Chapter 4 Lipids and Ketones A. Structure, Properties, and Assay of Long Chain Fatty Acids B. Synthesis of Long Chain Fatty Acids C. Catabolism of Long Chain Fatty Acids A. Structure, Properties, and Assay of Triacylglycerol B. Synthesis of Triacylglycerol C. Catabolism of Triacylglycerol A. Structure and Properties of Phospholipids C. Catabolism of Phospholipids A. Structure, Properties, and Assay of Cholesterol A. Structure, Properties, and Assay of Lipoproteins C. Digestion of Fat and Formation of Chylomicrons D. Very Low Density Lipoprotein: Synthesis, Export, and Metabolism E. Metabolism of High-Density Lipoproteins B. Canine Fasting Hyperlipidemias C. Feline Fasting Hyperlipidemias D. Equine Fasting Hyperlipidemia E. Pathophysiology of Ketonemia H. Ketosis Associated with Pregnancy and Lactation This chapter covers the biochemistry and clinical chemistry of long chain fatty acids, triacylglycerols, phospholipids, cholesterol, and ketones, a list that includes the majority of lipids found in vertebrates. The only remaining major classes are sphingolipids and waxes, which are not discussed here. Although lipids have many functions, two of the most important are energy storage and membrane structure. Triacylglycerols are by far the most important lipid with regard to energy storage, and phospholipids and cholesterol are the most important lipid membrane constituents. Lipids serve other functions, including being precursors for steroids and bile acids (cholesterol), thermal insulation (triacylglycerols), and electrical insulation (various lipids). Virtually all lipids are insoluble in water, which greatly complicates their handling in the body. Because of their insolubility, lipids must rely on proteins for transport for any significant distance in the body, and various proteins have evolved to provide this function. The insolubility of lipids is an asset as well as a liability. Because of their insolubility, lipids generate no osmotic force, so large amounts of triacylglycerol can be stored in adipose without the weight gain from water that would accompany it if it were soluble. The insolubility of lipids is vital to many of their functions in membranes. Long chain fatty acids (LCFA), frequently called free fatty acids or nonesterified fatty acids, are straight chain fatty acids containing twelve or more carbon atoms. Because LCFA are usually synthesized in animals or plants from acetyl-CoA and are then degraded two carbons at a time via β-oxidation in animals, the LCFA found most commonly in animals have an even number of carbon atoms. LCFA having carbon chain lengths of 16 and 18 constitute the greatest bulk of fatty acids in animal tissues and most animal diets. The saturated 16-carbon LCFA is palmitic acid, and the saturated 18-carbon LCFA is stearic acid. Unsaturated 18-carbon LCFA are common, with double bonds occurring at C9—C10 (oleic acid); at C9—C10 and C12—C13 (linoleic acid); and at C9—C10, C12—C13, and C15—C16 (linolenic acid). The double bonds found in fatty acids in nature are mostly of the cis configuration. Ruminant fat contains more trans-LCFA than that of nonruminants because rumen microbes isomerize some plant cis-LCFA to trans isomers. Unsaturated LCFA have a lower melting point than saturated LCFA with the same number of carbons and are more susceptible to spontaneous oxidation (Gurr et al., 2002). The 20-carbon polyunsaturated fatty acids, arachidonic acid (double bonds at C5—C6, C8—C9, C11—C12, C14—C15) and eicosapentaenoic acid (also called timnodonic acid), which is arachidonic acid with an additional double bond at C17—C18, are the precursors of the eicosanoids (prostaglandins, leukotrienes, thromboxanes). Long chain fatty acids are relatively insoluble in water at physiological pH. They dissolve readily in highly alkaline solutions, forming soaps. LCFA are amphiphilic, being quite polar (hydrophilic) at their carboxyl end and quite nonpolar (hydrophobic) at the methyl end. All LCFA must bind to proteins in order to be transported for any significant distance, and albumin is the primary transport protein in plasma (Gurr et al., 2002). Plasma LCFA concentrations can be determined spectrophotometrically with a specific enzymatic reaction, which involves direct reaction of plasma LCFA to form LCFA-CoA. Then, LCFA-CoA is oxidized using LCFA-CoA oxidase, which produces hydrogen peroxide. The hydrogen peroxide is used to produce a colored product under the catalysis of peroxidase (Demacker et al., 1982; Shimizu et al., 1980). If a sample contains triacylglycerol and lipase, which is not uncommon, LCFA may be released if the sample is allowed to stand. Falsely high LCFA may be avoided by centrifuging blood samples and freezing the plasma immediately after collection or by adding paraoxon, a lipase inhibitor (Degen and Van der Vies, 1985). LCFA may be synthesized in most tissues, but only liver, adipose, or mammary tissue does it on a large scale. Synthesis occurs in the cytosol from acetyl-CoA. The precursor of the acetyl-CoA used for LCFA synthesis is usually acetate or glucose, with the former being important in ruminants and the latter being important in nonruminant mammals. When acetate is the acetyl-CoA precursor, it is formed from plasma acetate in the cytosol, the same cellular location as the enzymatic machinery needed to manufacture the LCFA. However, when glucose is the precursor, it must go through glycolysis, which has its terminal enzyme, pyruvate dehydrogenase, located in the mitochondria. Thus, the acetyl-CoA is produced in the mitochondria, which is a problem if it is to be used for LCFA synthesis because the inner mitochondrial membrane is relatively impermeable to acetyl-CoA (Rangan and Smith, 2002). This problem has been solved by a mechanism known as the citrate shuttle, which is shown in Figure 4-1. Acetyl-CoA in the mitochondria combines with oxaloacetate under the catalysis of citrate synthase to form citrate. Citrate is translocated across the mitochondrial membrane where it is cleaved into acetyl-CoA and oxaloacetate by ATP-citrate lyase. Thus, acetyl-CoA has been effectively transported from mitochondrion to cytosol. What remains is for the oxaloacetate to reenter the mitochondria to complete the cycle. However, the inner mitochondrial membrane is also impermeable to oxaloacetate, so it is first converted to malate-by-malate dehydrogenase or aspartate-by-aspartate aminotransferase in the cytosol. The malate or aspartate is translocated into the mitochondrion where it can be converted back to oxaloacetate by reversal of the reactions that occurred in the cytosol. Alternately, malate in the cytosol can be converted to pyruvate by malic enzyme, and the pyruvate can enter the mitochondrion and be converted to oxaloacetate by pyruvate carboxylase (Rangan and Smith, 2002). Once acetyl-CoA reaches or has been formed in the cytosol, it must be carboxylated to produce malonyl-CoA via acetyl-CoA carboxylase if it is to be used for LCFA synthesis. This biotin-containing enzyme catalyzes the following reaction: Acetyl-CoA Carboxylase Acetyl-CoA carboxylase is the main regulatory site in the synthesis of LCFA, which makes sense because the cell has little use for malonyl-CoA other than the synthesis of LCFA. The enzyme is activated by citrate, which is logical because citrate will be abundant only when there is a plentiful supply of mitochondrial acetyl-CoA. In addition, acetyl-CoA carboxylase is directly inhibited by LCFA-CoA, which can be derived from the synthetic process itself or from uptake and activation of plasma LCFA. Acetyl-CoA carboxylase is also regulated by hormones via phosphorylation of the enzyme itself. Glucagon and LCFA-CoA stimulate phosphorylation, which inhibits the enzyme. Insulin activates the enzyme quickly by stimulating dephosphorylation (Rangan and Smith, 2002). These controls make sense in that a fasting or exercising animal will have its capacity for LCFA synthesis suppressed by increased plasma glucagon and LCFA levels, decreased plasma insulin, and increased intracellular LCFA-CoA. Conversely, in a recently fed animal, these controls will all be reversed to promote LCFA synthesis. Malonyl-CoA is used as the building block for LCFA in the cytosol by a large, complex, multiunit enzyme called fatty acid synthase. Fatty acid synthase uses malonyl-CoA to add two carbon units at a time to a growing LCFA chain that is attached to the enzyme itself, and it uses NADPH to reduce the oxygen that was attached to what was the end carbon of the old LCFA chain. The reaction proceeds in a series of distinct steps, which all occur on the same enzyme complex. The overall reaction is as follows: FIGURE 4-1 Fatty acid synthesis. Acetyl-CoA is generated in the mitochondria from pyruvate but cannot penetrate the mitochondrial membrane to reach fatty acid synthesizing enzymes in the cytosol. Citrate is formed from acetyl-CoA and oxaloacetate and migrates to the cytosol where it is cleaved to regenerate acetate and oxaloacetate. The acetyl-CoA is converted into malonyl-CoA and used for fatty acid synthesis. The oxaloacetate cannot penetrate the mitochondrial membrane but must be converted to malate or pyruvate, which can penetrate the membrane and be converted back to oxaloacetate in the mitochondria. NADPH needed for fatty acid synthesis is generated by the pentose phosphate pathway and malic enzyme. The subscript, n, in the structural formula for the growing LCFA is an even number ranging from zero (i.e., the starting acetyl group) to usually no more than eight (stearate). The process begins when an acetyl group binds to the enzyme complex and usually ends when a palmityl (16-carbon) group has been formed on the enzyme, at which point the LCFA is detached from the enzyme. New carbons are added to the carboxyl end, not the methyl end, of the growing LCFA. The carbon atom in the carbon dioxide produced in the fatty acid synthase reaction is the same carbon atom in the carbon dioxide used to form malonyl-CoA from acetyl-CoA. Cellular synthesis of the enzymes directly involved in LCFA synthesis (acetyl-CoA carboxylase and fatty acid synthase) and the enzymes involved in the generation of NADPH and acetyl-CoA translocation is stimulated by diets that are high in carbohydrate and low in fat and suppressed by fasting, high-fat/low-carbohydrate diets, and diabetes. These changes appear to be brought about, in part, by alterations in plasma insulin and glucagon that accompany diet changes or diabetes (Gurr et al., 2002; Rangan and Smith, 2002). Fatty acid synthesis is expensive energetically. To add a single acetyl-CoA to a growing LCFA chain, one ATP is used directly and six more are used indirectly (each of the two NADPH is equivalent to three ATP). Because fatty acid synthesis occurs in the cytosol and requires NADPH, there must be a generous source of that cofactor when fatty acid synthesis is active. The main source of NADPH for fatty acid synthesis is the hexose monophosphate pathway in the cytosol. This pathway utilizes plasma glucose in the case of adipose or mammary tissue, whereas in the liver, it can use plasma glucose, glycogen, or gluconeogenesis as the hexose source. Another source of NADPH in the cytosol is the malic enzyme reaction. These sources of NADPH are illustrated in Figure 4-1. Although the most common length for nascent LCFA when they are released from fatty acid synthase is 16 carbons, they can be 18 carbons or, in the case of fat synthesis in the mammary gland, as short as 4 carbons. When LCFA are detached from fatty acid synthase, they are rapidly thioesterified to CoA by LCFA-CoA synthetase, an enzyme found in the endoplasmic reticulum and outer mitochondrial membrane. Most of the palmitate produced by fatty acid synthase will be elongated to produce stearate by fatty acid elongase, an enzyme found mainly in the endoplasmic reticulum but also in mitochondria. This enzyme adds 2 new carbons at the carboxyl end of the existing LCFA. Fatty acid elongase uses the same substrates (malonyl-CoA and NADPH) as fatty acid synthase, but it is located in a different part of the cell and prefers palmityl-CoA as its substrate. However, fatty acid elongase can use longer LCFA-CoA as substrates to a limited degree to produce LCFA-CoA with a length of as great as 24 carbons (Cook and McMaster, 2002). Nonruminant mammals synthesize LCFA in liver, adipose, and mammary tissue. Ruminants synthesize LCFA primarily in adipose and mammary tissue with acetate being the most important precursor. Ruminants generally have a low capacity for LCFA synthesis in liver, but after eating large amounts of high-starch diets, they may synthesize some LCFA in the liver from acetate and propionate (Hanson and Ballard, 1967; Ingle, 1972a, 1972b; Liepa 1978). Most animals are capable of desaturating LCFA only at the Δ4, Δ5, Δ6, and Δ9 positions (counting from the carboxyl end). Animals are able to desaturate palmityl-CoA and stearyl-CoA between C9 and C10 by means of Δ9 desaturase system located in the endoplasmic reticulum to produce palmitoleyl-CoA and oleyl-CoA, respectively. However, animals are not able to create additional double bonds beyond C9 in these products to any significant extent, so linoleic and linolenic acids must be absorbed from the intestinal tract (Cook and McMaster, 2002). By a combination of the actions LCFA elongase and Δ4, Δ5, and Δ6 desaturase systems, the livers of most mammals can synthesize arachidonic acid and eicosapentaenoic acid from linoleic and linolenic acids, respectively. However, the cat has very low levels of Δ6 desaturase in its liver and must have arachidonic acid in its diet (MacDonald et al., 1984). The main catabolic route for LCFA is β-oxidation. Most tissues can perform β-oxidation (erythrocytes are an exception), but those most adept at it are liver, skeletal muscle, and heart. In addition, the liver can partially oxidize LCFA to ketones, an important process that will be discussed extensively later. Before LCFA can be subjected to β-oxidation, they must be esterified to CoA, which is accomplished by the following reaction: The reaction is catalyzed by LCFA-CoA synthetase, an enzyme bound to the endoplasmic reticulum and the outer mitochondrial membrane. The pyrophosphate (PP) is rapidly hydrolyzed, so the reaction effectively consumes two ATP. The activation of LCFA is not rate limiting for β-oxidation (Pande, 1971). For LCFA-CoA to be catabolized, it must pass into the mitochondrion, which is a problem because the inner mitochondrial membrane is impermeable to it. The CoA must be exchanged for a carnitine moiety, a reaction catalyzed outside the mitochondrion by carnitine acyltransferase I (Fig. 4-2): LCFA-carnitine passes readily through the inner mitochondrial membrane and is acted on by carnitine acyltransferase II, which converts the LCFA-carnitine back to LCFA-CoA (Kopec and Fritz, 1973). Carnitine acyltransferase I appears to be controlled by inhibition by malonyl-CoA (McGarry et al., 1977), and it is logical that when lipogenesis is stimulated, the LCFA that are produced should be prevented from entering the mitochondrion where they will be catabolized. In the mitochondrion, the process of β-oxidation per se cleaves the LCFA into acetyl-CoA units. The reaction sequence is as follows: The resulting acyl-CoA is two carbons shorter and can recycle through the pathway. Each trip of an acyl-CoA through the pathway generates one FADH2 and one NADH + H1, which can generate 5 ATP via oxidative phosphorylation. If the LCFA has an odd number of carbons, which is rare, the final product of β-oxidation will be propionyl-CoA. The double bond produced by the acyl-CoA dehydrogenase reaction is of trans configuration, not the cis configuration occurring in unsaturated LCFA found free or esterified to glycerol. Unsaturated LCFA can proceed through β-oxidation to within three carbons of the double bond. As this point, Δ2-enoyl-CoA hydratase cannot act because it requires a trans, rather than a cis, configuration in its substrates, and it requires that the double bond be between C2 and C3 rather than between C3 and C4. At this point, Δ3, Δ2-enoyl-CoA isomerase will convert the Δ3-cis double bond to a Δ2-trans double bond, which will allow β-oxidation to proceed (Kilponen et al., 1991). Polyunsaturated LCFA require an additional enzyme, 2,4-dienoyl-CoA reductase, because after enoyl-CoA isomerase acts, the new trans double bond will still have the second cis double bond in close proximity, which will prevent Δ2-enoyl-CoA hydratase from acting. 2,4-Dienoyl-CoA reductase effectively eliminates the second double bond by reducing it with NADPH (Roe et al., 1990). FIGURE 4-2 Long chain fatty acid (LCFA) oxidation and ketogenesis in the liver. Abbreviations: CAT, carnitine acyltransferase. β-Oxidation in the mitochondria appears to be controlled mainly by substrate availability. The acetyl-CoA units can be oxidized in the citric acid cycle provided there is sufficient oxaloacetate to condense with them to form citrate. Alternatively, acetyl-CoA units can be recondensed to form ketones, which will occur when there is not sufficient oxaloacetate for citrate formation or when citrate synthase is inhibited by high levels of citrate. Although β-oxidation occurs mostly in mitochondria, the process occurs to a minor extent in peroxisomes as well (Wanders et al., 1992). Although the main catabolic route for LCFA is β-oxidation, there are two quantitatively minor alternatives. α-Oxidation, in which carbons are removed one at a time from the carboxyl end of the LCFA, is used by brain tissue to produce LCFA of varying lengths for synthesis of complex lipids. ω-Oxidation, which is oxidation that occurs at the methyl, rather than at the carboxyl, end is conducted to a limited extent by the cytochrome P-450 system in the endoplasmic reticulum of liver. The resulting dicarboxylic acid can then undergo β-oxidation to a chain length of six carbons (adipate), most of which will be excreted in the urine (Gurr et al., 2002; Mortensen, 1990). The main storage forms of LCFA are the triacylglycerols (also called triglycerides), in which three LCFA are esterified to glycerol. Triacylglycerols are even less soluble than LCFA and also must be bound to proteins in complexes called lipoproteins for transport through plasma. Assay of triacylglycerol in plasma or serum is best accomplished by enzymatic hydrolysis using lipase followed by enzymatic determination of the released glycerol (Klotzsch and McNamara, 1990; McGowan et al., 1983). If high plasma glycerol levels are likely, as it is in animals that have not eaten lately, a plasma blank must be run. Older methods that use alkaline hydrolysis require caustic reagents, consume more time, and may assay phospholipids plus triacylglycerol. Contamination of samples with glycerol, which is sometimes used to lubricate stoppers of blood collecting tubes, or with soap, which may contain glycerol or fatty acids, will lead to falsely elevated values. If the sample contains lipase, which is not uncommon, triacylglycerol levels will decrease if it is allowed to stand. Prompt centrifugation of blood samples followed by rapid analysis or freezing of the plasma will prevent falsely low triacylglycerol levels. Although most cells can synthesize triacylglycerols, liver, adipose, mammary gland, and small intestine are particularly adept at it. LCFA-CoA are the building blocks for triacylglycerol synthesis, and it should be realized that there are two sources of LCFA-CoA for triacylglycerol synthesis: LCFA in the plasma and LCFA synthesized locally. Generally, physiological or pathological circumstances, such as starvation or diabetes, which promote high plasma levels of LCFA, suppress LCFA synthesis. Physiological circumstances that promote LCFA synthesis, such as eating a carbohydrate meal, also inhibit lipolysis in adipose, so plasma LCFA levels are not elevated. To form triacylglycerols, LCFA-CoA are esterified to glycerol-3-P. Glycerol-3-P can be produced in the liver from glycerol, which is absorbed from the plasma, and ATP in a reaction catalyzed by glycerol kinase: Glycerol is normally plentiful in plasma only when there is active lipolysis occurring in adipose tissue. When glucose is plentiful in the plasma and LCFA are being synthesized from glucose via acetyl-CoA, glycerol-3-P is also synthesized from glucose in liver, mammary gland, and adipose. This process occurs via glycolysis to dihydroxyacetone-P followed by a reduction catalyzed by glycerol-3-P dehydrogenase: LCFA-CoA is esterified to glycerol-3-P by glycerol-P acyltransferase: This reaction occurs in both mitochondria and smooth endoplasmic reticulum, but the smooth endoplasmic reticulum enzyme is more plentiful and most important in triacylglycerol synthesis. Next, another LCFA-CoA is esterified by the enzyme, acylglycerol-P acyltransferase, which is located in the smooth endoplasmic reticulum: Phosphatidate (the ionized form of phosphatidic acid) is 1,2-diacyl-glycerol-3-P. Next, the phosphate is hydrolyzed from phosphatidate by phosphatidate phosphohydrolase to produce a diacylglycerol: This reaction occurs in the smooth endoplasmic reticulum and cytosol. Finally, a last LCFA-CoA is esterified by the enzyme diacylglycerol acyltransferase, an enzyme located in the smooth endoplasmic reticulum (Bernlohr et al., 2002): If the triacylglycerol has been synthesized in adipose, it will migrate into the large storage vesicle that each adipocyte possesses. Most of the triacylglycerol synthesized in liver normally will be incorporated into and exported from the liver as part of very low density lipoproteins (VLDL). However, if triacylglycerol synthesis exceeds hepatic export capacity, triacylglycerol will accumulate in vesicles in hepatocytes, leading to fatty liver. If the triacylglycerol has been synthesized in mammary gland, the resulting triacylglycerols will accumulate in vesicles of secretory cells, and the vesicles will be extruded into the lumina of the gland acini. In liver, the limiting enzyme in the pathway appears to be phosphatidate phosphohydrolase. This enzyme is subject to an interesting control mechanism in which it is switched between a less active and more active state by the enzyme itself being translocated between the cytosol and endoplasmic reticulum, respectively. Intracellular cAMP, which increases with high plasma glucagon or low plasma insulin levels (e.g., fasting or diabetes), inhibits binding of the enzyme to the endoplasmic reticulum, whereas LCFA or LCFA-CoA promote binding of the enzyme to the endoplasmic reticulum (Bernlohr et al., 2002; Gurr et al., 2002). The role of LCFA and LCFA-CoA in promoting synthesis of triacylglycerols in the liver is important and explains how fat synthesis and fatty liver can occur in the fasting state when hormonal changes would oppose triacylglycerol synthesis. In adipose tissue, the synthesis of triacylglycerol is very much regulated by hormones, especially glucagon, catecholamines, and insulin. The first two hormones increase intracellular cAMP, and the latter tends to decrease it, although insulin probably has effects independent of cAMP. In conditions in which glucagon would be elevated and insulin would be decreased (e.g., fasting), hormone-sensitive lipase will be activated and lipolysis will be occurring. It is important that fat synthesis not be operative during lipolysis, so as not to waste energy. Low insulin and elevated catecholamine or glucagon levels decrease the level of lipoprotein lipase (LPL) in adipose tissue. Fat cells need LPL in order to hydrolyze plasma triacylglycerol so that the resulting LCFA can be absorbed and used for triacylglycerol synthesis. Decreased plasma insulin levels will decrease entry of glucose into adipocytes, which will result in less glycerolphosphate being synthesized. Increased intracellular cAMP in adipose tissue decreases the activity of several key enzymes in fat synthesis, including fatty acyl-CoA synthetase, glycerolphosphate acyltransferase, phosphatidate transferase, and diacylglycerol acyltransferase; however, the mechanism of inhibition is uncertain (Saggerson, 1988). Catabolism of triacylglycerol involves the action of lipases, which are specialized esterases that hydrolyze glyceride bonds. The major lipases are pancreatic lipase, hepatic lipase, hormone-sensitive lipase of adipose, lipoprotein lipase found on endothelial cells, and lysosomal lipases contained in most cells. Pancreatic lipase is the essential lipase for digestion of triacylglycerol in the GI tract and is discussed later. Hepatic lipase is synthesized in hepatocytes from where it migrates to the surface of hepatic endothelial cells. Hepatic lipase primarily attacks triacylglycerol in the plasma, which are part very low density lipoprotein (VLDL) remnants to produce low-density lipoproteins (LDL), and it attacks triacylglycerol in high-density lipoproteins (HDL) as well. Lipoprotein lipase attacks triacylglycerol in chylomicrons and VLDL in plasma and is found on the endothelium of many organs and tissues, but it is in greatest quantity in adipose, heart, skeletal muscle, and mammary gland. Lipoprotein lipase is synthesized by the underlying tissue and migrates to the capillary endothelium where it is anchored on the cell surfaces to glycoproteins, which have polysaccharide chains structurally similar to heparin. If heparin is injected into an animal, lipoprotein lipase can switch its attachment from cell surface glycoproteins to the free injected heparin and, thus, appears in the plasma. If the animal had a lipemia before injecting the heparin, the large amount of lipoprotein lipase released into the plasma will clear the lipemia. Phospholipids and apolipoprotein C-II must be present for lipoprotein lipase to have full activity (Fielding and Fielding, 2002). Most of the phospholipids found in the body consist of a core of glycerol, which has LCFA esterified to its 1 and 2 carbons and phosphate esterified to its 3 carbon, a compound called phosphatidate. In addition, the phosphate is often esterified to a hydroxyamino compound such as choline, ethanolamine, or serine to produce phosphatidylcholine (also called lecithin), phosphatidylethanolamine, and phosphatidylserine, respectively. Inositol may be esterified to the phosphate to produce phosphatidylinositol. Because of the phosphate group, phospholipids are very polar on one end but are nonpolar on the other end and still must be part of lipoproteins for transport through the plasma. Phospholipids are constituents of all cellular membranes, lipoproteins, and bile micelles. The fatty acid portion of the molecule is oriented toward the center of the membrane or micelle, and the phosphatidyl group is oriented toward the outer surface (i.e., toward the aqueous medium). In micellar structures, like lipoproteins and bile micelles, the surface coating of the polar ends of constituent phospholipids provides a surface charge that helps to keep the micelles in suspension. Phospholipids are synthesized either from phosphatidate (e.g., phosphatidylinositol) or diacylglycerol (e.g., phosphatidylcholine and phosphatidylethanolamine), both of which are intermediates in the synthesis of triacylglycerol. In all cases, cytidine triphosphate (CTP), a high-energy organophosphate that derives its phosphates from ATP, plays an important role. In the case of phosphatidylinositol, CTP reacts with phosphatidate to form CDP-diacylglycerol, which then reacts with inositol to form phosphatidylinositol and CMP. In the case of choline or ethanolamine, it must first be phosphorylated by reaction with ATP. Then the phosphocholine or phosphoethanolamine reacts with CTP to form CDP-choline or CDP-ethanolamine, respectively, which then reacts with diacylglycerol to produce phosphatidylcholine and phosphatidylethanolamine, respectively. Phosphatidylserine is formed by serine replacing ethanolamine in phosphatidylethanolamine. In the endoplasmic reticulum of the liver, a methyl group from S-adenosylmethionine can be transferred to phosphatidylethanolamine to produce phosphatidylcholine (Vance, 2002a). Figure 4-3 illustrates the synthesis of phospholipids. The enzymes that synthesize CDP-choline and CDP-ethanolamine (cytidylyltransferases) appear to be rate limiting for the synthesis of phosphatidylcholine and phosphatidylethanolamine, respectively. Phosphocholine cytidylyltransferase is subject to regulation similar to that of phosphatidate phosphohydrolase, the control enzyme in triacylglycerol synthesis. When phosphocholine cytidylyltransferase is bound to the endoplasmic reticulum, it is relatively active, but when it is free in the cytosol, it is relatively inactive. Factors that increase binding of the enzyme to the endoplasmic reticulum are decreased levels of phosphatidylcholine, increased levels of diacylglycerol or LCFA, and dephosphorylation of the enzyme. Opposite changes in these factors inhibit binding of the enzyme to the endoplasmic reticulum forcing it to remain inactive (Vance, 2002a). Phospholipids are hydrolyzed by phospholipases, which can be found in lysosomes of most tissues and in pancreatic secretion. Mammalian phospholipases are primarily of the A type, meaning that they hydrolyze the glycerol-LCFA ester bond at either position 1 (A1 type) or 2 (A2 type), but not both (Gurr et al., 2002; Waite, 2002). Phospholipase types B, C, and D, which hydrolyze at other locations in the molecule, exist in mammalian tissues, but with lower activities. FIGURE 4-3 Synthesis of phospholipids. Diacylglycerol is the lipid to which organic bases and phosphate are transferred via CDP derivatives. Abbreviations: CTP, CDP, CMP, cytidine tri-, di-, and mono-phosphate, respectively. Structurally, cholesterol is composed of a core of phenanthrene to which a cyclopentane ring is attached, and there is an eight-carbon side chain attached to the cyclopentane ring (Fig. 4-4). Cholesterol is found only in animals and is not present in plants or microorganisms. Cholesterol is the precursor of steroid hormones, vitamin D, and the bile acids, and is a constituent of cell membranes and bile micelles. Cholesterol can be obtained from the diet if it contains animal products, or it can be synthesized. The chief synthetic and catabolic organ for cholesterol is the liver. Steroidogenic endocrine organs (adrenal cortex, testis, ovary, placenta) can synthesize small amounts of cholesterol; however, these organs utilize hepatically synthesized cholesterol for most of their steroid synthesis (Pedersen, 1988). Pure cholesterol and cholesterol esters are insoluble waxy white solids and must be transported through plasma as part of lipoproteins. FIGURE 4-4 Synthesis of cholesterol. The first two reactions occur in the cytosol and the remainder in the smooth endoplasmic reticulum. Enzymatic methods are used almost universally for assay of cholesterol (Stein and Meyers, 1994). Older nonenzymatic methods used harsh reagents and lack specificity. The key enzymes in the assay are cholesterol esterase, which hydrolyses cholesterol esters, and cholesterol oxidase. The latter enzyme is of microbial origin and has an action analogous to that of glucose oxidase (i.e., it uses dissolved oxygen to oxidize cholesterol to produce cholest-4-ene-3-one and hydrogen peroxide). In the presence of added peroxidase, hydrogen peroxide will oxidize an added organic dye (e.g., dianisidine, ABTS, 4-aminoantipyrine plus phenol) to generate a colored product that can be quantified spectrophotometrically. If cholesterol esterase is included in the reagent, then total cholesterol will be determined. If cholesterol esterase is omitted from the reagent, then only nonesterified (i.e., free) cholesterol will be determined. If the assay is done with and without cholesterol esterase, then cholesterol ester concentration can be determined by subtraction. Because virtually all of the cholesterol and cholesterol esters in plasma are part of lipoproteins, they must be liberated before they can be acted on by the enzymes of the reagent. This liberation can be accomplished by extracting cholesterol and its esters with an organic solvent before the assay or, more conveniently, by including small amounts of detergents (bile acids or artificial detergents) in the reagent (Stein and Meyers, 1994). As is the case for LCFA and ketones, the substrate for cholesterol synthesis is acetyl-CoA. The beginning site of cholesterol synthesis is in the cytosol, so acetyl-CoA, which is generated primarily in the mitochondria, must be transferred to the cytosol via the citrate shuttle mechanism discussed earlier. The process of cholesterol synthesis is shown diagrammatically in Figure 4-4. In the cytosol, the first two steps of cholesterol synthesis are identical to the first two steps of ketone synthesis except that the process occurs in the cytosol rather than in the mitochondria. The enzymes that catalyze the first two steps are acetyl-CoA: acetoacetyl-CoA thiolase and hydroxymethylglutaryl-CoA (HMG-CoA) synthase: HMG-CoA reductase is the primary control point for cholesterol synthesis, and its control mechanisms will be discussed later. Next, via three steps, isopentenylpyrophosphate is formed. Six of these molecules, often called the active isoprenoid units, are linked to form cholesterol in a long and complex pathway, which is only partially understood (Faust et al., 1988; Liscum, 2002). The control of HMG-CoA reductase is complex and not completely understood. Artificially increasing plasma cholesterol levels in vivo decreases the activity of the enzyme in liver. However, cholesterol does not inhibit the enzyme directly, but represses synthesis of the enzyme mRNA (Liscum, 2002). Thus, if the amount of cholesterol consumed in the diet increases, the amount synthesized by the liver will decrease. This reciprocal relationship between cholesterol consumed and hepatic synthesis limits the extent to which plasma cholesterol levels can be decreased by restricting the amount of cholesterol in the diet. Hepatic HMG-CoA reductase is inhibited by phosphorylation of the enzyme and reactivated by dephosphorylation. The protein kinase system responsible for the phosphorylation of HMG-CoA reductase is stimulated by intracellular cAMP (Liscum, 2002). Hepatic intracellular cAMP levels are controlled in part by plasma glucagon, which increases it, and by insulin, which decreases it. Thus, conditions that increase insulin (e.g., eating) will increase cholesterol synthesis, and conditions that decrease insulin (e.g., diabetes) or increase glucagon (e.g., fasting) will decrease cholesterol synthesis. Other hormones that affect hepatic HMG-CoA reductase activity, but probably not by altering intracellular cAMP levels, are thyroid hormones (increase HMG-CoA reductase activity) and glucocorticoids (decrease HMG-CoA reductase activity). Some drugs, such as lovastatin and mevastatin, used in humans to decrease plasma cholesterol levels, operate by inhibiting HMG-CoA reductase (Brown and Goldstein, 1990). Once cholesterol has been synthesized in the hepatocyte, it can be secreted into the plasma as part of lipoproteins (mostly in VLDL), it can be secreted into the canaliculi and become part of bile micelles, it can be degraded to bile acids, or it can be esterified to an LCFA by acyl-CoA:cholesterol acyltransferase (ACAT), which is located in the smooth endoplasmic reticulum. Cholesterol esters are even less soluble than cholesterol and are found in membranes and micelles wherever cholesterol itself is found. Cholesterol ester can be exported as part of lipoproteins, or it can be converted back to cholesterol plus LCFA by cholesterol ester hydrolases, which are found in the cytosol, endoplasmic reticulum, and lysosomes. De-esterification is mandatory before cholesterol can be catabolized to bile acids. Because enzymes for the final steps of cholesterol synthesis and the first steps of its degradation are colocated in the endoplasmic reticulum, it might seem that most newly synthesized cholesterol would be immediately degraded. However, the negative feedback of bile acids on cholesterol degradation keeps this process in check. HDL contains lecithin:cholesterol acyltransferase (LCAT), which esterifies cholesterol by transferring an LCFA moiety from lecithin (phosphatidylcholine). The cholesterol to be esterified by LCAT can be then secreted with HDL at the time of its synthesis, or it can be cholesterol from other lipoproteins or cell membranes that come in contact with HDL at a later time. Lipoproteins are very large noncellular conglomerations (micelles) of lipids and proteins, which are suspended in plasma or lymph. Their main function is to transport most lipids (steroid hormones and LCFA being notable exceptions) among tissues. Another function of lipoproteins is the esterification of cholesterol. Lipoproteins have a micellar structure in which the least polar molecules (triacylglycerol and cholesterol) occupy the center and more polar molecules (proteins and phospholipids) coat the exterior. Lipoproteins are synthesized almost exclusively by liver and the small intestine. The main classes of lipoprotein are defined by their density as determined by ultracentrifugation and are chylomicrons (d < 0.94 g/ml), very low density lipoproteins (VLDL, d = 0.94 to 1.006 g/ml), low-density lipoproteins (LDL, d = 1.006 to 1.063 g/ml), and high-density lipoproteins (HDL, d = 1.063 to 1.21 g/ml). Less commonly considered are very high density lipoproteins (VHDL, d > 1.21 g/ml), which are usually very low in concentration in plasma. It is common to designate the lighter LDL (d = 1.006 to 1.019 g/ml) as intermediate density lipoproteins (IDL), and some schemes subdivide the HDL into HDL1, HDL2, and HDL3. (Chapman, 1986; Gotto et al., 1986). The lipid component of lipoproteins is less dense than the protein component, but the lipids have similar densities, and the proteins have similar densities. Therefore, the density of a lipoprotein is almost entirely dependent on its ratio of lipid to protein, with the chylomicrons having the highest ratio and, on the other end of the spectrum, the HDL having the lowest ratio (Table 4-1). More than one-half of the lipid in chylomicrons and VLDL is triacylglycerol, whereas in LDL and HDL the majority of the lipids are not triacylglycerol (Table 4-2). In domestic species, HDL is normally the most abundant plasma lipoprotein in the fasting state. TABLE 4-1 Composition of Lipoproteins of Domestic Animalsa aCattle: From Ferreri and Elbein (1982) (chylomicrons) and Stead and Welch (1975) (other lipoproteins). Dogs: From Blomhoff et al. (1978) and Mahley and Weisgraber (1974). Horses: From Le Goff et al. (1989) and Watson et al. (1993). Chylomicrons and VLDL particles are large enough to refract light significantly, so they make plasma appear turbid or creamy if in high enough concentration (lipemic plasma). The chylomicrons have a low enough density that they will rise to the top of an undisturbed refrigerated plasma sample in 6 to 12 hours. This phenomenon is the basis of the “chylomicron test,” in which a milky plasma sample is placed in the refrigerator overnight. If a “cream layer” has formed at the top, then hyperchylomicronemia is present, and if the bottom portion of the plasma is turbid, then elevated levels of VLDL are present. Because of the expense, time, and complexity involved with ultracentrifugation, electrophoresis in an alkaline medium has been used as an alternate method of lipoprotein classification. A variety of electrophoretic supports, ranging from paper to acrylamide gels, have been used. The sample is applied at the cathode end of the support, voltage is applied for a variable time, and the proteins are fixed and stained with a lipid stain such as oil red O. A densitometer is used to quantify the lipoprotein fractions on the stained electrophoretogram. Typically, three to five bands of lipoproteins can be discerned; however, additional bands may be present depending on the species of animal, electrophoretic technique, and presence of abnormal lipoproteins. The fastest moving band is HDL, which is designated as α-lipoprotein. The next fastest moving band is VLDL, which is designated pre-β-lipoprotein followed by the LDL band, which is designated as β-lipoprotein. The slowest moving band, which is still at the origin and seen primarily in the postprandial period, is composed of chylomicrons. With some electrophoresis systems, a separate IDL band, designated as slow pre-β-lipoproteins, can be discerned between the VLDL and LDL bands, and sometimes subbands of the HDL can be discerned. The correlation of electrophoretic and ultracentrifuge fractions established for humans does not always apply to animals. For example, bovine LDL can appear as α– or β-bands on electrophoresis (Puppione, 1983). Usually, two HDL bands can be discerned for dog plasma (Rogers, 1977). Figure 4-5 illustrates the distribution of lipoproteins in dog plasma. Although easier and cheaper to perform than ultracentrifugation, lipoprotein electrophoresis still requires considerable time and expense. Consequently, methods have been developed that involve precipitation of one or more lipoprotein classes followed by analysis of a particular lipid, usually cholesterol, in the remaining supernatant. For example, chylomicrons can be removed by low-speed centrifugation (they rise to the top), and then precipitation of VLDL and LDL in human plasma can be accomplished by treatment with magnesium and dextran sulfate. The main lipoprotein remaining in the supernatant will be HDL, and if cholesterol is determined, it will mostly be HDL cholesterol (Stein and Meyers, 1994). Such empirical methods may be species specific. For example, the preceding method, though valid for human plasma, does not work for dog plasma (Rhodes et al., 1992). FIGURE 4-5 Densitometric scan of an electrophoretogram of canine plasma lipoproteins. The scan is typical of a fasted dog. In a fed dog, an additional peak as a result of chylomicrons would be present at the origin. Abbreviations: HDL, LDL, and VLDL, high-, low-, very low density lipoproteins, respectively. The protein components of lipoproteins are called apolipoproteins. Some apolipoproteins are found in only one class of lipoproteins, whereas others can be found in multiple classes. Although there are species variations in the amino acid sequences of apolipoproteins, individual apolipoproteins in the domestic species are quite similar. The main classes of apolipoproteins are designated with a letter (A through E), sometimes followed by a number to indicate a distinct subclass. The main classes and subclasses of apolipoproteins found in domestic animals are A-I, A-II, A-IV, B48, B100, C-I, C-II, C-III, C-IV, and E. Characteristics of these apolipoproteins are listed in Table 4-2. The B100 apolipoprotein, synthesized in the liver and part of VLDL, is one the largest polypeptide chains in mammals, having a molecular weight of 527,000 in horses (Watson et al., 1991). B48 apolipoprotein is about one-half the size of B100 and contains a subset of the B100 amino acid sequence (i.e., they are probably coded by the same gene); however, B48 is synthesized in the small intestine and is part of chylomicrons. The origin of the “48” and “100” designations stems from the fact that human B48 is exactly 48% of the mass of human B100. Both B48 and B100 are glycoproteins and have a variety of carbohydrates attached to them (Chapman, 1986). In addition to apolipoproteins, HDL contains an additional protein in the form of the enzyme lecithin:cholesterol acyltransferase (LCAT), which esterifies cholesterol esters by transferring an LCFA moiety from phosphatidylcholine (lecithin) to cholesterol. LCAT is activated by lipoprotein A-I. The largest lipoproteins are the chylomicrons, and to understand their formation, the digestion of triacylglycerol must be discussed. The main site of digestion and absorption of triacylglycerol is the small intestine, and the chief enzyme involved is pancreatic lipase. The pancreas not only supplies lipase to attack triacylglycerol but also supplies cholesterol esterase to hydrolyze cholesterol esters and phospholipase A2 to attack phospholipids. For any of these enzymes to be effective, the lipids in food must first be emulsified with bile. Bile contains micelles composed mostly of bile acids, phospholipids, and cholesterol. Fats in food become part of these micelles, and then the enzymes can attack them on the outer surface of the micelles. The fatty acids, monoacylglycerols, and cholesterol resulting from the attack of the enzymes become part of the lipids of the brush border of the intestinal cells. The intestinal cells then use the monoacylglycerols and fatty acids to resynthesize triacylglycerol. Globules of triacylglycerol coated with protein are extruded from the basolateral membranes into the interstitium as chylomicrons. The lymphatic capillaries of the microvilli are called lacteals and have many large openings between the endothelial cells that line them. Consequently, the chylomicrons can enter the lymphatics, but not the blood capillaries. From the small intestine, the lymph flows to larger abdominal ducts to the thoracic duct and enters the right atrium. Thus, unlike most other nutrients, most of the absorbed fat bypasses the portal system and liver (Gurr et al., 2002; Vance, 2002b). The main apolipoproteins in chylomicrons are A-series, B48, C-series, and E. The A-series and B48 apolipoproteins are added by the small intestine, but the C-series and E apolipoproteins, which are synthesized in the liver, appear to transfer from HDL to nascent chylomicrons soon after they are released into the circulation. Chylomicrons are attacked by lipoprotein lipase, which resides on the surface of endothelial cells and hydrolyses triacylglycerol. Most of the resulting LCFA are absorbed by the tissue cells. As the chylomicron diminishes in size, some of the apolipoproteins, mostly A-series and C-series, transfer to HDL. Finally, a much diminished chylomicron remnant is left and will attach to an apolipoprotein-E receptor on hepatocytes. The remnant will be absorbed and its components hydrolyzed within the hepatocytes (Fielding and Fielding, 2002; Schneider, 2002). The transport and metabolism of chylomicrons are illustrated in Figure 4-6. Secretion of very low density lipoproteins (VLDL) into the plasma is the main method by which hepatocytes export triacylglycerol. Its main apolipoproteins are B100, C-series, and E, but some A-series is present as well. The A-series apolipoproteins, which are synthesized in the small intestine, transfer from HDL to VLDL soon after its secretion. Some C-series and E apolipoprotein may transfer from HDL to newly secreted VLDL as well. Like chylomicrons, the main lipid component of VLDL is triacylglycerol (see Table 4-1). VLDL and chylomicrons both serve as a means to distribute triacylglycerol to tissues. In the case of chylomicrons, the triacylglycerol is a product of fat digestion, whereas in the case of VLDL, the triacylglycerol is synthesized in the liver. TABLE 4-2 Apolipoproteins of Domestic Animalsa aFrom Bauchart (1993), Chapman (1986), Demacker et al. (1987), Watson et al. (1991), and Watson and Barrie (1993). FIGURE 4-6 Metabolism and transport of chylomicrons. Abbreviations: A, B48, C, E, apolipoproteins; HDL, high-density lipoprotein; TAG, triacylglycerol. The assembly process for VLDL is complex (Fig. 4-7). Final steps in the synthesis of triacylglycerol, phospholipid, and cholesterol occur in the smooth endoplasmic reticulum. Microdroplets containing these three lipids and cholesterol esters move toward the confluence of the rough and smooth endoplasmic reticulums where they are joined by apolipoproteins synthesized on the rough endoplasmic reticulum to form the nascent VLDL. The nascent VLDL particles move through microtubular membranes to the Golgi apparatus where the apolipoproteins are glycosylated. In the Golgi apparatus, the nascent VLDL particles reach final composition and are surrounded by membranes to form secretory granules. The secretory granules merge with the plasma membrane and spill their contents into the plasma (Alexander, 1976; Vance, 2002b). The capacity of the liver to synthesize the protein components of VLDL is stimulated by a diet high in carbohydrate. It has been hypothesized that this stimulation is due to increased insulin and decreased glucagon levels in plasma. Most studies have shown that glucagon partially inhibits hepatic VLDL secretion, whereas insulin stimulates it (Gibbons, 1990). Estrogens (Crook and Seed, 1990; Haffner and Valdez, 1995; Sacks and Walsh, 1994) and glucocorticoids (Gibbons, 1990; Martin-Sanz et al., 1990) stimulate VLDL secretion. The inherent capacity of the liver to synthesize the lipid components exceeds its inherent capacity to synthesize the protein components, a fundamental factor in the development of fatty liver. In addition, phosphatidylcholine is essential for lipoprotein assembly, so animals having a deficiency of choline tend to develop fatty livers (Vance, 2002a, 2002b). Triacylglycerol in plasma VLDL is hydrolyzed by lipoprotein lipase just like triacylglycerol of chylomicrons, and most of the released LCFA is absorbed by the underlying tissue cells. As the VLDL shrink, some of the apolipoproteins (C-series and E) transfer to HDL. Finally, the shrinking VLDL becomes an IDL and then an LDL. The LDL will attach to an apoprotein B100 or E receptor on hepatocytes or extrahepatic tissues and be taken into the cell where its component parts will be hydrolyzed. The transport and metabolism of VLDL are illustrated in Figure 4-8. FIGURE 4-7 Synthesis of very low density lipoprotein (VLDL) in liver. Triacylglycerol and phospholipid synthesis occurs in the smooth endoplasmic reticulum to generate lipid particles (large dots), which acquire small amounts of cholesterol and its esters as well. Apolipoproteins (small dots) are synthesized on the rough endoplasmic reticulum. Lipid particles acquire apolipoproteins at the convergence of the rough and smooth endoplasmic reticulum or by merging of sections of the two organelles. The nascent VLDL move through tubular membranes to the Golgi apparatus where apolipoproteins are glycosylated, and the nascent VLDL are collected in secretory vesicles. The secretory vesicles migrate to and merge with the plasma membrane (PM) and spill VLDL into the space of Dissé (SD). The VLDL migrate through the fenestrae (F) between endothelial cells (E) to enter the plasma in hepatic sinusoids. HDL is synthesized by both liver and small intestine. Nascent HDL produced in the small intestine has only A-series apolipoproteins and gains C-series and E apolipoproteins and LCAT, which are synthesized in the liver, from other lipoproteins after it enters the circulation. Nascent HDL produced in the liver gains its A-series apolipoprotein, which is synthesized in the small intestine, from other lipoproteins after it enters the circulation. HDL serves two main functions. It is a repository for A-series, C-series, and E apolipoproteins, and it transports cholesterol from peripheral tissues to liver. LCAT is important in this latter function. The conversion of cholesterol-to-cholesterol ester within HDL creates a favorable concentration gradient from tissue cell to HDL, which promotes migration of cholesterol from tissue cells to HDL (Fielding and Fielding, 2002; Gurr et al., 2002). FIGURE 4-8 Metabolism and transport of very low density lipoprotein (VLDL). Abbreviations: A, B100, C, E, apolipoproteins; HDL, IDL, and LDL, high-, intermediate-, and low-density lipoproteins, respectively; LCAT, lecithin:cholesterol acyltransferase; LCFA, long chain fatty acid; TAG, triacylglycerol. HDL is removed from the circulation primarily by the liver, and its component parts can be metabolized within the hepatocyte or some of its lipid components can be incor-porated into VLDL and enter the plasma again. Cholesterol can migrate from HDL into hepatocytes without the entire HDL being removed, and as mentioned earlier, apolipoproteins can migrate from HDL to chylomicrons, VLDL, and other HDL. In summary, chylomicrons and VLDL distribute triacylglycerol, cholesterol, and phospholipids from the small intestine and liver, respectively, to other tissues. IDL and LDL are effectively remnants of VLDL. HDL is a reservoir of some apolipoproteins and transports cholesterol from peripheral tissues to liver. Hyperlipidemia refers to increased plasma levels of cholesterol (hypercholesterolemia) and triacylglycerols (hypertriacylglycerolemia or hypertriglyceridemia). Note that increased plasma levels of LCFA alone do not constitute hyperlipidemia. Because cholesterol and triacylglycerols must reside within lipoproteins in plasma, hyperlipidemia is synonymous with hyperlipoproteinemia. Lipemia is a term denoting that hyperlipidemia is severe enough that the plasma looks milky (i.e., lactescent). If lipemia is marked, whole blood may have a light red color or “tomato soup” appearance. The most common form of hyperlipidemia is postprandial hyperlipidemia, which is observed after an animal consumes a meal containing fat and is due primarily to increased chylomicron levels. For evaluation of possible abnormalities in lipid metabolism, it is important that blood samples be taken from fasting animals to avoid confusion caused by postprandial hyperlipidemia. One exception is adult ruminants, which are usually on a very low fat diet and, because of the volume of the rumen and fermentative nature of digestion there, have absorption spread over a considerable time period. Healthy dogs normally do not develop significant hyperlipidemia upon fasting. Therefore, fasting hyperlipidemia in a dog usually is an abnormal sign with potential causes being hypothyroidism, diabetes, pancreatitis, hyperadrenocorticism, hepatic disease, nephrotic syndrome, and inherited defects in lipid metabolism. Hyperlipidemia is commonly observed in dogs with hypothyroidism, whether of congenital or acquired origin (Barrie et al., 1993; DeBowes, 1987; Liu et al., 1986; Manning et al., 1973; Medaille et al., 1988; Watson and Barrie, 1993). The main lipid that is increased is cholesterol, but triacylglycerol may be increased too, and most of the increased lipid is in LDL and HDL, but some animals have increased VLDL or chylomicron levels as well (Rogers, 1977; Whitney, 1992). The mechanism by which hypothyroidism causes hyperlipidemia in dogs is unknown; however, a similar phenomenon occurs in humans, and in that species, it appears that hypothyroidism decreases lipoprotein lipase and hepatic lipase activities (Valdemarsson et al., 1983). The prolonged hypercholesterolemia associated with chronic hypothyroidism in dogs may lead to atherosclerosis (Liu et al., 1986; Patterson et al., 1985), although other factors may be involved as well. Dogs with naturally occurring pancreatitis frequently have hyperlipidemia (Hardy, 1992; Whitney et al., 1987). Because the pancreatitis in some of these animals causes diabetes, the hyperlipidemia in those individuals may be due to diabetes. Plasma lipid levels of dogs with pancreatitis induced by injecting bile into or ligating the pancreatic duct are comparable to control dogs (Bass, 1976; Whitney et al., 1987; Zieve, 1968). In some cases, hyperlipidemia may play a role in the pathogenesis of pancreatitis rather than being a result of pancreatitis. This proposition is supported by the fact that humans with some forms of hyperlipidemia have increased risk of pancreatitis (Cameron et al., 1974; Greenberger, 1973). One proposed mechanism is that increased lipids, especially chylomicrons, entering the pancreatic capillaries will be hydrolyzed by pancreatic lipase, and the resultant LCFA may injure endothelial or acinar cells (Havel, 1969). Once the initial damage occurs, there is a positive feedback in which more lipase enters the circulation and hydrolyses more triacylglycerol leading to more LCFA release and more damage. In support of this theory, when dog pancreata were perfused with a medium containing high levels of triacylglycerol or LCFA, they became edematous and hemorrhagic and released large amounts of amylase compared to pancreata perfused without these additions (Saharia et al., 1977). In a related proposed mechanism, large amounts of chylomicrons or VLDL may impede the microcirculation of the pancreas, leading to partial stasis, which allows blood lipids and their hydrolysis products more contact with pancreatic cells (Hardy, 1992). Dogs with uncontrolled diabetes frequently have hyperlipidemia (Medaille et al., 1988; Rogers, 1977; Whitney, 1992). In naturally occurring cases, plasma triacylglycerol levels are increased with concomitant increases in VLDL levels and often hyperchylomicronemia is present as well (DeBowes, 1987; Ford, 1995; Rogers, 1977; Rogers et al., 1975b). The increase in VLDL is due in part to increased mobilization of LCFA from adipose. The liver removes LCFA from plasma and reissues some of them to the plasma as triacylglycerol in VLDL. In addition, synthesis of lipoprotein lipase by peripheral tissues is partially dependent on insulin, so less of this enzyme is available to remove triacylglycerol from the circulation (Brown and Goldstein, 1994). Hyperlipidemia with increases in plasma triacylglycerol and cholesterol levels have been noted in dogs with cholestasis (Bauer et al., 1989; Meyer and Chiapella, 1985). The increase in cholesterol can be explained in part by the inability of the liver to remove and catabolize cholesterol. However, there is evidence of production of an abnormal LDL, called lipoprotein-X, which is rich in cholesterol (Bauer et al., 1989; Blomhoff et al., 1978; Danielsson et al., 1977; Meyer and Chiapella, 1985). Dogs with hyperadrenocorticism (Cushing’s disease) often have hyperlipidemia with increased total plasma cholesterol levels (Barrie et al., 1993; Feldman, 1995; Ling et al., 1979; Medaille et al., 1988; Scott, 1979). Most of the increased plasma cholesterol is associated with LDL, and although the mechanism of the hyperlipidemia is unclear, it may be related to a decrease in activity of hepatic LDL receptors (Barrie et al., 1993). Dogs with nephrotic syndrome often have hyperlipi-demia (Ford, 1995; Lewis and Center, 1984; McCullagh, 1978; Medaille et al., 1988). Hypercholesterolemia is present most commonly, but hypertriacylglycerolemia may be present as well, especially in more severe cases (McCullagh, 1978). In humans with nephrotic syndrome, the hyperlipidemia appears to be related to the loss of albumin or regulatory factors in the urine, and infusion of albumin or dextran into afflicted patients lowers lipid levels (Glassock et al., 1991). Albumin or regulatory factors may inhibit VLDL production by the liver, and without this inhibition, more VLDL will be released to the plasma increasing VLDL and LDL levels (Glassock et al., 1991). Idiopathic hyperlipidemia, which is probably inherited, occurs in some miniature schnauzers (Ford, 1993; Richardson, 1989; Rogers et al., 1975a). Animals present with abdominal pain, diarrhea, and vomiting, and sometimes with seizures and pancreatitis. Affected animals have hypertriacylglycerolemia, hypercholesterolemia, and increased chylomicron levels and often have increased levels of other lipoproteins as well. The animals often have elevated liver enzymes as well (Xenoulis et al., 2008). It has been proposed that these animals may have low levels of lipoprotein lipase or perhaps deficient apolipoprotein C-II, the activator of lipoprotein lipase. However, some dogs have shown clearing of the plasma following heparin injection, so the mechanism remains unknown. The primary treatment is to place the animal on a low-fat diet. A similar syndrome has been reported in mixed-breed dogs (Baum et al., 1969; Rogers et al., 1975a) and in Brittany spaniels (Hubert et al., 1987). Hypercholesterolemia, which is probably inherited, has been reported in rough collie dogs (Jeusette et al., 2004) and Shetland sheepdogs (Sato et al., 2000). Corneal lipidosis, which responded to dietary manipulation, was observed in the former, and possibly increased incidence of atherosclerosis was observed in the latter. Not surprisingly, some of the same diseases that are associated with hyperlipidemia in dogs are associated with hyperlipidemia in domestic cats, including diabetes and nephrotic syndrome (Jones, 1995; McCullagh, 1978; Watson and Barrie, 1993). Some cats, however, have a well-characterized familial hyperlipidemia because of lipoprotein lipase deficiency (Brooks, 1989; Jones, 1993, 1995; Jones et al., 1983; Watson et al., 1992b; Whitney, 1992). There is lipemia with hyperchylomicronemia and increases in plasma levels of cholesterol and triacylglycerol (Jones, 1993, 1995; Whitney, 1992). The high plasma levels of lipids lead to deposition in tissues (xanthoma formation) in the skin, nerve sheaths, and other locations (Jones, 1993; Whitney, 1992). Pressure on spinal or other nerves from xanthomas or subsequent granulomas may lead to peripheral neuropathy (Jones et al., 1986). The disease appears to be autosomal recessive, and homozygotes apparently manufacture a defective lipoprotein lipase and do not have a defective or missing apolipoprotein C-II activator (Peritz et al., 1990; Watson et al., 1992b). The phenomenon of equine hyperlipidemia was reported in horses with maxillary myositis (Hadlow, 1962) and equine infectious anemia (Gainer et al., 1966). It is likely that the hyperlipidemia described in these early reports was due to anorexia, and it has been shown that fasting alone causes hyperlipidemia in horses and that pregnancy, lactation, and obesity accentuate the effect (Eriksen and Simesen, 1970; Schotman and Kroneman, 1969; Schotman and Wagenaar, 1969; Schotman and Wensing, 1977). Total plasma triacylglycerol may increase from a normal fed value of less than 500 mg/l to more than 2000 mg/l (Morris et al., 1972; Naylor et al., 1980) and, in severe cases, may exceed 10,000 mg/l (Freestone et al., 1991; Naylor et al., 1980; Schotman and Wensing, 1977). Although most horses are susceptible to this effect of calorie deprivation, it is harmless for the majority, and only becomes pathological for a few. Ponies and females are more susceptible to the pathological syndrome, which can be fatal (Hughes et al., 2004). Survival rates are inversely proportional to plasma triacylglycerol concentrations (Schotman and Wagenaar, 1969), and severe fatty liver and increased plasma levels of liver enzymes have been reported (Schotman and Wagenaar, 1969). Like in other mammals, fasting increases plasma levels of LCFA in horses and ponies (Baetz and Pearson, 1972; Naylor et al., 1980; Watson et al., 1992a), and the hyperlipidemia in horses and ponies is due to increased VLDL levels (Bauer, 1983; Morris et al., 1972; Watson et al., 1992a). Presumably, horse liver is removing LCFA from plasma and reesterifying them into triacylglycerol, which are released to the plasma as VLDL. It appears that the liver of fasting horses has a high capacity for reesterification of LCFA into triacylglycerol, which is exported as VLDL. Horses do have increased plasma ketone levels when fasting (Rose and Sampson, 1982), so some of the LCFA removed from plasma by liver are converted to ketones, but additional LCFA are reesterified to glycerol and are recycled to the plasma as triacylglycerol in VLDL. The mechanism of fasting equine lipemia is uncertain; it could be increased secretion or decreased uptake of VLDL or a combination thereof. In one study, fasting horses were injected with Triton WR 1339, a compound shown to inhibit lipoprotein lipase in rats, and the rate of increase in plasma triacylglycerol levels was observed (Morris et al., 1972) and compared with the preinjection level of triacylglycerol. The authors claimed that the rate of increase was not related to the level of triacylglycerol concentration. However, only four horses were used, which may not have been enough given the high variation in fasting triacylglycerol levels observed among them. On close examination of the data, it is apparent that three horses showed a perfect rank correlation of fasting triacylglycerol level and rate of triacylglycerol increase after Triton injection, with the fourth being a considerable outlier to the trend. In addition, the dose of Triton was such that all the horses developed anemia because of intravascular hemolysis. Thus, elucidation of the mechanism of fasting equine lipemia will have to await additional kinetic or enzyme studies. There are reports of apparent therapeutic success with intravenous glucose and oral carbohydrates (Dunkel and McKenzie, 2003; Durham, 2006; Hallebeek and Beynen, 2001; Mogg and Palmer, 1995; Watson and Love, 1994). This therapy makes sense in that increasing plasma glucose levels should lead to increased insulin and decreased glucagon levels, which should inhibit lipolysis in adipose which is generating plasma LCFA used for triglyceride synthesis. In addition, the hormonal changes may stimulate lipoprotein lipase activity. Although supplemental insulin has been used with carbohydrate therapy, its efficacy and safety have not been adequately evaluated, and one report indicates that hyperlipemic horse have hyperinsulinemia as well (Oikawa et al., 2006). The ketones or ketone bodies, which are composed of acetoacetic acid, 3-hydroxybutyric acid (also known as β-hydroxybutyric acid), and acetone, are important compounds in the metabolism of birds and mammals. Ketosis simply means that ketones are present in body fluids in elevated concentrations. Ketones are important clinically and have a rather sinister reputation because of the ketoacidosis that is often present when their plasma levels are high. In recent years though, the survival value of ketogen-esis has become clearer, and although increased levels of ketones in biological fluids will continue to be regarded as a pathological sign in many situations, perhaps the beneficial aspects of ketogenesis will be more widely appreciated. The ketones, acetone, 3-hydroxybutyric acid, and acetoacetic acid, are relatively simple chemical structures. Of the three, only 3-hydroxybutyric acid can exist as stereoisomers, having L-( + ) and D-(–) forms. Only the D-(–) form is produced in a free state in intermediary metabolism. The L-( + ) form exists only as its CoA thioester produced and destroyed in β-oxidation (Newsholme and Leach, 1983). Acetone is relatively volatile, whereas the other two ketones are not. Acetone has a characteristic organic solvent odor that may be detectable in the exhaled breath of animals with elevated blood ketone levels. Anecdotal evidence indicates that people vary greatly in their olfactory sensitivity for acetone. Acetone does not ionize appreciably, whereas 3-hydroxybutyric acid and acetoacetic acid do readily ionize. Acetoacetic acid has a pKa of 3.58, and 3-hydroxybutyric acid has a pKa of 4.41 (Dean, 1985). Consequently, at normal plasma pH of 7.40, 99.9% of either compound exists in its ionized form. Therefore, the compounds will usually be referenced by the names of their ions whenever their metabolism is discussed. Acetoacetic and 3-hydroxybutyric acids are more powerful acids than the volatile fatty acids (VFA; acetic, propionic, and butyric acids), which have pKa’s of 4.76 to 4.87 (Dean, 1985). Acetoacetic acid is more powerful, and 3-hydroxybutyric acid is less powerful as an acid than lactic acid, which has a pKa of 3.86 (Dean, 1985). Acetone and acetoacetic acid are miscible in water in all proportions, and 3-hydroxybutyric acid is exceedingly soluble, but not in all proportions (Dean, 1985). The common metallic salts of acetoacetic acid and 3-hydroxybutyric acid are soluble in water. Acetone and 3-hydroxybutyric acid and its salts are relatively stable compounds. Acetoacetic acid spontaneously decomposes to acetone and carbon dioxide. This reaction occurs readily without catalysis, and its rate is accelerated by increased temperature and hydrogen ion concentration. Apparently, there can be some nonspecific catalysis of acetoacetate decarboxylation by cellular proteins (Williamson, 1978). The lithium, sodium, and potassium salts of acetoacetic acid are relatively stable if stored in dry form below 0°C. a. Qualitative The most common qualitative test for ketones is the alkaline nitroprusside test, which is also known as the Rothera test (Rothera, 1908). This test has been used for decades in clinical practice and is still exceedingly useful today. The test relies on the reaction of nitroprusside with acetone or acetoacetate to produce a purple chromogen. The nitroprusside test has been used for virtually every body fluid imaginable including whole blood, serum, plasma, urine, and milk. The test is most sensitive for acetoacetate (0.5 mmol/l can be detected), gives only a slight response to acetone, and is completely insensitive to 3-hydroxybutyrate. The nitroprusside test is available commercially in the form of strips, tablets, and powders. The maximum sensitivity of all three forms is approximately 0.5 mmol/l, although specific formulations may have a sensitivity less than this value. The strip form is commonly used for urine. The powder form and strips are both commonly used for milk. The tablet form is used for serum, plasma, and whole blood and can be used for milk and urine as well. The test is often used in a semiquantitative manner with the result expressed in adjectival form (negative, weak, strong) or as a series of pluses (–, + , + + , etc.). A number of drugs or other substances may appear in urine and give a false positive with the nitroprusside test. Some compounds react with nitroprusside to yield a purple or near purple color. Included in this group are phenyl-ketones, levodopa, methyldopa, acetaldehyde, paraldehyde (Caraway and Kammeyer, 1972), cysteine, cysteamine, pen-icillamine, and mesna (Csako, 1987). In general, substances with keto, aldehyde, or sulfhydryl groups have the potential for reacting with nitroprusside. Because the nitroprusside test is performed in an alkaline medium, some substances, like sulfobromophthalein and phenolsulfonphthalein, which may exist in urine and are otherwise colorless, may yield a purple or near purple color simply because of the alkaline pH (Caraway and Kammeyer, 1972). More recently a semiquantitative color test for 3-hydroxybutyrate in milk has been used for diagnosis of clinical and subclinical ketosis in dairy cows (Gutzwiller, 1998; Jorritsma et al., 1998). This test, which is quite specific, is based on the same enzymatic method used for quantitative determination of 3-hydroxybutyrate (see the next section) except that a color visible to the human eye is produced. b. Quantitative Commonly used means of quantitative assay for ketone concentrations in biological fluids include microdiffusion methods, used primarily for assay of acetone, and enzymatic methods, used primarily for assay of acetoacetate and 3-hydroxybutyrate. Regardless of the method to be used for analysis, proper handling of the samples before analysis is crucial for obtaining representative results. In particular, the volatility of acetone and instability of acetoacetate must be respected. Blood samples should be cooled immediately after collection. Ketones can be determined on whole blood or plasma. Serum is not recommended because of losses, particularly of acetoacetate, that may occur during the time required for clotting. Any of the common anticoagulants (heparin, fluoride, oxalate, citrate, or EDTA) may be used. If whole blood is to be used, it should be mixed with perchloric acid immediately after collection to precipitate proteins. The tube should be chilled on ice until centrifuged, which should be performed within a few hours. The supernatant should be frozen until analyzed. If plasma is to be used, the red cells should be spun down within a few hours, and the plasma proteins precipitated with perchloric acid. The supernatant should be frozen until analyzed. The microdiffusion method can be used to determine the concentration of acetone or acetone plus acetoacetate in any biological fluid. The reagents are relatively simple and inexpensive although rather corrosive. The diffusion step requires specialized, but inexpensive, apparatus and adds to the complexity and time to complete the assay. The method relies on the reaction of acetone with vanillin (Henry et al., 1974) or salicylaldehyde (Nadeau, 1952) to produce a colored product that can be quantified in a spectrophotometer. In the author’s experience, vanillin provides more sensitivity than salicylaldehyde, but variability in the purity of vanillin batches from commercial sources makes salicylaldehyde the reagent of choice. Salicaldehyde must be stored under nitrogen or argon to preserve its purity. The method as described by Henry et al. (1974) was shown to determine acetone, and there was speculation that it would also detect acetoacetate simultaneously. In fact, the method as described by Henry et al. (1974) is specific for acetone. It has been found in the author’s laboratory that to use the method for acetone plus acetoacetate, it is necessary to preincubate the sample with an equal volume of 10 N sulfuric acid for 4 hours at 50°C in a sealed container to decarboxylate all of the acetoacetate. The method can be adapted to measure 3-hydroxybutyrate as well by introducing a step in which 3-hydroxybutyrate is oxidized to acetoacetate with potassium dichromate (Procos, 1961). However, if the primary interest is the determination of acetoacetate or 3-hydroxybutyrate, rather than acetone, the enzymatic method described later should be used. For determination of acetone on large numbers of samples, flow injection analysis (Marstorp et al., 1983) or infrared spectroscopy (Hansen, 1999) has been used. The enzymatic method for assay of acetoacetate or 3-hydroxybutyrate in biological fluids is accurate and precise (Williamson et al., 1962) and is probably the most common method used for quantitative assay of ketone concentrations. The method has been successfully adapted to a variety of automated analysis systems (Harano et al., 1985; Ozand et al., 1975; Työppönen and Kauppinen, 1980) and is a relatively straightforward spectrophotometric or fluorometric method. For a detailed step-by-step practical description of the method, see Mellanby and Williamson (1974) and Williamson and Mellanby (1974). The method relies on the reversible reaction catalyzed by 3-hydroxybutyrate dehydrogenase: The reaction is run in the forward direction by including an excess of NAD1 in the reaction mixture to assay 3-hydroxybutyrate and in the backward direction by including an excess of NADH in the reaction mixture to assay acetoacetate. The equilibrium constant of the reaction is 1.42 × 10–9 and therefore, is highly favorable toward the reduction of acetoacetate at pH 7.0 (Krebs et al., 1962). To force the reaction to completion in the direction of oxidizing 3-hydroxybutyrate, hydrazine is used as a trapping agent to remove acetoacetate as it is formed, and the reaction mixture is buffered at an alkaline pH. The change in NADH concentration is measured by the change in absorbance at 340 nm in either case. Alternately, a fluorometer can be used to measure the change in NADH concentration. To avoid interference from lactate or pyruvate in the sample, the 3-hydroxybutyrate dehydrogenase should be free of lactate dehydrogenase, or alternately, the lactate dehydrogenase inhibitor, oxamic acid, can be added to the reaction mixture (Harano et al., 1985). Table 4-3 lists normal blood and plasma ketone concentrations for several domestic species. The values are for healthy fed animals. It is assumed that plasma and blood ketone concentrations should be similar because of the generally high permeability of cell membranes to ketones and lack of protein binding of ketones; however, reports of definitive studies on this problem are not apparent in the literature. For clinical purposes, there is no lower normal limit for ketone concentrations. Ketones are primarily products of intermediary metabolism. Only under unusual circumstances would more than trace amounts be absorbed from the contents of the gastrointestinal tract. The real source of ketones is fatty acids including those with short (1 to 4 carbons), medium (5 to 11 carbons), and long (>11 carbons) chains. Of course, any compound (glucose, lactate, glycerol, amino acids, etc.) that can be converted to fatty acids can be considered as a source of ketones, but for the purposes of this discussion, the origin of ketones will be considered to be fatty acids, either esterified or nonesterified. TABLE 4-3 Blood and Plasma Ketone Concentrations of Domestic Animalsa aValues are means ± standard errors for healthy fed animals. Ketone concentrations were determined by the method of Williamson et al. (1962) or a modification thereof.
I. INTRODUCTION
II. LONG CHAIN FATTY ACIDS
A. Structure, Properties, and Assay of Long Chain Fatty Acids
B. Synthesis of Long Chain Fatty Acids
C. Catabolism of Long Chain Fatty Acids
1. Desaturation
2. β-Oxidation
III. TRIACYLGLYCEROL
A. Structure, Properties, and Assay of Triacylglycerol
B. Synthesis of Triacylglycerol
C. Catabolism of Triacylglycerol
IV. PHOSPHOLIPIDS
A. Structure and Properties of Phospholipids
B. Synthesis of Phospholipids
C. Catabolism of Phospholipids
V. CHOLESTEROL
A. Structure, Properties, and Assay of Cholesterol
B. Metabolism of Cholesterol
VI. LIPOPROTEINS
A. Structure, Properties, and Assay of Lipoproteins
B. Apolipoproteins
C. Digestion of Fat and Formation of Chylomicrons
D. Very Low Density Lipoprotein: Synthesis, Export, and Metabolism
E. Metabolism of High-Density Lipoproteins
VII. HYPERLIPIDEMIA
A. Introduction
B. Canine Fasting Hyperlipidemias
C. Feline Fasting Hyperlipidemias
D. Equine Fasting Hyperlipidemia
VIII. KETOGENESIS AND KETOSIS
A. Introduction
B. Chemistry of Ketones
1. Structure and Properties
2. Detection and Assay
C. Synthesis of Ketones
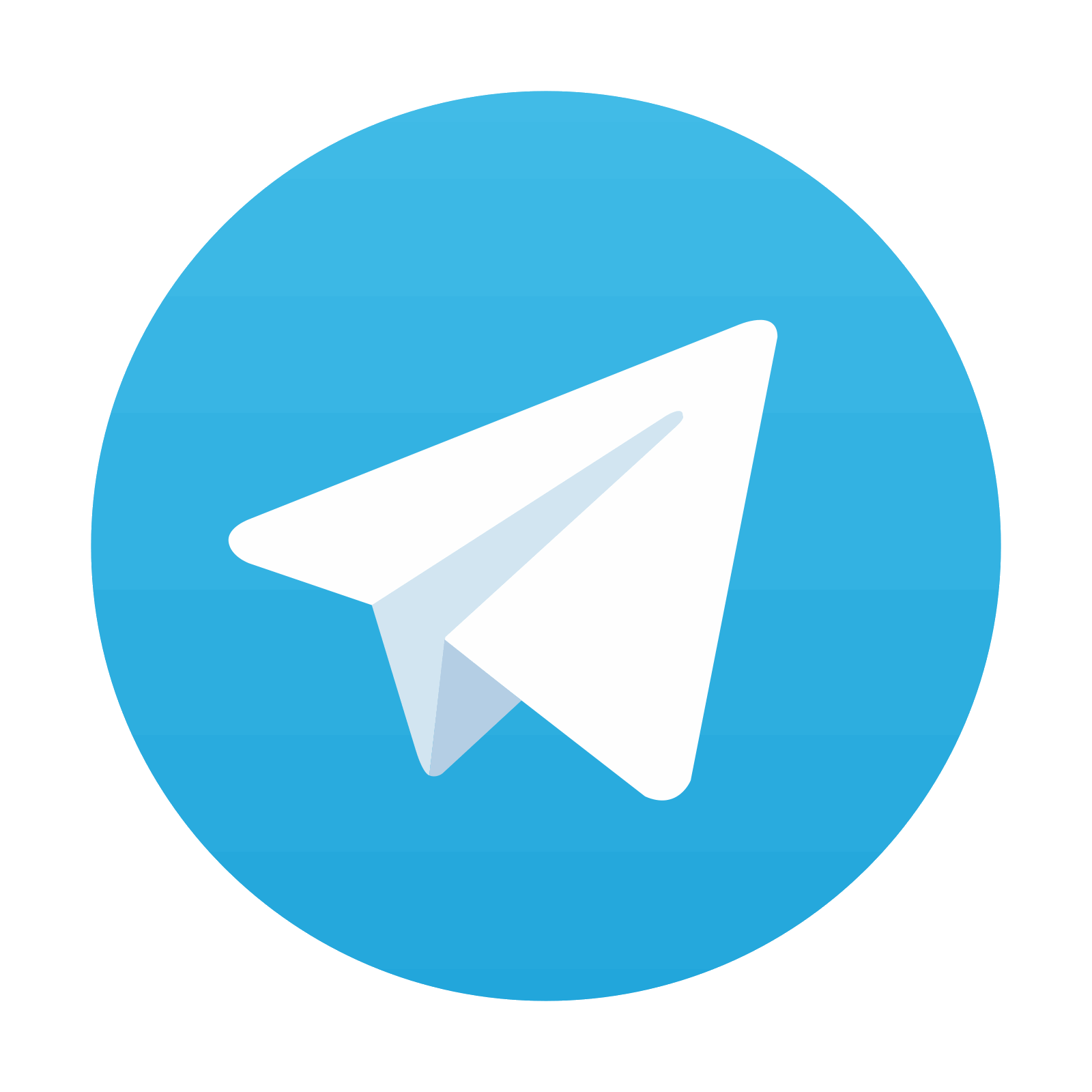
Stay updated, free articles. Join our Telegram channel
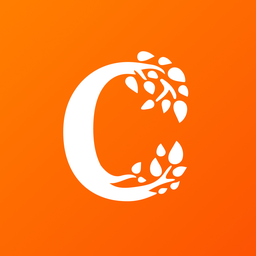
Full access? Get Clinical Tree
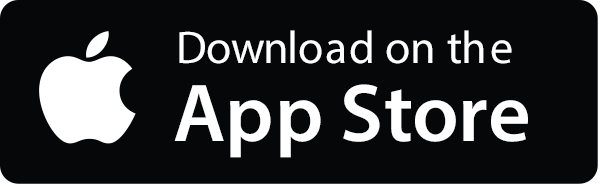
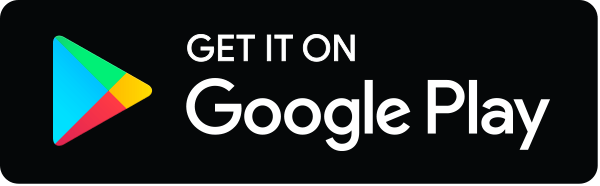