Chapter 4 However, detailed knowledge is still lacking on the neuroanatomical and neurophysiological basis of locomotion in horses. Investigations into such neurobiological aspects generally require invasive procedures, and ethical considerations, the economical value and the size of this animal are the main reasons for a paucity of data. Non-invasive techniques such as transcranial magnetic stimulation with the recording of responses in the EMG or fMRI-techniques have not yet been adapted to equine research for a variety of reasons. On the other hand, research into the biomechanical aspects of walking, galloping and trotting in horses, and also into gait analysis with high-speed filming, have a long tradition. Results from this research have set standards which, in turn, inspired investigations in man and smaller laboratory animals. The ‘golden age’ of research into equine locomotion in the 1960s, (Van Weeren, 2001) developed a promising sequel by research in several laboratories in the USA, in Canada as well as in several European countries, most notably in The Netherlands and in France. The first edition of Equine Locomotion marked this interest and the results obtained so far were comprehensively summarized there. Concepts on the neurobiological basis of locomotor movements in horses, therefore, necessarily mainly rely upon knowledge obtained in laboratory animals. Older textbooks on comparative neuroanatomy include only a few data on horses. These textbooks are mainly limited to comparing the gross anatomy of the brains in smaller mammals and man, with that of primitive vertebrates such as teleosts, reptiles, amphibians and birds. Larger mammals are rarely included in these comparisons (e.g. Ariens Kappers et al., 1967). Also, data on neural connections in the horse’s brain are scarce. Verhaart and Sopers-Jurgens (1957) described Bagley’s bundle by dissection of the horse’s brain. This projection runs from the cerebral cortex to the mesencephalic and pontine tegmentum and is considered to be the phylogenetic predecessor of the corticospinal tract (CST) which is present in other mammals. In the last four or three decades of the 20th century a variety of neuroanatomical tracing techniques have been developed and these opened a multitude of new insights into brain circuitry. By the injection of retrogradely or anterogradely transported vital stains, terminals of neural projections or their site of origin can be studied. Such investigations, however, require surgical interference as well as, after a short survival period, post-mortem processing of the brain tissue. These studies have therefore not been performed in horses. For similar reasons, studies involving the implantation of depth electrodes for recording or stimulating central nervous structures have not been carried out in horses. Modern imaging techniques of the brain (as fMRI) have been widely applied in human research and results may indicate the brain areas involved in specific tasks. These studies require immobility of the skull in the scanner and that is one of the reasons why these techniques do not lend themselves to investigations in horses. Possibly, the newest advance in brain-imaging, MRI-diffusion tractography, which allows non-invasive identification of tracts in the CNS, might ultimately be applied to study neural projections in animals and possibly in larger animals (Behrens et al., 2007). The relationships between the terminal projection patterns of the CST in a variety of animals in relation to their manipulative skills, is a case in point (Armand, 1982). In higher primates, the pyramidal neurons in the primary motor cortex are monosynaptically connected to the motoneurons of the hand and wrist muscles, located in the cervical spinal cord. By virtue of these direct connections, apes and man are able to independently move their fingers and toes, to manipulate objects and to use tools, while standing upright (Lawrence & Hopkins, 1976). In cats, however, fibers of the CST mainly end in the dorsal and medial laminae of the spinal cord and they connect to the so-called premotor neurons at cervical and lumbar levels. Indeed, cats cannot move their toes independently. Rats take an intermediate position between primates and cats as these animals do have a limited number of direct connections between cortical and spinal motoneurons and these do allow them to manipulate their food by independent toe movements. In rabbits and goats the CST only descends as far as the upper cervical segments and its fibers do not even reach the premotor neurones of the cervical and lumbar spinal cord. Horses most probably are similar to these animal species with respect to the descent of the CST or its analogon (Armand, 1982; Verhaart & Sopers-Jurgens, 1957). Leg and trunk movements are produced by skeletal muscles. Histological and neurophysiological aspects of muscles at adult age have been reviewed several times (Burke, 1981; Partridge & Benton, 1981; Kernell, 1998) and a few of these will be repeated only briefly here. The different types of muscle fibers can be visualized histologically. Post-mortem studies allow the investigation of the distribution of fiber types in sections taken perpendicular to the longitudinal axis of the muscle at several levels. Multiple biopsies only allow an approximation of such data. In a classical method of processing (Dubowitz & Brooke, 1973), the muscle material is preincubated at different pHs and the ATPase staining technique then enables differentiation of the muscle fiber types. More recent methods apply myosin-related antibodies to identify several subtypes of muscle fibers (Schiaffino et al., 1986). Investigations with these methods have demonstrated that all muscles consist of a mixture of type I, type IIa and type IIb muscle fibers, but the relative proportions of these fibers differ greatly. Extremity muscles and particularly the flexor muscles may contain higher proportions of type IIa and IIb fibers and fewer type I muscle fibers. The antigravity muscles in the extremities, in contrast, contain increased proportions of types I and IIa muscle fibers. This is understandable when realizing that these muscles have to carry the weight of the animal for long periods of time. The proportions of type I muscle fibers are particularly pronounced in the soleus muscle in the hind legs, and portions of the flexor carpi ulnaris muscle in the forelegs. In these muscles, the type I muscles fibers may be as high as 100% (e.g. in rats). It is noteworthy that the type I fibers both in the extensors and the flexor-muscles are mostly concentrated in the deeper portions of the muscles, close to the skeletal elements (Wang & Kernell, 2001). Similar data have been obtained in horses (Grotmol et al., 2002). The trunk muscles also contain elevated percentages of type I and IIa muscle fibers. This holds particularly for the multifidus and the longissimus muscles in the back (Gramsbergen et al., 1996). Similar results have been obtained in horses (Gellman et al., 2002). The high percentage of type I and IIa muscle fibers make these particular muscles fatigue resistant and therefore these muscles are ideally suited for postural functions, such as keeping the trunk straight and carrying the body weight (for a review on regional differences in the distribution of type I and type II muscle fibers, see Kernell (1998)). Horse breeds differ in the size and composition of their muscles. Horses of some breeds are heavily built with bulky muscles that have a large cross-sectional area and these horses are kept in order to sustain enduring agricultural or transportation tasks. Their muscles contain large proportions of type I and type IIa muscle fibers which are able to produce forces for long-lasting periods (see Miyata et al., 1999). Horses of other breeds are characterized by a slender and athletic build that is appropriate for speed racing. Their muscles have increased proportions of type II muscle fibers that allow them to produce immense forces but only for relatively short periods of time. Investigations in man with EMG recordings and, in addition, power spectral analysis of the EMG and the calculation of EMG-fatigue indices, indicated that loading for long periods induces the recruitment of satellite cells in the muscle (see below), their development into muscle cells and a relative increase in type II muscle fibers (Mileva et al., 2009). Investigations, e.g. in rats, have shown that per individual muscle the number of type I muscle fibers remains unchanged (or, is genetically determined; see Wilson et al., 1988; see also, Mileva et al., 2009) and Mileva’s results, therefore, point towards an absolute increase in type II fibers. Other investigators suggest, in contrast, that training may influence the fiber type distribution of muscles by shifts in the numbers of both type I and type II fibers (e.g. Duclay et al., 2009). Other training effects are hypertrophy of muscle fibers as indicated by an increase in their cross sectional area, increases in mitochondrial content (important for aerobic glycolysis) (Grünheid et al., 2009), changes in muscular architecture (which might be secondary to the muscle fiber hypertrophy) (Duclay et al., 2009), as well as vascular changes by angiogenesis and an increase in arteriolar density (for review, Laughlin and Roseguini (2008)). Short-term effects of training aim at increasing the storage of large quantities of glycogen for rapid use in type II muscle fibers. Muscle cells or myocytes develop by the fusion of a longitudinal array of mesenchymal cells. The first generation of these multinuclear myoblasts, extending from tendon to tendon, are the primary myotubes. Each of these is soon innervated by a multitude of motoneuronal axons. At a later stage, a second generation of secondary myotubes develops in the vicinity of the middle region of the primaries. The secondary myotubes also are initially innervated by multiple motoneuronal axons (Ontell & Dunn, 1978; Goldspink & Ward, 1979; Bennett, 1983). During further development, the contractile proteins actin and myosin increase enormously in the myotubes of both types. The motor endplate develops its folded appearance and from a certain stage of development these cells have acquired the characteristics of the mature myocyte. It is generally accepted that the primary myotubes are the precursors of type I muscle fibers and the secondary myotubes of the type II muscle fibers (see Wilson et al., 1988). Wilson and coworkers studied in rats the effects of severe under-nutrition from early gestation and through the lactation period on muscular development. The number of primary myotubes remained unaffected but they detected a dramatic decrease in the number of secondary myotubes. These and other results has led them to hypothesize that the development of primary myotubes is genetically determined while that of the secondary myotubes is susceptible to environmental factors, such as under-nutrition, hormonal factors and also training (Wilson et al., 1988). In addition, non-differentiated satellite cells develop and these cells may differentiate at a later stage into myocytes after damage to the muscle or after training. Research mainly in cats, has indicated that the small-sized motoneurons innervate the type I muscles fibers and in neurophysiological research, these units have been coined ‘S-type units’ (slow-twitch, fatigue-resistant). Motoneurons of intermediate sizes innervate type IIa muscle fibers and physiologists have distinguished ‘FR-type units’, fast-twitch, fatigue-resistant units and ‘Fint-type units’, with fast-twitch and intermediate fatigue-resistant units. The largest motoneurons are part of the ‘FF-type units’, innervating type IIb muscle fibers; these units are fast-twitch and fatigable (Burke, 1981). The motorunits systematically differ in the numbers of muscle fibers which are innervated by the motoneuron. The smaller motoneurons innervate relatively small numbers of type I muscle fibers. Both the low tension produced by each of these fibers and the relatively small number of muscle fibers in each of these units, explain why these units produce relatively low forces. The larger motoneurons innervate larger numbers of (type II) muscle fibers. Each of these fibers produce higher tensions, and consequently these units produce relatively large forces (for a review see Kernell, 2006). At early stages of development, muscle cells are innervated by more than one axon (see above) and this is known as polyneural innervation. Later, and already in early development, this multiple innervation of muscle cells is replaced by mononeural innervation (for reviews, Bennett, 1983; Jansen & Fladby, 1990). Research from many groups has indicated that this regressive process is near completion when the behavioral function in which the muscle is involved has developed (Gramsbergen et al., 1997). Rats are a precocious species and they are born at an early stage of brain development. Still, mononeural innervation of respiratory muscles in this species has already been reached at birth when the animals start breathing continuously. On the other hand, in the psoas muscle in the hind leg, this regression of polyneural innervation is only completed around the 16th postnatal day (IJkema-Paasen & Gramsbergen, 1998). This is precisely the point at which they start the adult-like pattern of walking. Horses are a precocious species, born with a nervous system at an advanced stage of development. They are able to stand and walk shortly after their birth and, therefore, it is safe to postulate that most of their muscles are mononeurally innervated from birth. It has been suggested that the great excess of axons innervating the primitive muscle fibers at early stages and the selective regression of supernumerary nerve fiber-endings thereafter plays a role in matching the properties of muscle fibers to those of the motoneurons (O’Brien et al., 1978; Greensmith & Vrbova, 1991). More recent research has indicated, however, that even at the earliest stages ingrowing axons follow a trajectory towards a specific muscular region with a preponderance of particular muscle fiber types (Wang et al., 2002). Therefore, the polyneural innervation at first and its regression thereafter should rather be regarded as a fine-tuning in the matching-process. The motoneurons within a motoneuronal pool differ in their sizes (see above). Henneman & Mendell (1981) have demonstrated that within a motoneuronal pool, the motoneurons are recruited in an orderly fashion from small to large, with increasing intensities of neuronal drive (e.g. from higher brain centers). During motor tasks which require only low muscle forces only the smallest motoneurons are activated (these motorneurons innervate groups of type I-muscle fibers). With increasing forces, additional motoneurones with larger sizes are recruited, first those with FR properties, later those with Fint properties and finally the FF units are activated with their large numbers of type IIb muscle fibers. This orderly recruitment of motoneurons is related to differences in the membrane properties of the smaller and larger motoneurons. Interestingly, the relative force-increase by recruiting additional motor units at adult age is more or less the same over a wide range of forces (Milner-Brown et al., 1973) and this leads to a smooth increase of force with increasing intensities of neuronal drive. Apart from their size, motoneurons also differ in the morphology of their dendritic tree. The motoneurons in particular pools have their dendrites running in bundles both in a longitudinal direction as well as in transverse directions, while in other motoneuronal pools such organization is absent. Motoneurons with dendrite bundles have been described in cats (Scheibel & Scheibel, 1970), in rats (Gramsbergen et al., 1996) and in the human spinal cord (Schoenen, 1982) and because of their wide occurrence it seems highly likely that dendrite bundles also occur in the horse’s spinal cord. Dendrite bundles consist of up to 15 dendrites in close vicinity for several hundreds of millimeters (Fig. 4.1), and connected via long-stretched gap-junctions (Matthews et al., 1971; van der Want et al., 1998
Locomotor neurobiology and development
Introduction
Muscles, motors of movements
Muscle fiber-types
Muscular development
The motor unit and motoneurones
Development of muscular innervation
Size principle
Dendrite bundles
Stay updated, free articles. Join our Telegram channel
Full access? Get Clinical Tree
Locomotor neurobiology and development
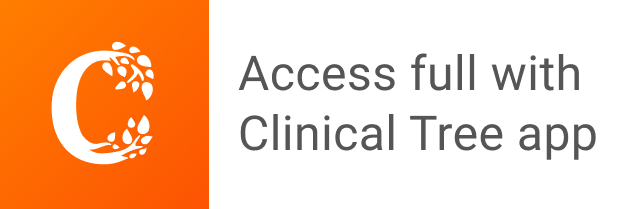