CHAPTER 17 The axial skeleton consists of the vertebral column from the first cervical vertebra down to the last coccygeal vertebra, and the pelvic bones. Together with the skeletal structures in the limbs, called the appendicular skeleton, it forms the bony basis of the equine musculoskeletal system that gives the horse its speed and agility. The species was originally domesticated approximately 5000 years ago (Dunlop and Williams, 1996). The role of the horse in society has changed significantly over the past 50 years from an economically, militarily working animal to a popular sports and leisure animal. The musculoskeletal system of the horse is considered the horse’s main asset. It may be not surprising, therefore, that disorders of the musculoskeletal system are the main reason for wastage of racehorses (Rossdale et al., 1985; Williams et al., 2001). Epidemiologic studies of equestrian disciplines other than racing are virtually nonexistent, but there is general consensus among equine veterinarians that the same applies to almost all other equine athletic activities. Historically, equine orthopedics has focused on limbs rather than the back. This is partly because not much overt lameness has its origin in the back but also because of the limited accessibility of this vast, complex structure. However, in the modern elite sport horse, it is often not the obvious, overt lameness that presents the biggest challenge to the equine practitioner but the marginally worse-than-normal performing horse that may suffer from very subtle pathologies or, more often, a combination of very minor to subclinical pathologies, adverse environmental conditions such as suboptimal riding or training techniques, ill-fitting tack, and so on. In this subtle interplay of physical factors and environmental conditions, as well as the mental states of both the horse and the rider, any impairment of function of the back or the pelvis may be hard to define, as is the case in humans, in whom lower back pain is one of the most important, but equally intangible, causes of occupational disability (Waddell and Main, 1998). The difficult accessibility of the equine back for diagnostic purposes, the complex interaction of back (dys)function with other physical and mental factors, and the lack of objective quantitative methods to evaluate therapies have provided an ample opportunity for the myriad of “equine therapists,” with highly variable degrees of skill and trustworthiness, who claim to be able to influence the function of the equine back. Installed therapies range from treatments that at least theoretically could have some physiological basis, for example, chiropractic treatment, to completely irrational actions such as imposition of hands or sending a hair from the affected animal to somebody with a black box, who will provide the diagnosis by mail (Jeffcott, 1998). Thanks to the significant and rapid advancements in both diagnostic imaging techniques and kinematic analysis in the past two decades, our understanding of the function and dysfunction of the equine back has improved considerably. These technological advances have greatly improved our diagnostic capacity and are a great help in the rationalization of the therapeutic approach to the equine back. In fact, they may help in the demystification of many therapies that claim effectiveness on irrational grounds. In the following sections of this chapter, which discusses the kinematics of the equine back and pelvis, a short introduction is given into the current biomechanical concept of the quadrupedal back. After a quick overall review of the fundamental research that has led to the currently used techniques to quantify kinematics of back and pelvis (for a more in-depth review, see van Weeren, 2009), the discussion will move on to the applications of back-related kinematical research to the modern performance horse and the significance of research outcome for both equine health care and the equestrian sports. The chapter concludes on a more speculative note, discussing some likely future developments and their possible impact. The Roman physician Galen (129–200 a.d.) is the first scientist known to have written about the concept of the mammalian back, describing it as a vaulted roof sustained by four pillars, that is, the limbs (cited by Slijper, 1946). The next concept dates from the middle of the nineteenth century. It depicts the back as a bridge, with the limbs representing the land abutments of the bridge, the gap between these being spanned by the bridge itself (Bergmann, 1847;Krüger, 1939; Zschokke, 1892). The bridge consists of an upper ledge, representing the supraspinous ligament; a lower ledge, representing the vertebral bodies; and a number of smaller girders between them, pointing in either the craniodorsal or the caudodorsal direction, representing the spinous processes and the interspinous ligaments (Figure 17-1). This model was generally accepted until the time of World War II and is even used by some today when the biomechanics behind equine locomotion and certain equestrian activities are discussed. However, it contains an important conceptual error. The upper ledge representing the supraspinous ligament and the lower ledge representing the string of vertebral bodies imply that the upper ledge is loaded under tension and that the lower ledge is under compression because ligamentous structures (such as the supraspinous ligament) inherently cannot withstand compressive loads. In reality, however, the always-downward-facing gravitational forces that act on bridges and mammalian trunks alike cause compression in the upper ledge and tension in the lower one. The bridge model has been replaced by the so-called “bow-and-string” concept, in which the bow is the thoracolumbar vertebral column and the string is the “underline” of the trunk, consisting of the linea alba, the rectus abdominis muscle, and related structures. The concept was already described in the late eighteenth century by Barthez (1798), but apparently he was too ahead of his time, and the model did not become generally accepted until its rediscovery by Slijper (1946) (Figure 17-2). The bow-and-string model is the first concept that takes a holistic view of the entire trunk and is not limited to only the thoracolumbar vertebral column with adnexa. As in an old-fashioned hand-bow, the entire system is under intrinsic tension due to a dynamic balance, and changes in one component will inevitably influence the biomechanical dynamics of the other. There are many factors that influence this dynamic balance (Figure 17-3). Gravitational forces will always act along a vertical line in a downward direction. They will, thus, tend to straighten the bow, that is, extend the back or make the back hollower. Of course, every horse is subjected to gravitational forces, but all factors that increase the mass on which the gravitational forces act will make the effect more pronounced. These factors include pregnancy (the typical hollow-backed broodmare), tack, and, of course, the rider, whose weight is of considerable importance, as elegantly demonstrated by DeCocq et al. (2004). Muscular action is another factor that influences the dynamic equilibrium between the bow and the string. Contraction of the ventral musculature will tense the bow, that is, flex the back or make it more arched. Contraction of the massive epaxial musculature will have the opposite effect because the work line of these muscles runs dorsal to the axis through the centers of the vertebral bodies. The only dorsally located muscles that have a flexing effect on the back are the psoas muscles. Because these are located along the pelvis, the ventral aspect of the lumbar, and the last three thoracic vertebrae (Koch, 1970), they will principally affect lumbosacral flexion. Further toward the craniad direction, there is no musculature ventral to the vertebral column, hence no flexing action on the spine occurs. Protraction and retraction of the limbs are important events that strongly affect the balance in the bow-and-string system. Protraction of the hindlimbs results in a more forward position of the point of support. Given the anatomic connection between the gluteus medius muscle and the lumbar and sacral spinous processes through the gluteal and lumbodorsal fascia (Koch, 1970), this will have a flexing effect on the back (tensing of the bow). Retraction of the forelimb will have a similar effect through increased forward traction on the spinous processes of the first few thoracic vertebrae that form the basis of the withers. The opposite movement of the limbs, that is, protraction of the forelimbs and retraction of the hindlimbs, will have an opposite effect on back motion and thus produce a hollow (extended) back. The last factor, which has been central to the recent discussions on training techniques for dressage horses, is the effect of head and neck position. Lowering of the neck will tense the nuchal ligament and exert a forward rotating moment on the spinous processes of T2–T6. These long spinous processes provide a big lever arm, and traction on them in the forward direction will provoke flexion of the back (tensing of the bow). Elevation of the head will produce the opposite effect (Figure 17-4). FIGURE 17-2 “Bow-and-string” concept of the back, according to Slijper. The vertebral column is the bow, the ventral musculature and the sternum are the string. The ribs, lateral abdominal musculature, spinous processes, and ligamentous connections are additional elements. (From Slijper EJ: Comparative biologic-anatomical investigations on the vertebral column and spinal musculature of mammals, Proc K Ned Acad Wetensch 42:1, 1946.) The bow-and-string model is at present considered by the scientific community as the best representation of the biomechanical concept of the equine back. It should be realized, however, that the model is an oversimplification of the real, highly complex situation. For instance, it does not account for the function of the pelvis and does not describe the interaction among hindlimbs, the pelvis, and the sacrolumbar spine (Haussler et al., 2007). Eadweard Muybridge and Etienne Marey (both 1830–1904) were, through the combination of their pioneering work on serial photography and their interest in horses, the founding fathers of what has been called the “first golden age of equine locomotion research” (van Weeren, 2001). In their wake, much research into many aspects of equine locomotion was initiated, using the novel techniques of photography and cine film, especially by the flourishing German veterinary anatomists in the era between the beginning of the twentieth century and World War II. Most of the work focused on limb motion (Schmaltz, 1922; Walter, 1925), but Wilhelm Krüger from Berlin dedicated part of his research effort to studying equine back motion (Krüger, 1939) and was also interested in the relative position of the center of gravity (Krüger, 1941). Krüger still held the traditionalist view of the back being a bridge spanning the gap between the limbs but was probably the first to perform in vivo kinematic research explicitly aiming at studying equine back motion, filming his subjects from above with help of a camera mounted on a tree. There was significant decline in equine kinematic research following World War II, until the start of what has been named the “second golden age of equine locomotion research” (van Weeren, 2001) in the early 1970s. Around that time, in Sweden, a novel technology from the aeronautics industry was introduced to study equine gait (Fredricson and Drevemo, 1971), but it took a while before interest in equine back motion resurged. First, a number of ex vivo studies using cadaver specimens were conducted in Canada (Townsend and Leach 1984; Townsend et al., 1983) and in France (Denoix, 1987; Denoix, 1992). These studies provided interesting and much-needed data on bony and ligamentous constraints on equine back motion (Figure 17-5), but these studies were not representative of the in vivo situation, as the specimens were isolated and the studies did not include muscular action. The motion of any rigid body in space can be described as the resultant of three translations and three rotations, respectively, around the three axes of a Cartesian orthogonal coordinate system that defines the space. The translations describe the progression of that rigid body within the space; the rotations describe positional changes of the body. For the description of back motion in relation to the rest of the animal, knowledge of the three rotations is sufficient, as rotation around the vertical axis (Z-axis) represents lateromotion or lateral bending (LB), rotation around the axis along the line of progression (described as Y-axis, or sometimes as X-axis) represents axial rotation (AR), and rotation around the remaining axis perpendicular to the sagittal plane (X-axis, sometimes designated as Y-axis) represents flexion–extension (FE) (Figure 17-6). In some publications, (aero)nautical terminology—pitch, yaw, and roll—is used for FE, LB, and AR, respectively. It should be realized that the motion of thoracolumbar vertebrae relative to each other is severely limited because of the anatomic constraints posed by the numerous ligaments connecting the vertebrae and the geometrical shape of the intervertebral joints (Denoix, 1999). For this reason, lateral bending cannot occur without a certain degree of axial rotation (Figure 17-7). The mutual dependency with respect to motion between the constituting elements of the thoracolumbar vertebral column is much larger than that between individual limb segments. Most of the currently used techniques for kinematic analysis rely on the use of skin markers for motion capture of segments of the body. Although convenient, the use of skin markers introduces an important source of error, which is the sliding of skin over skeletal structures, which are the real objects of study (Fick, 1910). When using skin markers for in vivo capture of spinal motion, this artefact is even more important than when studying limb motion because of the much smaller range of motion of the vertebral segments in comparison with limb segments and because of the intrinsic coupling of motion patterns as indicated above. The latter factor makes it impossible to quantify the degree of axial rotation and lateral bending underlying the displacement from the midline of a marker positioned on top of any of the thoracolumbar vertebrae. The skin displacement artefact in the limbs has been investigated by using invasive techniques to detect the position of the skin marker and underlying bone simultaneously (van Weeren and Barneveld, 1986; van den Bogert et al., 1990; van Weeren et al., 1990). For the accurate measurement of spinal motion in all three planes, a similar approach was used by Faber et al. (2000), who implanted Steinmann pins mounted with markers into the spinous processes of a number of thoracic and lumbar vertebrae. In this way, rigid bodies composed of the vertebra, the Steinmann pin, and the marker were created, which permitted drawing conclusions about vertebral motion based on motion capture of the markers. For data analysis, the method used allowed for the determination of three-dimensional spinal kinematics without defining a local coordinate system (Faber et al., 1999). Because the equine subject had been measured before surgery with skin markers fixed to the insertion sites of the pins, a program could be developed that could translate noninvasively collected skin marker data into motion patterns of the underlying vertebrae with reasonable accuracy and repeatability (Faber et al., 2002). This so-called Backin®1 program has been used in many more applied studies focusing on equine thoracolumbar kinematics, which will be discussed later in this chapter. Using the invasively obtained data, Faber calculated the three rotations describing spinal motion for the thoracic vertebrae T6, T10, T13, and T17 and the lumbar vertebrae L1, L3, L5, and S3 at the walk, trot, and canter (Faber et al., 2000; Faber et al., 2001a; Faber et al., 2001b). Motion patterns of all three basic rotations had a sinusoidal shape with FE showing two peaks, and LB and AR only one (Figure 17-8). This is caused by the fact that FE is induced by hindlimb motion and is identical (in symmetrically moving horses) for the left and right hindlimbs, whereas left and right hindlimb motion induces opposite patterns (clockwise and anti-clockwise, respectively) with respect to LB and AR, resulting in a single sinusoidal shape. In the method used by Faber et al. (2001c), the angle of a given vertebra is defined as the angle with a horizontal line through the marker of this vertebra that is parallel to the line connecting the markers of the two adjacent vertebrae, that is, the angle of L3 is defined by the line connecting L1 and L5. Outcome parameters of kinematic measurements of back motion are, thus, angular motion patterns (AMPs), which describe the thoracolumbar position, and also range of motion (ROM), which describes the extent of thoracolumbar motion in terms of FE, LB, and AR. As expected, thoracolumbar motion is limited compared with the motion patterns of most limb segments. Maximal ROM of FE is about 8 degrees at the walk, does not exceed 6 degrees at the trot, and is greatest at the canter with approximately 16 degrees (Faber et al., 2000; Faber et al., 2001a; Faber et al., 2001b). At the canter, most of the FE motion is found at the lumbosacral junction, although in vivo recorded motion is considerably less than the maximal ROM found in ex vivo experiments (Townsend et al., 1983). Figures that were a little lower but of the same order of magnitude were found by Haussler et al., who used a different invasive technique based on liquid metal strain gauges connecting the Steinmann pins inserted into the spinous processes of the vertebrae (Haussler et al., 2000; Haussler et al., 2001). In a more recent study, Johnson and Moore-Colyer (2009) determined the relationship of FE ROM at the lumbosacral junction with speed at the canter. They found a linear relationship, with FE ROM increasing from 6.1 ± 1.9 degrees at 6 ms-1 to 7.2 ± 1.9° at 8 ms-1. The ROM for LB is generally less than for FE. At the walk, maximal values of approximately 5 degrees were found that reduced to 1.9 to 3.6 degrees at the trot and were 5.2 ± 0.7 degrees at the canter (Faber et al., 2000; Faber et al., 2001a; Faber et al., 2001b). Axial rotation, for the calculation of which a reference line through the markers on both tubera coxae was used, varied at the walk between 4 degrees (T6) to 12 degrees (S3) and was 3.1 to 5.8 degrees at the trot and 7.8 ± 1.2 degrees at the canter (Faber et al., 2000; Faber et al., 2001a; Faber et al., 2001b). From these figures, it is clear that the trot is the gait at which the back is held most rigid in all possible directions of motion. Variability in back motion is considerable, with within-horse variability ranging from 6% for AR at the walk to 7.8% to 18.2% for LB and also at the walk. Between-horse variability was highest for LB as well, and between-horse variability was four to five times higher compared with within-horse variability; for FE and AR, this was two to three times higher (Faber et al., 2001a; Faber et al., 2001b). The high variability in back motion should be taken into account when assessing the effects of interventions on individual horses and also when judging back motion in horses that are presented for the first time with suspected back dysfunction.
Kinematics of the equine back and pelvis
Biomechanical concept of the quadrupedal back
Research leading to current analytical techniques for equine thoracolumbar kinematics
Basic kinematics
Thoracolumbar motion in the horse
Stay updated, free articles. Join our Telegram channel
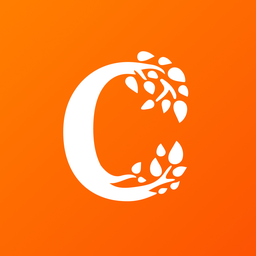
Full access? Get Clinical Tree
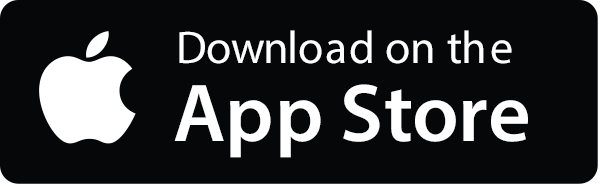
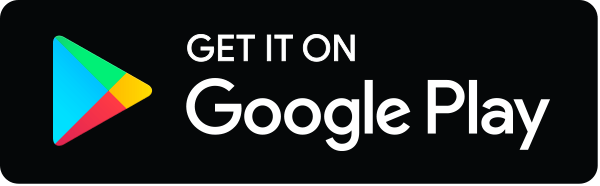