion decreases and the diffusion distance increases, resulting in impaired delivery of oxygen (hypoxia) well before CO2 removal is inadequate (hypercapnia). Oxygen and CO2 are transported into the blood in different forms.101 The oxygen–Hb dissociation curve describes the interaction between oxygen and heme. The curve has a very characteristic sigmoid form that represents several physiologic advantages (Figure 103-3). The flat upper portion means that a decrease in partial pressure in alveolar gas will have little effect on oxygen saturation when oxygen partial pressure is 80 mm Hg or more. Also, because oxygen diffuses along a positive-pressure gradient from the alveoli to capillary blood, oxygen reserve is large enough for sufficient oxygen to diffuse into the blood and saturate Hb. The steep part of the dissociation curve means that the peripheral tissues can withdraw a large amount of oxygen for only a small decrease in capillary oxygen partial pressure. The oxygen dissociation curve can be shifted to the right with acidosis and increased temperature, 2,3-diphosphoglycerate in red blood cells (RBCs), and CO2 (Figure 103-4). It shifts in the opposite direction with the opposite causes. A rightward shift allows better unloading of oxygen, which is beneficial in peripheral tissue. CO2 is carried in the blood in its dissolved form (∼5% of transported arterial CO2) and in chemical combination with proteins (primarily Hb) as carbamino compounds (∼20% of excreted CO2); however, the majority is transported in the form of bicarbonate (Figure 103-5). Within RBCs, carbonic anhydrase accelerates transformation of CO2 into carbonic acid, which dissociates into bicarbonate and hydrogen ions. Whereas bicarbonate ions diffuse along a concentration gradient back into plasma, hydrogen ions are trapped in RBCs. To maintain electrical neutrality and osmotic balance, respectively, chloride ions (Cl−) and water diffuse into the cells. The reaction continues to move to the right because Hb buffers the hydrogen ion. In the lungs, CO2 is removed with ventilation, decreasing PCO2 and reversing the effect. Pulmonary gas exchange refers to the collective process by which O2 and CO2 are exchanged between the alveolus and the arterial blood.103 Exchange of O2 is complex and dependent on several factors, including diffusion across the alveolar–capillary membrane, amount of venous admixture in arterial blood, and relationship (or matching) of ventilation to perfusion. PaO2 should be nearly equal to alveolar oxygen pressure (PAO2) and should approach 100 mm Hg at sea level under normal physiologic conditions. Impaired gas exchange occurs when PaO2 becomes less than PAO2. The average alveolar partial pressure of oxygen can be calculated with the equation: PAO2 = [FIO2 × (Pbarometric − 47 mm Hg)] − [1.2 × PaCO2], with FIO2 = fraction of inspired oxygen (21% on room air) and PH2O = 47 mm Hg. The alveolar–arterial oxygen difference can be calculated to quantify the severity of the gas exchange impairment using the formula: PA-aO2 = PAO2 – PaO2 = [FIO2 × (Pbaro − 47 mm Hg) − 1.2 × PaCO2] − PaO2. In a normal animal breathing room air, PA-aO2 should be less than 10 mm Hg. If PA-aO2 is greater than 30 mm Hg, there is severe gas exchange impairment. After thoracotomy, hypoxemia, residual pneumothorax, pleural effusion, and pain must be corrected to help restore normal pulmonary and cardiac physiology.95 Atelectasis that develops during the surgical procedure contributes to hypoxemia.66 It is aggravated in the postoperative period by lateral recumbency of the patient. In lateral recumbency, the dependent lung collapses under the weight of the heart and any accumulated fluid. Ventilation is mostly distributed to the nondependent lung, and gravity distributes blood flow to the poorly ventilated dependent lung, aggravating V/Q mismatch. Patients should be turned regularly to help reduce V/Q mismatch and monitored for development of aspiration pneumonia that would contribute to V/Q mismatch. Thoracotomy is a painful procedure because of retraction applied on the thoracic wall. Pain prevents full thoracic wall excursion, which then results in reduced ventilation, contributing to hypoxemia. Pain also results in catecholamine release, which contributes to vasoconstriction, reduction of tissue perfusion, tachycardia, and arrhythmias. Pain management is therefore critical in the postoperative period. Analgesics, especially opioids, however, have a significant depressant effect on the respiratory center. Therefore, a good balance between maintenance of respiratory rate and pain management should also be achieved. Epidural analgesia has been reported as an efficient technique to control pain without interfering with ventilation.68,71 Local regional blocks, such as intercostal nerve block or intrapleural lidocaine and bupivacaine, also facilitate postoperative pain control without affecting ventilation.11,93 Short-acting opioids, such as fentanyl or fentanyl–lidocaine constant rate infusion (CRI) are recommended after surgery because the dose and rate can quickly be adjusted to the needs of the patient.90,93 The goal of the postoperative care is to optimize oxygen delivery, and because oxygen delivery is a function of cardiac output and arterial oxygen content, it is important to monitor cardiac and respiratory functions.19,66,97 Hypothermia, hypotension, hypoventilation, electrolyte imbalance, and shock are very common after thoracic surgery and need to be recognized and treated aggressively. Heart rate, arterial pressure, central venous pressure, and urine production are monitored constantly for the first 24 hours. Blood gases are performed in the postoperative period to evaluate ventilation, detect hypoxemia, and determine if the patient will need oxygen supplementation. RBC transfusions and oncotic support may be necessary in some patients to optimize tissue oxygenation and perfusion. An intercostal thoracotomy starts with an incision through the skin and subcutaneous tissue with the cutaneous trunci muscle (see Figure 104-7). As a general landmark, the caudal-dorsal tip of the scapula lies at the level of the fourth intercostal space. The latissimus dorsi muscle is incised from ventral to dorsal with cautery to minimize intraoperative bleeding. After incising the latissimus dorsi muscle, intercostal spaces can be counted from cranial to caudal by palpating under the muscle and subcutis. After the appropriate intercostal space has been identified, the serratus ventralis muscle is separated between muscle fibers. Ventrally, the scalenus muscle is transected if the thoracotomy is cranial to the fifth rib and the rectus abdominis muscle is incised if caudal to the fifth rib. To spare the intercostal artery and nerve, intercostal muscles are incised close to the cranial border of the caudal rib in the intercostal space. Pleura is punctured to allow collapse of lung lobes and entry into the pleural space. The pleural incision is extended dorsally and ventrally as needed; ventrally, the internal thoracic artery should be identified by internal palpation along the sternum so as to avoid damage. Before closing the thoracotomy, a thoracostomy drain is placed to establish negative pressure in the pleural space and monitor the pleural space in the postoperative period. To prevent air leakage in the postoperative period, the drain is tunneled underneath the latissimus dorsi muscle over two to three intercostal spaces.107 The intercostal thoracotomy is closed by placing circumcostal sutures of 1-0 absorbable material to approximate the ribs. Alternatively, holes can be drilled in the caudal rib of the intercostal space to reduce the risk of intercostal nerve compression.77 This technique has been associated with less postoperative pain; however, it can only be performed in large-breed dogs because of the risk of rib fracture.77 Each muscle layer is closed with a simple continuous suture pattern with a 3-0 absorbable monofilament suture materials. The subcutaneous tissue and skin are also closed with continuous patterns. An important technical consideration when performing a sternotomy is that the manubrium, xiphoid, or both should be left intact. Doing so greatly enhances the ability to achieve a stable sternotomy closure, thereby decreasing the risk of postoperative complications. Reluctance to use this approach because of a perception that it is associated with excessive postoperative pain or complications is not justified. In dogs, pain, the degree of cardiopulmonary impairment, and complication rates with this approach are not different from those after intercostal thoracotomy.16,75,79 In humans, median sternotomy causes less postoperative discomfort than intercostal thoracotomy.21,98 The decision to use a sternotomy or thoracotomy approach should therefore be based on the kind of access to the thoracic cavity needed, not on a perception that one approach is superior with regard to intraoperative or postoperative morbidity. For a median sternotomy, the patient is placed in dorsal recumbency, and the skin is incised on midline from the manubrium to the xiphoid. The pectoralis muscle is dissected off the midline with electrocautery until the central line of the sternum is exposed (see Figures 104-10 to 104-14). Sternotomy is performed with a sternotomy or oscillating saw. If an oscillating saw is used, intrathoracic structures should be protected with a malleable retractor. The xiphoid or manubrium is left intact. A thoracostomy drain is placed before sternotomy closure, which is accomplished with cerclage wires in a figure of eight fashion around sternebrae to provide maximum stability.14 Stainless steel is preferred over nonabsorbable sutures, especially in large-breed dogs, because sutures are not strong enough to maintain apposition of the two halves of the sternum until it is healed.69 Thoracoscopy can be performed through a transdiaphragmatic or an intercostal approach. The intercostal approach is primarily used for surgical procedures such as partial or complete lung lobectomy. The transdiaphragmatic approach is preferred for exploration of the thoracic cavity and pleural space. For an intercostal approach, the patient is positioned in lateral recumbency. Cannulas are placed as needed within the fourth to ninth intercostal spaces. Cannulas should be inserted in the ventral two thirds of the intercostal space because the ribs are stiff along the most dorsal part of the intercostal space, making manipulation of instruments and camera difficult. The skin is incised to a size equivalent to cannula diameter. The thoracic wall is bluntly dissected with a mosquito forceps until the thoracic cavity is perforated. The cannula with its blunt trocar is introduced through the incision into the thoracic cavity. Three or four trocars are usually required to perform a thoracoscopy. Biopsy of hilar lymph nodes, sternal lymph nodes, lung lobes, or the pleural surface can be collected with this approach. Lung lobectomy is performed with an intercostal thoracotomy associated with one-lung ventilation.38,39 Congenital Diseases of the Lungs Congenital disease of the lungs is either compatible or incompatible with life;45 severe anomalies of agenesis or hypoplasia cause death shortly after birth. Hypoplasia of one lung or lung lobe is rarely diagnosed unless thoracic radiographs are taken or a necropsy is performed.80 Congenital tracheoesophageal and bronchoesophageal fistulas are extremely rare in dogs and cats.6,31,77 Cystic and bullous lesions in the lungs are characterized by a thin-walled cavity within the lung parenchyma.2,45,60 Cysts can be fluid filled or air filled and are covered by a respiratory epithelium.4,60 Blunt trauma to the chest with pulmonary contusion seems to be the most common cause of pulmonary cysts in dogs and cats, and younger animals appear to be at greater risk.4 An infected cyst will become a pneumatocele or an abscess, with destruction of respiratory epithelium. Pneumatocele can also result from pneumonia.60 Lung bullae and blebs (pseudocysts) are similar to cysts; however, they have no epithelial lining.7,34,35,60 Bullae and blebs are described as large blisters with fibrous walls. Bullae are large air spaces that develop within the lung parenchyma, and blebs are small accumulations of air between the parenchyma and visceral pleura (Figure 103-6).2 These cavities develop from traumatic rupture and coalescence of alveoli and are frequently secondary to obstructive lung disease.34,35,42,45,60 Pulmonary bullae have been reported in 16% of dogs with pulmonary blastomycosis, most often in association with an alveolar pattern.12 Bullae and cysts show similar complications of infection: abscessation; rupture, resulting in pneumothorax; and local compression of lung tissue, leading to dyspnea, exercise intolerance, and abdominal respiration.60 Auscultation may reveal decreased ventilation sounds on the affected side and increased heart sounds on the contralateral side (displaced heart from a space-occupying cyst). Spontaneous idiopathic pneumothorax warrants close examination of radiographs detailing lung profile and parenchyma for bleb, bullae, or cyst formation.42,72 Radiographs usually reveal unilateral or bilateral pneumothorax and occasionally pneumomediastinum; unfortunately, however, bullae or blebs are usually not identified.42 Computed tomography (CT) has been recommended for the diagnosis of bullae in dogs with spontaneous pneumothorax.5,30,41 Atelectasis of the lobe containing the ruptured cavity may obscure underlying cysts. Conservative management of spontaneous pneumothorax by continuous intrathoracic drainage can be attempted for 2 to 3 days before partial or complete lobectomy is considered.27,42,72 Many patients improve with conservative therapy, but recurrence rate is high.72 In a study of 12 dogs, none responded to conservative treatment with thoracocentesis or thoracostomy tube placement. Lung lobectomy is the treatment of choice for spontaneous pneumothorax in dogs.42 The preferred approach is a median sternotomy because dogs commonly have more than one lesion, and many lesions are bilateral.42 Most dogs have lesions in the cranial lung lobes, but other lobes can be affected concurrently. If the bulla or bleb cannot be visualized, the thorax is filled with warm saline so that leaks can be identified during ventilation. Visible lesions are removed by partial or complete lung lobectomy, often with a stapler. Resected tissues should be submitted for culture and histologic evaluation if underlying conditions are suspected. In one study, recurrence was not reported in 12 of 12 dogs after partial or complete lobectomies for bullae and blebs.42 Thoracoscopy has been used to evaluate visceral surfaces for additional cysts and locating the ruptured cavity;8 however, thorough evaluation of the entire lung surface can be difficult with this modality. Mechanical pleurodesis can be attempted at the time of surgery to induce complete pleural adhesion and reduce the risk of recurrence; unfortunately, successful production of a complete adhesion for control of the disease is uncommon in dogs.29
Lungs
Anatomy
Lungs
Pulmonary Physiology
Gas Diffusion Across the Blood–Gas Interface
Gas Transport by Blood
Gas Exchange
Consequences of Thoracotomy on Pulmonary Physiology
Hypoxemia
Pain
Postoperative Monitoring
Surgical Approaches
Median Sternotomy
Thoracoscopy
Specific Conditions
Cysts, Bullae, and Blebs
Stay updated, free articles. Join our Telegram channel
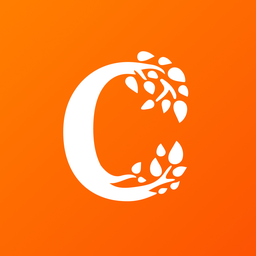
Full access? Get Clinical Tree
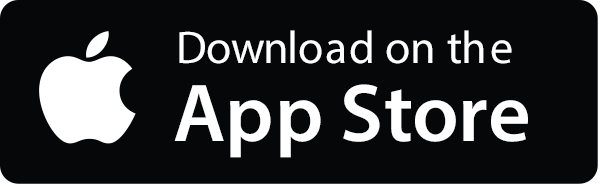
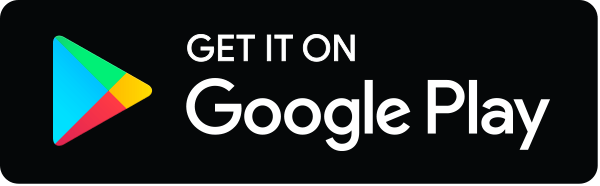