Chapter 16 Kidney Function and Damage II. KIDNEY MORPHOLOGY AND FUNCTION A. General Structure of the Kidneys C. Tubule: Reabsorption and Secretion A. Indirect Tests of Glomerular Function B. Direct Tests of Glomerular Function V. BIOCHEMICAL CHANGES IN KIDNEY DISEASE Clinical biochemistry in nephrology is mainly used to diagnose and monitor renal dysfunction or damage. This is of special importance in human medicine, because of the frequent observation of renal failure in elderly people, and in canine and feline medicine, especially for the early detection of chronic renal failure (CRF), which is frequent. In U.S. private practice, however, renal disease was not reported as one of the 29 most common disorders in dogs and was ranked 17th in cats (Lund et al., 1999), and in a survey of Australian practices, the expected frequency of renal failure ranged from one case per week to one case every 2 weeks (Watson et al., 2001). A British survey indicated that 0.2% of dogs were presented with suspected renal disease, which was confirmed in 25% of the cases with no evidence of breed or sex predisposition (MacDougall et al., 1986). The reported prevalence of CRF in a U.S. university hospital was three times higher in cats than in dogs (Polzin et al., 1992). However, the frequency of renal disease increases with age, especially in dogs and cats, as in humans (Cowgill and Spangler, 1981; Gobar et al., 1998; McCall Kaufman, 1984). In U.S. veterinary university hospitals, renal disease was observed in 15% of dogs more than 10 years old, in 33% of cats more than 15 years old (Polzin et al., 1989), in 3% of cats aged 7 to 10 years, and in 30% of cats more than 15 years old (Krawiec and Gelberg, 1989). Kidney diseases are uncommon in equids and cattle (Fetcher, 1985). The latter show better resistance to a loss of kidney function than monogastric animals because of the filtration function of the rumen epithelium as shown by bilateral nephrectomy (Fetcher, 1986). The mammalian kidney consists of tens of thousands to millions of nephrons that function as parallel units. The larger the species, the greater the number of nephrons per kidney (Kunkel, 1930; Rytand, 1937–1938; Vimtrup, 1928). This ranges from about 10,000 in mice (Cullen-McEwen et al., 2003), 175,000 in cats (Brown et al., 1993), 300 to 700,000 in dogs (Finco and Duncan, 1972), and 7 million in elephants, as compared to about 1 million in humans. The number of nephrons progressively increases during fetal development and is complete at birth (e.g., in sheep or humans) or during the few days following birth (e.g., in rat) (Gimonet et al., 1998). The number of nephrons in the dog decreases slightly (5%) during the 2 first months of life, whereas the glomerular volume increases by 33% (Horster et al., 1971). However, great interindividual variability is observed within species. In dogs, the size or the weight has little influence on the number of glomeruli, but the size of these latter is larger in larger breeds (Finco and Duncan, 1972; Kunkel, 1930). In sheep, the number of nephrons in twins was about 30% lower than in single lambs (Mitchell et al., 2004) or showed no difference (Bains et al., 1996). FIGURE 16-1 Schematic representation and international nomenclature of the parts of the nephron. 1: Renal corpuscle including Bowman’s capsule and the glomerulus (glomerular tuft). 2: Proximal convoluted tubule. 3: Proximal straight tubule. 4: Descending thin limb. 5: Ascending thin limb. 6: Distal straight tubule (thick ascending limb). 7: Macula densa located within the final portion of the thick ascending limb. 8: Distal convoluted tubule. 9: Connecting tubule; 9*: Connecting tubule of the juxtamedullary nephron that forms an arcade. 10: Cortical collecting duct. 11: Outer medullary collecting duct. 12: Inner medullary collecting duct. Reproduced with permission of the publisher from Kriz and Bankir (1988). The general architecture of the nephrons is identical in all species (Fig. 16-1) (Kriz and Bankir, 1988), but their disposition within the kidney differs between species (Bankir and de Rouffignac, 1985). Blood is supplied to the kidneys by the renal arteries. These divide into interlobar and arcuate arteries located at the corticomedullary junction. Branches of the latter supply blood to the afferent arterioles of the tuft of capillaries in the glomerulus, from which it is collected by the efferent arterioles. Depending on the location of the glomeruli, blood is then supplied to a network perfusing the cortical tubules or to the vasa recta vessels, which penetrate deep into the medulla in “hairpins” parallel to the loops of Henle. See the review in Pallone et al. (1998). The total blood supply to the kidneys (renal blood flow, RBF) is very high, about 20% of the cardiac output, and most of it goes to the cortex. Only a fraction of the plasma flow (renal plasma flow, RPF) is filtered resulting in the glomerular filtration rate (GFR). This is the filtration fraction (FF), which generally amounts to 20% to 30% of RPF: GFR = RPF × FF. FIGURE 16-2 Electron micrograph of the glomerular filtration barrier. 1: Footlike processes of the podocytes; arrow shows the slit diaphragm of a filtration slit. 2: glomerular basement membrane. 3: fenestrated endothelium of the glomerular capillaries. The RBF remains quite stable, because of autoregulation, even with variations in systemic blood pressure. The precise mechanism of autoregulation is unknown but results from vasoconstriction/dilation of the afferent and efferent glomerular arterioles, which maintains an almost constant hydrostatic pressure within the glomerulus. Autoregulation is efficient in healthy dogs between 70 to 180 mmHg and may be altered in disease, especially during CRF (Brown et al., 1995). Each glomerulus consists of a tuft of anastomosed capillaries within the Bowman’s capsule, which collects the primitive urine formed by plasma filtration and opens into the tubule conducting urine to the renal pelvis. The filter between the plasma and urine consists of three layers (Fig. 16-2) (see reviews in Deen et al. [2001], and Rennke and Venkatachalam [1977]): • The fenestrated endothelium of the capillaries, allowing direct contact between the plasma and the basal membrane via large pores and fenestrae (~50 to 100 nm); the luminal face is coated with sulfated glycosaminoglycans and glycoproteins. • The basement membrane made of a gel containing approximately 90% water and negatively charged sulfated glycosaminoglycans. • The filtration slits (~25 nm) between the footlike processes of the podocytes (i.e., the visceral epithelial cells covering the external surface of the capillaries) (Gubler, 2003). The slits are made porous by the slit diaphragm in which proteins, consisting mainly of the extracellular part of the nephrin molecule anchored in the membrane of the podocytes, are arranged in zipper-like fashion (Wartiovaara et al., 2004). This slit diaphragm seems to be the basis of filter selectivity, although this has long been considered a function of the basement membrane; it may also act as a signaling system to modulate filtration (Benzing, 2004). FIGURE 16-3 Pressures involved in glomerular filtration within a glomerular capillary. The driving force is the hydrostatic pressure in the capillary (Phc), which is opposed by hydrostatic pressure within Bowman’s space (Phb) and oncotic pressure in the capillary (Po). Values are pressures in dogs and cats. The driving force of filtration is hydrostatic pressure of cardiac origin (Fig. 16-3). This is opposed by plasma colloidal (oncotic) pressure produced by plasma proteins and urine hydrostatic pressure within the Bowman’s capsule. The colloidal pressure in the Bowman’s capsule is negligible because of the almost total absence of proteins. As a result, the effective filtration pressure is 10 to 15 mmHg (Navar et al., 1977). The limits of filtration (see the review in Tryggvason, 1999) are as follows: • Size and shape: Neutral molecules with a diameter <2.5 nm diffuse freely. Then as diameter increases, filtration decreases to approximately 0 when the diameter is >3.5 nm (i.e., the approximate diameter of the albumin molecule). • Charge: Because of the high concentration of sulfated glycosaminoglycans at the surface of endothelial cells and in the GBM, the filtration slit tends to repel negatively charged molecules (i.e., most plasma proteins at blood pH). As a result of glomerular filtration, all small hydrosoluble plasma molecules, including water and ions, are freely filtered but high molecular weight proteins are not. Albumin, which has an MW ~67,000 and a pI = 4.9 (Purtell et al., 1979), is very close to the limit of filtration so that only a minimal amount is filtered by “normal” kidneys. The albumin concentration in primitive urine is ~20 to 30 mg/l and many smaller proteins are also present, most of which are reabsorbed in the tubule. The tubule is divided into different segments (Fig. 16-2) (see standard nomenclature in Kriz and Bankir, 1988): • The proximal tubule begins with a convoluted portion followed by a straight section dipping toward the medulla; the epithelium of the proximal tubule consists of thick cuboidal cells with a very dense brush border on the luminal side, which provides an immense surface of exchange with the glomerular urine; this is the portion of the nephron where most solutes and water are reabsorbed. • The loop of Henle produces a “hairpin” bend within the medulla, ending close to the glomerulus at the juxtaglomerular apparatus. Long and short loops descend only into the inner or outer medulla, respectively. The loop of Henle is essential to urine concentration mechanism and it is often stated that long loops are mostly observed in species living in desert areas, and thus related to higher concentrating ability. Almost all the nephrons in dogs and cats are long looped (Bulger et al., 1986), whereas those in humans and pigs are mostly short looped (Bankir and de Rouffignac, 1985), and the average urines are more concentrated in dogs and cats than in humans or swine. The two main points regarding function are that (1) the water and urea permeability of the descending thin limb is high, because of the presence of the aquaporin 1 water channel and (2) the ascending thin limb shows very low water and high sodium chloride permeability. • The juxtaglomerular apparatus is a morphological entity at the confluence of the afferent and efferent arterioles of the glomerulus and a differentiated part of the loop called the macula densa (Spangler, 1979a, 1979b). The cells in the macula densa respond to decreases in blood pressure or hyponatremia by secreting renin stored in the granules, thus activating the angiotensin-aldosterone response. • The distal convoluted tubule stretches from the macula densa to the confluence into a collecting tubule within the cortex. The reabsorption capacity is lower than in the proximal nephron (e.g., only ~5% to 10% of sodium and chloride), and secretion of potassium may occur. See the review in Reilly and Ellison (2000). • The collecting tubules leading to the renal pelvis. The final regulation of urine volume and solute excretion occurs in the final segment of the distal tubule and the collecting tubule, and it is partly regulated by hormones. The main tubule functions are the reabsorption of water, electrolytes, and small molecules and, to a lesser extent, the secretion of ions and small molecules. Reabsorption is dominant in healthy animals and mainly occurs in the proximal tubule by active and passive transport. Further adjustment of urine excretion occurs in the distal tubule and is controlled by hormones, so that the final urine is usually more concentrated than the ultrafiltrate. See the review in Reilly and Ellison (2000). Reabsorption and secretion continually adapt to maintain an almost constant plasma composition, whereas the intake and utilization of ions and small molecules vary with food and water supply, proximity of meals, environment, physical effort, and so on. This is why the following occurs: • Urine composition can show large variations in the same healthy or diseased subject. • A notable overlap of urinary analyte concentrations is observed in healthy and diseased animals. • The reference intervals for urine analytes are of little relevance when determining spot urine compositions. a. Small Hydrophilic Molecules Glucose, amino acids, and low-molecular-weight proteins are mostly reabsorbed in the proximal tubule. Almost 100% of the glucose, amino acids, and proteins are reabsorbed. The former return to the plasma, whereas small proteins are degraded in the tubular cells. Other small hydrophilic molecules are not or only poorly reabsorbed (e.g., creatinine) (see Section II.A.1.a). Glucose reabsorption is permitted by the SGLT1 (sodium glucose transporter) in the apical membrane, which couples glucose reabsorption with sodium transport down a gradient produced by an Na/K-ATPase in the basolateral membrane, across which glucose diffuses in a concentration gradient by means of GLUT-2 transporters. This glucose reabsorption capacity is limited by the finite number of transporters: maximal tubular reabsorption (Tm) is attained when plasma glucose concentration (P-Glucose) is about 12 mmol/l in dogs, 15 to 18 mmol/l in cats, and 8 to 10 mmol/l in cows. No urea reabsorption occurs before the medullary part of the collecting duct because of the presence of urea transporters activated by antidiuretic hormone (ADH). This is part of the mechanism creating a high inner medullary osmolality. A small amount of urea is even secreted into the medullary part of the ascending branch of the loop of Henle. Urea reabsorption is increased when urine flow is low (e.g., during dehydration or volume depletion). Urea is central to the concentrating mechanism in the kidney and is ensured by the presence of urea transporters at the thin extremity of the descending loop of Henle and corresponding segments of the vasa recta, which enable urea diffusing from the medullary part of the collecting tubule to enter the nephron and to a lesser degree the blood. See the review on urea transporters in Smith and Rousselet (2001). b. Electrolytes Electrolytes are mostly reabsorbed in the proximal tubule. The rate of reabsorption differs considerably according to the internal balance of each ion. Under “normal” conditions it is almost 100% for sodium, chloride, calcium, and phosphates, but much lower for potassium, especially in ruminants owing to their high dietary intake. The extent of reabsorption can be estimated from the fractional excretion (FE) of solutes (i.e., the fraction of the filtered load of an electrolyte that is finally excreted in urine) (see Section II.C.3): • Sodium concentration is kept low in tubule cells, as in other cells, by an Na/K-ATPase in the basolateral membrane. See the review in Feraille and Doucet (2001). This active transport of sodium ions from the urine accounts for most sodium reabsorption. It also creates a sodium concentration gradient that allows cotransport of amino acids, glucose and other ions, and so on. See the review in De Weer (1992). Further reabsorption of sodium and chloride occurs in the ascending branch of the loop of Henle via an Na-K-2Cl cotransporter in the luminal membrane. See the review in Russell (2000). Final adjustment in the distal part of the nephron is hormonally controlled by aldosterone and natriuretic peptides. • Chloride is the most abundant anion in the extracellular compartment. The plasma concentration and elimination of chloride are usually concomitant with those of sodium, except in the case of acid-base disorders. In metabolic acidosis, bicarbonate ions secreted by the kidney cells are exchanged with chloride by an Na-independent Cl/HCO3 exchanger, thus increasing urine chloride elimination and the plasma anion gap. • Potassium is reabsorbed in the proximal tubule (~70%) and in the ascending part of the loop of Henle, the distal tubule, and the medullary collecting duct. Potassium is also secreted by the distal tubule and cortical collecting duct, mainly during hyperkalemia (Berliner and Kennedy, 1948). See the review in Hebert et al. (2005). Part of this process is based on aldosterone secretion, which induces the synthesis of Na/K-ATPases, thereby favoring potassium excretion and sodium reabsorption. • The inorganic phosphates (Pi) in plasma comprise about 80% HPO42– and 20% • Calcium: Free and complexed calcium ions are freely filtered by the glomerulus. Calcium is mostly reabsorbed in the proximal tubule and in the ascending branch of the loop of Henle, except in horse and rabbit, which excrete significant amounts of this ion when supply is sufficient. Reabsorption occurs by paracellular route. Epithelial channels in the distal part of the nephron permit the transcellular transport of about 15% of filtered calcium. See the reviews in Hoenderop and Bindels (2005)and Hoenderop et al. (2005). This final reabsorption is mainly under the hormonal control of PTH. • Magnesium: Non-protein-bound magnesium is filtered by the glomerulus. Only about 25% are reabsorbed in the proximal tubule. Most reabsorption occurs in the ascending branch of the loop of Henle (50% to 60%) (Rosol and Capen, 1996). c. Water Most water (~75%) is reabsorbed passively in the proximal tubule along with ions and small hydrophilic molecules, so that the fluid entering the descending branch is isotonic. Water is reabsorbed in the descending branch of Henle’s loop as a result of the corticopapillary osmolar gradient, whereas the ascending branch is impermeable to water. Final reabsorption occurs in the collecting tubule, mainly under the hormonal control of the antidiuretic hormone (ADH). This hypothalamic nonapeptide is secreted by the posthypophysis when osmolality increases and during hypovolemia or decreased blood pressure. Receptors in the collecting tubule cells trigger the AMPc-dependent synthesis and shuttling of aquaporins, mainly the AQP2 water channel, into the apical and basolateral membrane, thereby permitting water reabsorption and the final adjustment of urine concentration. See the review in Nielsen et al. (2002). The final urine concentration is usually much greater than that of the glomerular filtrate (Fig. 16-4). d. Acid-Base Regulation The main organs involved in acid-base regulation are the kidneys and lungs. The lungs modulate the elimination of CO2 and the kidneys the elimination of protons and generation of bicarbonate ions. The carbonic acid (H2CO3)-bicarbonate ( Bicarbonate ions filtered by the glomerulus are mainly reabsorbed in the proximal tubule (~80%) as CO2, which is lipophilic and able to diffuse across the membrane. Within the cell, CO2 is hydrated by carbonic anhydrase into carbonic acid, which dissociates into bicarbonate ions and protons. The secretion of protons into the tubule lumen ensures the conservation of bicarbonate and its transfer to the plasma. This occurs principally in the distal tubule, where protons are excreted and buffered in urine with filtered organic anions, phosphate, and ammonia generated from glutamine in the tubule cells by the action of glutaminase and glutamate dehydrogenase. This process is enhanced during acidosis, when distal tubule and collecting tubule cells excrete protons against a concentration gradient by the action of an H+-ATPase in the basolateral membrane. FIGURE 16-4 Water reabsorption in the kidney tubule (values are osmolalities). The corticomedullary osmotic gradient results from reabsorption of sodium and chloride without concomitant reabsorption of water in the ascending limb of the loop of Henle and from urea reabsorption in the medullary part of the collecting ducts. Owing to water permeability of the descending limb of the loop of Henle plasma isoosmotic urine flowing from the proximal tubule is first concentrated in the descending branch of the loop of Henle, then is diluted by escape of NaCl and water retention in the ascending branch, and finally is concentrated along the collecting ducts. Reproduced with permission of the publisher from Finco (1995b). e. Endocrine Functions Two major hormones, erythropoietin (EPO) and 1α, 25-dihydroxycholecalciferol (calcitriol), are synthesized by the kidneys and released into the blood. EPO is a cytokine that regulates erythrocyte production synthesized in the peritubular cells in response to hypoxia. Minor amounts are also produced in the liver, mainly in the newborn. EPO binds to receptors of bone marrow progenitor cells and acts synergistically with other growth factors to proliferate and mature the erythroid progenitor cells. In advanced chronic renal disease, the synthesis of EPO decreases and is insufficient to meet the demands for new red cell production, resulting in anemia. See the review in Fisher (2003). Calcitriol is a secoBsteroid hormone derived from vitamin D3. It is produced in the proximal tubule cells by the action of 1α-hydroxylase on 25-hydroxyvitamin D3 produced by liver hydroxylation of vitamin D3. Calcitriol is a major antihypocalcemic hormone acting at the transcriptional level to induce active intestinal absorption of calcium. It acts in synergy with PTH to activate calcium release from bone. PTH increases the expression and activity of 1α-hydroxylase activity during calcium and vitamin D3 deficiency. The direct modulating effects of calcium and phosphates are weaker. 1α-hydroxylase activity was recently shown to be down-regulated by phosphaturic peptides called phosphatonins. See the reviews in Ebert et al. (2006), Jones et al. (1998), and Kumar (1984). Calcitriol synthesis is decreased in CRF, and its administration is recommended for the treatment of animals with CRF and concomitant hyperparathyroidism. See the review in Nagode et al. (1996). Kidney function can be evaluated from the concentrations of plasma or urine analytes, which are mainly dependent on their elimination (e.g., P-Creatinine). These indirect markers can be easily and rapidly measured, but their sensitivity is poor and generally remains unaltered until 75% of renal function has been lost and their concentrations may be modified by extrarenal factors. Direct tests of kidney function are based on the elimination kinetics of markers of glomerular filtration, blood flow, or tubule reabsorption/secretion and are based on the clearance concept. These tests are more difficult and take longer to perform but allow earlier detection of reduced function. P-Creatinine is the test most often used to diagnose and monitor kidney disease in human and animal clinical pathology. P-Urea is also used frequently but is subject to more numerous extrarenal factors of variation. These molecules are almost totally eliminated by glomerular filtration, so that in the case of kidney failure their plasma concentration increases. However, neither test is sensitive in the early diagnosis of kidney disease because of the large functional reserve of the kidneys. Moreover, variations of P-Urea and P-Creatinine are not proportional to the number of functional nephrons (e.g., a mean increase of 85% in P-Creatinine and of 140% in P-Urea was observed after a two-fold reduction of GFR, with values close to the upper limit of the reference interval) (Lefebvre et al., 1999). a. Creatinine Metabolism Creatinine is a small molecule (MW 113) produced by degradation of creatine and creatine-phosphate, an energy-storing molecule mainly present in skeletal muscles. See the reviews in Braun et al. (2003), Perrone et al. (1992), and Wyss and Kaddurah-Daouk (2000). Creatine is synthesized from the amino acids glycine, arginine, and methionine, the final step occurring in the liver (Fig. 16-5). It is then taken up by the muscles where it is reversibly phosphorylated by creatine-kinase into creatine-phosphate. Skeletal muscles contain about 95% of the total body creatine and creatine-phosphate pool. The estimated turnover of creatine-phosphate (about 2%) is fairly constant in a given individual. The resulting estimated daily input of endogenous creatinine into plasma was 380 ± 45 μmol/kg BW in healthy beagles and 10% to 20% lower in animals with reduced kidney mass (Watson et al., 2002a). In carnivores and omnivores, creatinine can also originate from the creatine and creatinine in food (Harris and Lowe, 1995; Harris et al., 1997). FIGURE 16-5 Schematic representation of creatinine metabolism. The first step is the transamidination of arginine and glycine yielding guanidinoacetic acid (GAA) by the kidney enzyme arginine: glycine amidinotransferase. In the liver, N-methylation of guanidinoacetate into creatine is catalyzed by guanidinoacetate methyltransferase, using methyl groups donated by S-adenosylmethionine. Creatine is distributed to muscle cells where it is reversibly phosphorylated to creatine phosphate by creatine kinase. Creatinine is the product of the spontaneous, irreversible, non-enzymatic, internal dehydration of creatine, and dephosphorylation of creatine phosphate. Creatinine mainly circulates in a free form in the plasma and is distributed into the whole body water compartment (Schloerb, 1960; Watson et al., 2002a). It was reported that, in dog plasma, 6% were bound to plasma proteins (Kennedy et al., 1952). Creatinine is freely filtered by the glomerulus and is not reabsorbed or secreted in cats (Finco and Barsanti, 1982) and ponies (Finco and Groves, 1985), but it may be strongly secreted in horses (Bickhardt et al., 1996). In dogs, either no secretion has been observed (Finco et al., 1993; Watson et al., 2002a) or very weak proximal tubule secretion has been reported in males but not in females (O’Connell et al., 1962; Swanson and Hakim, 1962). This secretion is slightly increased following reduction of the renal mass (Robinson et al., 1974). Secretion of creatinine by active transport in the proximal tubule has been reported in humans, in whom it is nonuniformly increased in renal failure (Walser et al., 1988), also in sheep (Bickhardt and Dungelhoef, 1994), rabbit (Matos et al., 1998), pig (Wendt et al., 1990), and goat (Ladd et al., 1957). Creatinine is reabsorbed by the tubules in newborn rabbits and humans, probably by back-leak through the immature tubules (Matos et al., 1998). As observed in humans and rats, extrarenal intestinal catabolism may be suspected in cases of renal failure. This may involve the bacterial flora, as demonstrated in the rat by accumulation creatine bacterial catabolites including methylguanidine (Jones and Burnett, 1972; Mitch et al., 1980). This hypothesis is supported by the observed increase in cats with ARF (Ohashi et al., 1995). Finally, creatinine is rapidly cleared from plasma (half-life of 3 h in dogs; Watson et al., 2002a) and eliminated in urine, where its total excretion depends on body weight (Gartner et al., 1987). b. Preanalytical Factors of Variation • Specimen: Serum and plasma creatinine concentrations are identical, although a slightly higher serum value has been reported in dogs (Thoresen et al., 1992). Differences between jugular and cephalic vein may be overlooked (5 μmol/l in dogs) (Jensen et al., 1994). Canine P-Creatinine is not changed by storage at –20°C or –70°C for up to 8 months (Thoresen et al., 1995) or by three freeze-thaw cycles (Reynolds et al., 2006). In human urines, the creatinine concentration decreased by about 20% to 30% in 1 week at any temperature between 4°C and –80°C, and was not altered by five freeze-thaw cycles (Schneider et al., 2002), whereas others found that it was stable up to 30 days when stored at 4°C and minimally decreased at 25°C (Spierto et al., 1997). • Diet and meals: P-Creatinine in dogs and cats was increased by meals containing meat, especially when this was cooked (Epstein et al., 1984; Evans, 1987; Harris and Lowe, 1995; Lowe et al., 1998; Sagawa et al., 1995; Watson and Church, 1980; Watson et al., 1981) or after oral loading with creatine (Lowe et al., 1998), although large interindividual differences were observed. P-Creatinine was higher in dogs fed chicken-based diets than egg- or casein-based diets (Bartges et al., 1995a). No differences in P-Creatinine were observed between cats receiving high- or low-protein diets (Adams et al., 1993). P-Creatinine was moderately higher in young dogs on a low-salt diet (Bagby and Fuchs, 1989) and about 50% higher in goats fed on a low-protein diet (Valtonen et al., 1982). • Hydration status: P-Creatinine was only moderately increased in dogs deprived of water for 4 days, even when the weight loss was ≥10% (English et al., 1980; Hardy and Osborne, 1979). • Physical exercise: P-Creatinine was decreased (by about 40%) by physical training in sled dogs (Kronfeld et al., 1977; Querengaesser et al., 1994). It was not significantly changed after strenuous physical exercise in untrained dogs (Chanoit et al., 2002). It was increased by about 20 μmol/l after a 400 m sprint in greyhounds (Rose and Bloomberg, 1989; Snow et al., 1988) and by approximately 50% after strenuous sprint efforts in sled dogs (Hammel et al., 1997; Querengaesser et al., 1994), but not after very long races (up to 415 miles) (Hinchcliff et al., 1993; Querengaesser et al., 1994). • Housing: P-Creatinine was slightly higher (10 to 20 μmol/l) in dogs kept indoors than outdoors (Kuhn and Hardegg, 1988; Rautenbach, 1988), whereas others found no difference (Spangenberg et al., 2006). • Drugs: P-Creatinine was decreased in dogs receiving glucocorticoids (Braun et al., 1981), whereas U-Creatinine was increased (Iversen et al., 1997). P-Creatinine was unchanged or little affected by a single dose of nonsteroidal anti-inflammatory drugs (NSAID) (Lobetti and Joubert, 2000; Mathews et al., 2001) or by halothane anesthesia (Lobetti and Lambrechts, 2000), and moderately increased by furosemide administration (Adin et al., 2003) and high-dose trimethoprim-sulfadiazine in dogs (Lording and Bellamy, 1978). P-Creatinine could increase (Kitagawa et al., 1997), remain unchanged (Atkins et al., 2002), or decrease (Pouchelon et al., 2004) in dogs treated with ACE inhibitors for heart failure. c. Analytical Factors of Variation HPLC is considered to be the reference method (Blijenberg et al., 1994; Hanser et al., 2001), but routine analyses are based on the nonspecific Jaffé reaction (alkaline picrate) and enzymatic procedures (Guder et al., 1986). See the reviews on creatinine measurement in Spencer (1986) and recommendations for improvement in Myers et al., (2006). The enzymatic methods give slightly lower results than HPLC and Jaffé reaction, ~5 and 20 μmol/l, respectively, in dog plasma (Evans, 1987; Palm and Lundblad, 2005). The main interferents in the Jaffé reaction are glucose, ketones, and hemoglobin, but canine plasma was unaffected by hemolysis up to 25 g/l (Jacobs et al., 1991, 1992; O’Neill and Feldman, 1989). Interference in the kinetic Jaffé technique is limited in normal dog plasma as the other chromogens react more slowly than creatinine (Palm and Lundblad, 2005). There is less interference in urine because of the lower proportion of Jaffé chromogens, so that calculated creatinine clearances may differ greatly according to the technique used, as shown in rats (Jung et al., 1987). The accuracy of plasma creatinine measurement is far from satisfactory in human medicine and cannot be expected to be better in veterinary medicine (Miller et al., 2005; Myers et al., 2006). The measurement of urine creatinine has not been validated in animals, but a poor correlation between different techniques was shown in dogs, especially in concentrated urines (Trumel et al., 2004). d. Reference Intervals and Physiological Factors of Variation Reference intervals for P-Creatinine have been poorly defined in most species because of animal selection and the chosen analytical method. The reference intervals for dogs were shown to differ considerably from one textbook to another (Lefebvre et al., 1998b), so that any interchanging of reference limits or decision levels would be risky: • Breed: P-Creatinine is higher in large breeds of dogs (Braun et al., 2002; Feeman et al., 2003; Hilppo, 1986; Medaille et al., 2004), even in puppies (Kühl et al., 2000). • Gender: No differences related to gender were observed in dogs (Broulet et al., 1986; Passing, 1981), although moderately higher concentrations (~ + 10%) were observed in 1- to 3-year-old and 9- to 11-year-old male beagles (Fukuda et al., 1989). • Age: A high P-Creatinine concentration was reported in newborn calves, puppies, and foals, probably because of the accumulation of creatinine ingested from the allantoic fluid (9 to 23 mmol/l in bovines) (Edwards et al., 1990; Klee et al., 1985; Kühl et al., 2000; Lupke et al., 1967). This decreased during the following weeks and then showed a moderate increase again. In dogs and cats, P-Creatinine almost doubled from the first weeks to 1 year of age, then remained stable up to 10 years (Kraft et al., 1996; Passing and Brunk, 1981; Strasser et al., 1993, 1997; Swanson et al., 2004; Vajdovich et al., 1997; Wolford et al., 1988), although other authors reported a regular decrease with age in dogs (Fukuda et al., 1989; Lowseth et al., 1990). In piglets, P-Creatinine almost doubled between 2 and 6 months of age (Krogh et al., 1979). • Biological rhythms: Both a circadian and a seasonal rhythm were observed in dogs, but they were of limited significance (Singer and Kraft, 1988; Sothern et al., 1993; Strasser et al., 2001), whereas no such rhythms were reported in bulls (Boehnke, 1980). In healthy sheep, creatinine was 25% higher in summer than in winter (Nawaz and Shah, 1984). e. Variations of P-Creatinine in Disease An inverse curvilinear relationship was observed between P-Creatinine and GFR in dogs (Fig. 16-6) (Finco et al., 1995, 1993; Miyamoto, 2001a; Westhoff et al., 1993). This was the same in both sexes (Finco et al., 1995). The assessment of GFR from creatinine is hazardous because of interindividual variability (e.g., P-Creatinine = 200 μmol/l could be observed in dogs with GFR ranging from 0.4 to 1.4 ml/min/kg) (Finco et al., 1995). In cats the relationship is almost linear (Haller et al., 2003). FIGURE 16-6 Relationship between P-creatinine and GFR in dogs. Observe that (1) on the right-hand part of the graph, GFR can be decreased from about 6 to 2 ml/min/kg without any significant change of P-creatinine; (2) on the left-hand part of the graph, dramatic reductions of P-creatinine, as observed during animal rehydration in clinics, do not imply a significant increase in GFR. Reproduced with permission of the publisher from Finco (1995a). The sensitivity and specificity of P-Creatinine for the diagnosis of CRF are not very high in dogs and neither are the predictive values (Braun and Lefebvre, 2005; Gleadhill, 1994). The critical difference for P-Creatinine in dogs is 35 μmol/l (Jensen and Aaes, 1993). These criteria have not been reported for other species. It was suggested that the evolution of renal disease in dogs could be monitored by repeated creatinine measurement and that the time of death could be predicted from the 1/P-Creatinine versus the time curve (Allen et al., 1987); however, this approach gave false estimates in humans (Walser et al., 1988). P-Creatinine is the most efficient indirect marker of GFR in mammals. It is increased in chronic and acute renal failure, and also in some conditions not directly involving the kidney. For example, see the review as it pertains to dogs in Table 16-1 and in Braun et al. (2003). Similar variations were also observed in cats, equines, and bovines. a. Urea Metabolism Urea is a small hydrosoluble molecule (MW 60) synthesized in the liver from bicarbonate and ammonium in the Krebs-Henseleit cycle. Urea is the main form in which nitrogen is eliminated in mammals. After synthesis, it is distributed into the total body water compartment (Dunegan et al., 1978; Schloerb, 1960). It is freely filtered by the kidney glomeruli and reabsorbed from the collecting tubule. Its passive reabsorption is increased when urine flow in the tubule is reduced (Park and Rabinowitz, 1969), which can lead to increased P-Urea in dehydrated patients or in patients with hemorrhage or to decreased P-Urea in overhydrated patients. Some urea also filters into the intestine, where it is degraded by bacteria into ammonium, which is absorbed and provides a notable proportion of the ammonium supply to the liver. Another important source of ammonium is the catabolism of amino acids. Proteins are thus a major source of ammonium for urea synthesis. Intense recycling of urea occurs in ruminants by transfer to the gastrointestinal tract and to saliva. Urea can also be added to ruminant food (Cirio et al., 2000; Marini and Van Amburgh, 2003), whence it is incorporated into bacterial proteins. The dietary supply of urea is low in other species. b. Preanalytical Factors of Variation • Specimen: No difference between canine serum and heparin plasma was observed and only minor changes were noted when specimens were stored frozen for up to 8 months (Thoresen et al., 1995). P-Urea is little affected by hemolysis (up to 25 g hemoglobin/l) and icterus in cattle, horses, cats, or dogs; it is decreased by lipemia in dogs (Jacobs et al., 1992; O’Neill and Feldman, 1989). P-Urea is stable in plasma and whole blood stored for up to 3 days at 20°C (Thoresen et al., 1992) and in serum or plasma stored frozen at –20°C and at –70°C up to 8 months (Thoresen et al., 1995). • Diet and meals: P-Urea is increased in dogs after meals. Peak postprandial increase can be as high as 7 mmol/l about 6 h after the meal and last for more than 18 h; it is greater with high-protein diets or in animals fed large amounts (Anderson and Edney, 1969; Epstein et al., 1984; Evans, 1987; Vogin et al., 1967). In most species, basal P-Urea reflects the balance between nitrogen utilization and excretion and can be greatly influenced by nutrition (Kohn et al., 2005). The fasting concentration of P-Urea was lower in dogs on low-protein diets with normal or reduced renal function (Polzin et al., 1983, 1991; Reynolds et al., 1999), in horses (Doreau and Martin-Rosset, 1985), in sheep (Rabinowitz et al., 1973), in goats (Valtonen et al., 1982), and in cats (Hesta et al., 2005). P-Urea was also increased by prolonged fasting, because of catabolism of body proteins (Rabinowitz et al., 1973). • Hydration status: Dehydration had little effect on P-Urea in dogs (≤12.5 mmol/l in 4 days, with weight loss up to 16%) (Hardy and Osborne, 1979), but it produced a two-fold increase in calves within 4 days (Bianca et al., 1965). • Drugs: P-Urea was unchanged by halothane anesthesia in dogs (Lobetti and Lambrechts, 2000) and was increased at high doses of trimethoprim-sulfadiazine (Lording and Bellamy, 1978). • Physical exercise: P-Urea in sled dogs was higher after 12 weeks of training, probably as a result of increased protein intake (Reynolds et al., 1999). In greyhounds, P-Urea was unchanged by a 235 m sprint and moderately increased 30 min after a 420 m run (Snow et al., 1988). c. Analytical Factors of Variation Most techniques are based on the specific action of a bacterial urease. The accuracy of P-Urea measurements is not usually reported. d. Reference Intervals and Physiological Factors of Variation 1 mmol/l = 6 mg/dl. There is no good reason to continue using BUN, as the analytical procedure was abandoned long ago and is merely a source of confusion. If required, the factors are BUN (mg/dl) × 0.356 = Urea (mmol/l), and BUN (mg/dl) × 21.4 = Urea (mg/l). • Gender: No significant effect of gender was observed in young beagles (Passing and Brunk, 1981) or in adult dogs (Broulet et al., 1986). • Age: P-Urea decreases in dogs (by about 50%) between birth and 1 to 2 months (Kühl et al., 2000; Wolford et al., 1988), after which irregular but moderate variations have been reported (Broulet et al., 1986; Cowgill and Spangler, 1981; Lowseth et al., 1990; Passing and Brunk, 1981; Strasser et al., 1993, 1997; Vajdovich et al., 1997). P-Urea increased from birth to 6 months in Great Danes, except those fed on low-protein diets (Nap et al., 1991). P-Urea was similar in adult horses and newborn foals, decreasing by about 50% in foals on the first day and remaining low for at least 2 months (Edwards et al., 1990). P-Urea decreased during the first week in calves (Hartmann et al., 1987), although others did not report any change during this time (Klee et al., 1985). • Individuals: Intraindividual variations in horses were more important than the variations observed before and after foaling (Doreau and Martin-Rosset, 1985). • Biological rhythms: P-Urea in sheep was 30% higher in summer than in winter (Nawaz and Shah, 1984), but other authors found no seasonal difference in dogs (Strasser et al., 2001). e. Pathological Factors of Variation The efficiency of P-Urea in renal failure diagnosis has not been reported. The critical difference in dogs was 2.4 mmol/l around a mean value of 5 mmol/l (Jensen and Aaes, 1993). A threshold of decision of 10 mmol/l has been suggested for cattle with suspected renal disease (Campbell and Watts, 1970). The relationship between P-Urea and P-Creatinine in dogs was reported to be low (Gabrisch, 1973) or high and linear (Toutain et al., 2000), except for frequent increases in P-Urea concentration, with normal P-Creatinine likely the result of poor preanalytical conditions (Medaille et al., 2004). Because of great interindividual variations, differences in the urea/creatinine ratio cannot be related to specific diseases (Finco and Duncan, 1976). The variations in P-Urea with disease are similar to those of P-Creatinine, but numerous extrarenal factors may contribute to increased P-Urea, such as gastrointestinal hemorrhage, fasting, or sepsis, which increase protein catabolism; thyrotoxicosis, which lowers P-Urea by increasing GFR; and decreased renal perfusion, which increases renal reabsorption (DiBartola et al., 1996; Prause and Grauer, 1998). Other factors of decreased P-Urea include portosystemic shunts, malnutrition (Davenport et al., 1994), liver insufficiency, and Krebs-Henseleit cycle enzyme defects. See the reviews in Dial (1995) and Sutherland (1989). These extrarenal factors of variation explain why P-Urea is less specific than P-Creatinine for the diagnosis and management of CRF and should not be recommended as a test of renal function. However, as P-Urea greatly depends on protein supply, it is a useful tool for monitoring the effects of dietary protein restriction (Devaux et al., 1996). P-Urea is less effective in cattle than P-Creatinine to evaluate decreased GFR in diarrheic calves (Brooks et al., 1997), and only 30% of cattle with P-Urea above the upper limit of the reference interval had renal disease (Campbell and Watts, 1970). f. Effects of Prolonged Increases of P-Urea: Carbamylated Hemoglobin The carbamylation of proteins is an irreversible nonenzymatic reaction occurring between amino groups of proteins and isocyanate, the active form resulting from cyanate isomerization. This latter is derived from the spontaneous dissociation of urea in solution into ammonium and cyanate ions. Thus, the higher and longer the concentration of P-Urea, the higher the concentration of carbamylated proteins, for instance, of hemoglobin (a process analogous to protein glycation in diabetes mellitus). As proposed in human medicine (Stim et al., 1995; Wynckel et al., 2000), carbamylated hemoglobin may be useful for assessing canine CRF and distinguishing it from ARF (Heiene et al., 2001b), whereas others found a significant overlap with specificity and sensitivity equal to 96% and 84%, respectively, at a 100 μg/g Hb threshold (Vaden et al., 1997a). Cystatin C is a small constitutive protein (MW ~14,000) synthesized by all nucleated cells and only cleared by glomerular filtration. The plasma concentration is thus increased in cases of renal failure. Cystatin C is considered the most sensitive marker of renal failure in humans. See the reviews in Filler et al. (2005), Laterza et al. (2002), and Price (2000). It can be measured with reagents used in humans in plasma from dogs (Almy et al., 2002; Jensen et al., 2001; Martin et al., 2002) but not cats (Martin et al., 2002). A 50% decrease of P-Cystatin C was reported in dogs following a meal, so sampling should be done after a 12-hour fast (Braun et al., 2002). The upper limit of the reference interval in dogs is 1.3 mg/l (Braun et al., 2002) and similar to the human threshold. P-Cystatin C is well correlated with P-Creatinine and GFR in normal dogs and in dogs with reduced GFR (Almy et al., 2002; Braun et al., 2002). At present, no diagnostic advantage of P-Cystatin C over P-Creatinine has been demonstrated in dogs. The determination of glomerular filtration rate (GFR)—that is, the volume of ultrafiltrate produced per unit of time (e.g., ml/min) by glomerular filtration—is considered the best way to evaluate kidney function. This is based on the hypothesis of the “intact nephron”: as “the surviving nephrons of the diseased kidney largely retain their essential functional integrity” and “retain a remarkably uniform relationship between glomerular and tubular function” (Bricker et al., 1997). GFR depends on the size of the animal, so different modes of expression have been proposed. One of the most frequently used is to relate GFR to body weight (ml/min/kg) or better still to lean body mass in humans (Swaminathan et al., 2000). Another approach is to use the body area (ml/min/m2), but as the equations used to calculate this from BW have not been validated in all species, this may introduce further inaccuracy (Price and Frazier, 1998). Another mode of expression consists of relating GFR to the volume of the extracellular compartment, as one kidney function is the regulation of body water content. This indexing is rarely adopted but has been used in humans (Peters et al., 2000) and dogs (Gleadhill, 1994; Gleadhill and Michell, 1996). There is no easy method for determining GFR from a single blood or urine specimen. In human clinical pathology, there are equations to estimate GFR from P-Creatinine, gender, weight, and age. The most frequently used are the Cockroft-Gault’s equations, but these are imprecise and the results depend on the techniques used for P-Creatinine (Grubb and Nordin, 2006; Wuyts et al., 2003). No such equations are available for use in animals. To our knowledge, the only study relating GFR to P-Creatinine showed that the estimation of canine GFR from P-Creatinine was imprecise, and the authors did not recommend using the equation to estimate GFR (Finco et al., 1995). The measurement of GFR is based on the clearance of markers freely filtered by the glomerulus and having no or minor secretion and reabsorption. See the review in Heiene and Moe (1998). The accepted reference for GFR determination is the urinary clearance of inulin, a low-molecular-weight polysaccharide (Shannon, 1935). The total amount of this marker eliminated in urine over a period of time is equal to the filtered load: Total amount eliminated during time t = (U-Inulin) × (U-Volume) = GFR × (P-Inulin) GFR = (U-Volume) × (U-Inulin)/(P-Inulin) × t in which U-Inulin and P-Inulin are in the same units, volume is in ml, and time in min, thus GFR is in ml/min. However, such determinations are labor intensive: they necessitate the maintenance of a constant plasma concentration by constant infusion of the marker, the accurate determination of urine volume, and precise and accurate measurement of the marker. Urine collection is often impractical as the total urine is required, which implies careful washing of metabolic cages (Watson et al., 2002a) or the use of indwelling catheters. See the review on urine collection and preservation in cats and dogs in Osborne (1995). Easier procedures have been proposed, based on the following: • The use of other markers: Some markers; such as ferrocyanide (Ladd et al., 1956), thiosulphate (Bing and Effersoe, 1948; Dalton, 1968), and sulfanilate (Maddison et al., 1984; Ross and Finco, 1981) have been abandoned. The endogenous or exogenous clearance of creatinine is the most frequently used technique, whatever the species. Iodinated radiocontrast media (e.g., iohexol) provide a good alternative to inulin, but most laboratories are not equipped to measure iodine (Westhoff et al., 1993, 1994). The metabolism of these various markers has not been documented in domestic animals. In some cases extrarenal metabolism can be significant, as shown in cats for diethylenetriaminepentaacetic acid (DTPA), which first concentrates in the heart and lung, then accumulates moderately in the liver before being concentrated in the kidneys (Drost et al., 2000; Uribe et al., 1992). Radiolabeled markers (see the review in Daniel et al. [1999]) allow easier and more accurate measurement of concentrations, but their use is restricted to specially equipped centers: 131I-Inulin, 51Cr-EDTA (Biewenga and van den Brom, 1981; van den Brom and Biewenga, 1981), and 99 mTc-DTPA (Gleadhill et al., 1999). They have also been used in large species such as horses (Matthews et al., 1992; Walsh and Royal, 1992). • The technique of administration: Single injection instead of continuous infusion and determination of plasma clearance of the marker, with calculations based on the decreased plasma concentration of the marker (Pihl and Nosslin, 1974; Summerville and Treves, 1986). • The use of nuclear imaging techniques and 99mTc-labeled tracers such as DTPA. These permit the functions of each kidney to be evaluated separately (Assailly et al., 1977; Drost et al., 2000; Krawiec et al., 1988), as these may be similar in healthy but not always in diseased dogs (Cowgill and Hornhof, 1986; Lourens et al., 1982). Determination of GFR by imaging is not as precise as clearance methods (Barthez et al., 1998; Kampa et al., 2002). a. Preanalytical Factors of Variation • Anesthesia: In most cases, anesthesia/sedation was reported to have little or no effect on GFR determination in the dog (Bostrom et al., 2006; Gagnon et al., 1982; Lourens et al., 1982; Newell et al., 1997), but a decrease was reported after acepromazine alone (Lourens et al., 1982) and others found that GFR was slightly higher in anesthetized than in nonanesthetized dogs (Balint and Forgacs, 1967), maybe as a consequence of the intravenous fluids. Anesthesia could lead to reduction of GFR in dogs with reduced renal mass (Stone et al., 1981). Thiopental anesthesia had no effect on GFR in sheep (Cirio et al., 1990). • Hydration status: GFR was higher in dogs that were hyperhydrated, so hydration status should be standardized (Kerr, 1958; Kunze et al., 2006; Tabaru et al., 1993). In cats, infusion of Ringer lactate at 1 to 2 ml/min produced on average a 40% increase of GFR (Rasmussen et al., 1985). In sheep, GFR was lower in summer than in winter because of hemoconcentration (Nawaz and Shah, 1984). • Diet: GFR was higher in dogs fed animal proteins rather than other proteins (Bartges et al., 1995a, 1995b, 1995d; Kerr, 1958). It was higher with high levels of proteins in the diet in normal dogs and cats and after renal mass reduction (Adams et al., 1994; Bartges et al., 1995c; Bovee, 1992; Bovee and Kronfeld, 1981; Robertson et al., 1986). In partially nephrectomized dogs, low- or high-sodium diets had little influence on GFR (Greco et al., 1994). In cats, GFR was moderately reduced by low-sodium diets (Buranakarl et al., 2004), unchanged in cats receiving potassium-depleted food, but lowered when the same food was acidified with ammonium chloride (Dow et al., 1990). In cows, GFR was unchanged by mineral supply (Hartmann et al., 2001). GFR was higher in sheep fed a normal or high rather than a low protein diet (Cirio and Boivin, 1990a, 1990b; Cirio et al., 1990; Eriksson and Valtonen, 1982; Rabinowitz et al., 1973; Valtonen et al., 1982). • Meals: GFR increases ranging from 10% to 45% were observed after a meal of proteins in normal dogs and in dogs with renal mass reduction (Bourgoignie et al., 1987; Brown, 1992; Ewald, 1967; Jolliffe and Smith, 1932; Moustgaard, 1947; O’Connor and Summerill, 1976; Reinhardt et al., 1975). This postprandial increase of GFR was not observed in dogs with experimental Fanconi’s syndrome (Woods and Young, 1991). It was not reduced by fasting for more than 1 day (Moustgaard, 1947). No difference in GFR was observed when ponies were fed twice a day or received the same amount of food every 2 h (Clarke et al., 1990). • Exercise: GFR was unchanged by training in horses and dogs (McKeever et al., 1985, 2002) or by submaximal exercise for 20 or 60 min despite hemoconcentration (Hinchcliff et al., 1990; McKeever et al., 1991). During effort under anaerobic conditions in horses, GFR decreased sharply then returned to prerun values within 15 min (Schott et al., 1991). • Drugs: In dogs and sheep, GFR was increased by glucocorticoids, probably by an increase of plasma flow rate as in rats (Baylis and Brenner, 1978; Gans, 1975). GFR was decreased by furosemide in dogs and cats (Bostrom et al., 2003; Hanna et al., 1988). Controversial effects of NSAIDs were reported in dogs: either no change of GFR with meloxicam, carprofen, and ketoprofen (Crandell et al., 2004; Ko et al., 2000; Narita et al., 2005) or a moderate decrease with carprofen or ketoprofen (Forsyth et al., 2000). GFR was moderately decreased after repeated excretory urograms in dogs (Feeney et al., 1980a). In cats with experimental CRF, the ACE inhibitor benazepril increased GFR up to 30% (Brown et al., 2001), whereas ACE inhibitors could decrease GFR in sodium-restricted dogs (Hall et al., 1979). • Biopsy: GFR was not affected after repeated renal biopsies in dogs (Drost et al., 2000; Groman et al., 2004). b. Techniques of GFR Measurement The procedures used to calculate plasma clearances may also influence the results as they depend on the mathematical model chosen (Heiene and Moe, 1999; Powers et al., 1977; Watson et al., 2002a) and on the proposed limited sampling strategies (Barthez et al., 2001; Blavier et al., 2001; Finco, 2005; Watson et al., 2002a). Others have suggested measuring the concentration of an exogenous marker at a given time after injection (e.g., P-Creatinine after I.V. load in dogs) (Labato and Ross, 1991; Watson et al., 2002a). The measurement of creatinine concentrations by Jaffé technique (discussed earlier) led to erroneously high values for creatinine clearance in early studies. Because of the larger proportion of interfering substances in plasma than in urine with the Jaffé technique, P-Creatinine was overestimated in comparison to U-Creatinine (e.g., in dog urine) (Shannon et al., 1932). This discrepancy between creatinine and inulin clearances does not exist when the measurements are based on the more accurate enzymatic techniques (Finco et al., 1993). The results of GFR determination may vary according to the technique so their transferability is limited (Driehuys et al., 1998; Finco, 2005; Gagnon et al., 1971; Izzat and Rosborough, 1989; Oester et al., 1968; Rogers et al., 1991), and caution is required when using decision limits from other laboratories or from the literature. Correcting factors have been proposed in some cases, for instance, for iohexol plasma clearance and exogenous creatinine urinary clearance in dogs (Finco et al., 2001; Gleadhill and Michell, 1996). However, such results remain controversial. The estimation of GFR based on iohexol clearance, for example, was considered reliable in dogs and cats (Brown et al., 1996), whereas others showed fdifferences of up to 0.95 ml/min/kg with creatinine clearance (Miyamoto, 2001b). The urine clearance of endogenous creatinine and the urine and plasma clearance of exogenous creatinine gave similar results (Finco et al., 1981, 1991; Lee et al., 1983; Watson et al., 2002a), but plasma DTPA clearance was 1.15 time higher than plasma iohexol clearance in dogs (Moe and Heiene, 1995). FIGURE 16-7 Examples of mean GFR values in the dog, cat, horse, cattle, sheep, and swine. Each point is the result of an original study. Dog: Asheim et al., 1961; Bostrom et al., 2003; Brown and Finco, 1992; Ellis et al., 1973a; Ewald, 1967; Fettman et al., 1985; Finco, 1971, 2005; Finco and Cooper, 2000; Finco et al., 1993; Forsyth et al., 2000; Gagnon et al., 1971, 1982; Greco et al., 1994; Haller et al., 1998; Hartenbower et al., 1974; Henegar et al., 2001; Houck, 1948; Kampa et al., 2003; Kaneko et al., 1978; Kunze et al., 2006; Labato and Ross, 1991; Lee et al., 1983; Lefebvre et al., 1998, 1999; Lora-Michiels et al., 2001; Newell et al., 1997; Pihl and Nosslin, 1974; Russel et al., 1987; Tabaru et al., 1993; Watson et al., 2002; Westhoff et al., 1993. Cat: Adams et al., 1993, 1994; Bolliger et al., 2005; Brown et al., 1996; Buranakarl et al., 2004; Deguchi and Akuzawa, 1997; Drost et al., 2000; Fettman et al., 1985; Finco et al., 1997; Haller et al., 2003; Miyamoto, 1998; Miyamoto, 2001a, b; Osbaldiston and Fuhrman, 1970; Reichle et al., 2002; Rogers et al., 1991; Ross and Finco, 1981; Russo et al., 1986; Uribe et al., 1992. Horse: Clarke et al., 1990; Finco and Groves, 1985; Gelsa, 1979; Hinchcliff et al., 1990; Knudsen, 1959; Kohn and Strasser, 1986; Lane and Merritt, 1983; Matthews et al., 1992; Morris et al., 1984; Rawlings and Bisgard, 1975; Schott et al., 1991; Traver et al., 1977; Walsh and Royal, 1992; Zatzman et al., 1982. Cattle: Boehnke, 1980; Deetz et al., 1982; Fleming et al., 1991; Mercer et al., 1978. Sheep: Bickhardt and Dungelhoef, 1994; Cirio and Boivin, 1990a, 1990b; English et al., 1977; Filippich et al., 1985; Gans, 1975; Garry et al., 1990b; Nawaz and Shah, 1984; Nesje et al., 1997; Rabinowitz et al., 1973. Goat: Brown et al., 1990a, 1990b; Valtonen et al., 1982. Swine: Chaiyabutr et al., 1987; Mercer et al., 1979; Wendt et al., 1990. c. Reference Values and Physiological Factors of Variation As discussed previously, GFR measurements differ considerably according to the technique used and to the species. The mean values found in studies devoted to renal function are presented in Fig. 16-7. • Species: GFR varies with kidney weight between species. The GFR/BW ratio was proportionally higher in the rat than in dogs or humans (Holliday and Egan, 1962), but it was lower in cats than in dogs. • Breed: There is no published report of breed differences, but in dogs, GFR (ml/min/kg) was lower in large than in small animals, but large interindividual variability was observed in 20 to 40 kg dogs (Haller et al., 1998). • Gender: There was no gender difference in young dogs (Asheim et al., 1961). • Age: Great changes in GFR occur during the perinatal period. See the review in Horster (1977). GFR is low in newborn dogs, then steadily increases to peak between 1 and 3 months at values of about 50% higher than in adults, then decreases until about 6 months (Arant et al., 1974; Horster and Valtin, 1971; Kleinman and Lubbe, 1972; Lane et al., 2000; Laroute et al., 2005; Pelayo et al., 1984). Similar results were obtained in cats (Hoskins, 1991). Creatinine clearance was higher than inulin clearance in rabbit and human newborns; this might be because of tubular reabsorption by backflow across the leaky immature tubule (Guignard and Drukker, 1999). GFR in lambs was multiplied by 4 between the end of pregnancy and second day of life (Berry et al., 1995). GFR increased during the first 3 weeks in calves (Hartmann et al., 1987) and decreased in piglets between 3 and 6 months of age (Krogh et al., 1979). • GFR values in mature dogs (Laroute et al., 2005) were similar to those reported in young adults. In cats, GFR decreased by 40% between 10 months and 7 years (Bolliger et al., 2005). The GFR did not differ between 3- year- and 8- to 11-year-old cows (Deetz et al., 1982). • Pregnancy/lactation: GFR was higher in late pregnancy and lactation in ewes (Bickhardt, 1994) and was identical in nonpregnant nonlactating cows and in cows pre- and postpartum (Sellers et al., 1958). • Individuals: Intraindividual variability cannot easily be distinguished from analytical variability. In cats, the estimated CVs for the repeatability of iohexol, inulin, and creatinine clearances were <10% (Miyamoto, 1998, 2001b), and GFR values measured 1 month apart differed by a maximum of 20% (Graves et al., 1994) or did not change over several months (Adams et al., 1993). Interindividual variability in dogs was higher than intraindividual variability (Kampa et al., 2003), which was relatively low (Ewald, 1967; Finco et al., 2001): for example, in five dogs examined over 4 days, CVs ranged from 8% to 28% (Bovee and Joyce, 1979). Intraindividual variability was high in horses as GFR could vary by a factor of 3 in 2 consecutive 6-h periods (Morris et al., 1984). Interindividual variations were very large in sheep (Nesje et al., 1997). When examined together, the clearances of both kidneys were within 30% in cats (Drost et al., 2000) and almost the same in both kidneys of dogs (Groman et al., 2004). d. Variations with Disease Decreased GFR is the gold standard of renal failure, whatever its cause or subsequent evolution (discussed later). A critical approach should always be applied in the clinical evaluation of renal function (Finco and Barsanti, 1989), especially as the results obtained differ according to the method used (discussed earlier). Alterations of GFR may be secondary to many extrarenal diseases. GFR is decreased in hypothyroidism (Adams et al., 1997) and hypoxia (Lobetti et al., 1996). It may be decreased or not in experimental or spontaneous diabetes mellitus (Kaneko et al., 1978, 1979). GFR evaluation is also useful for monitoring the toxicity of drugs eliminated by renal filtration (e.g., carboplatin) (Bailey et al., 2004; Shapiro et al., 1988) and as an indicator of the effects of renal dysfunction on the pharmacokinetics of drugs (e.g., oxytetracyclin in dogs) (Duffee et al., 1990). a. Urine Osmolality versus Urine-Specific Gravity One of the most important functions of the kidney in mammals is to reabsorb more than 99% of the filtered water, so that the final urine is more concentrated than the glomerular ultrafiltrate. Urine concentration is best evaluated by osmolality (Bovee, 1969)—that is, the concentration of particles in a solution, independently of their chemical characteristics (1 sodium ion has the same osmotic effect as 1 molecule of urea or as 1 molecule of albumin). The main determinant of specific gravity in dog urine is urea (Meyer et al., 1997). The laws of osmolality are only valid for dilute solutions in which the different particles are completely independent and this is not verified in urine or plasma. See the review in Sweeney and Beuchat (1993). The urine concentration is determined in routine tests from the specific gravity (U-SG)—that is, the ratio of the weight of 1 l of solution to 1 l of water, which depends on the relative proportions and the molecular weight of all the compounds in solution (for instance, 300 mOsm/kg solutions of NaCl, urea, and glucose would have SGs of approximately 1.009, 1.014, and 1.054, respectively; the latter would correspond to ~1 mOsm/kg solution of albumin). Urine osmolality and specific gravity were highly correlated in dogs (Bovee, 1969; Dossin et al., 2003; Harvey, 1973; Hendriks et al., 1978; Meyer et al., 1997), sheep (English and Hogan, 1979), and cats (Lees and Osborne, 1979). The correlation was weaker in calves (Thornton and English, 1976). Maximum concentrations could be as high as 1400 mOsm/l in rabbits, 2400 mOsm/l in dogs, and 3200 mOsm/l in cat and sheep (Anderson, 1982). b. Preanalytical Factors of Variation • Specimen: In cats, U-SG was not changed by freezing (Lees and Osborne, 1979). Spot urines could give very different U-SG results in the same animal depending on the time of sampling. • Diet: U-SG was lower in cats supplied with moist food than with dry food (Palmore et al., 1978). In cows, U-osmolality was little changed when the mineral supply was reduced by 50% or increased to 200%, but the urine volume was greatly increased during the high mineral supply period (Hartmann et al., 2001). • Physical exercise: In greyhounds, U-osmolality was decreased (~8%) by training because of increased diuresis resulting from plasma volume expansion (McKeever et al., 1985). U-SG was transiently decreased for 1 to 2 h after an effort under anaerobic conditions in horses (Schott et al., 1991). The 25% to 75% interval for U-SG in postrace thoroughbred horses was 1.021 to 1.033 (Cohen et al., 2002). • Environment: Water intake, urine volume, and osmolality differed significantly in sheep depending on whether the environment was cool or hot, dry or humid (Guerrini et al., 1980). • Drugs: U-SG was increased in dogs after the administration of radiographic contrast media (Feeney et al., 1980b). In dogs, urine volume was increased and osmolality decreased after medetomidine (Burton et al., 1998) and after glucocorticoids administration, which interferes with the action of vasopressin on the kidney (Joles et al., 1980; Sirek and Best, 1952; Waters et al., 1997). In horses, U-Osmolality was increased after oral sodium bicarbonate loading (Rivas et al., 1997) and was low after treatment with furosemide (median 1.018) (Cohen et al., 2002). • Anesthesia: U-SG was not changed by halothane anesthesia in dogs (Lobetti and Lambrechts, 2000) but was decreased by isoflurane anesthesia in horses because of increased diuresis, maybe from the fluid support (Watson et al., 2002b). In horses, U-Volume was increased, and U-SG and U-Osmolality were decreased by xylazine and detomidine (Gasthuys et al., 1986, 1987; Nunez et al., 2004; Steffey and Pascoe, 2002; Thurmon et al., 1984; Trim and Hanson, 1986). c. Analytical Factors of Variation Measuring osmolality requires expensive equipment, thus is not easy to do in most veterinary clinics. Freeze-point osmometers are usually unsuitable for the direct measurement of highly concentrated urines, especially in cats (Lees and Osborne, 1979). U-SG must be measured by refractometry. See the review in George (2001). The reagent strips available for U-SG determination in humans should not be used for animal urine. The measurement is based on changes in ionic strength and gave satisfactory results with 80% to 90% of human urines (Burkhardt et al., 1982), but it was less accurate than refractometry (Dorizzi et al., 1987). Results with these test strips did not correlate with osmolality or refractometry in dogs (Allchin et al., 1987; Dossin et al., 2003; Paquignon et al., 1993; van Vonderen et al., 1995). To the authors’ knowledge this test strip has not been validated in other domestic species. d. Reference Values and Physiological Factors of Variation The range of variations of U-SG or U-Osmolality in healthy animals is large in all species, so that reference intervals are devoid of any relevance for spot urines. • Breed: U-SG was higher in miniature schnauzers than in labradors and might be a factor in oxalate stone formation (Stevenson and Markwell, 2001). • Gender: Sex had no effect on canine U-SG (van Vonderen et al., 1997). • Age: U-SG was lower in aged dogs than in young adults (van Vonderen et al., 1997). U-SG was low at birth in puppies, then increased over the first 2 months to values higher than those of mature dogs (Faulks and Lane, 2003; Laroute et al., 2005). U-SG/osmolality in newborn foals was similar to or moderately lower than that of adults, then it decreased to a state of hyposthenuria during the first day of life and remained so for at least 2 months (Edwards et al., 1990). • Inter- and intraindividual variability: U-SG high in canine urine, CVs were in the range of 30% to 40% (van Vonderen et al., 1997). • Biological rhythms: U-SG in dogs was slightly lower in evening than in morning samples (van Vonderen et al., 1997). e. Pathological Factors of Variation As variations in healthy animals can be large, hypo- or isosthenuria must either be confirmed on repeated samples or observed in moderately dehydrated or azotemic animals to be interpreted as an indicator of kidney dysfunction. A concentrating ability below the commonly accepted limits of 1.030 and 1.035, in dogs and cats, respectively, is considered inadequate. See the review as this pertains to dogs in Watson (1998). When plasma osmolality increases, osmoreceptors in the hypothalamus stimulate the release of ADH in blood, thus increasing water reabsorption and equilibrating plasma osmolality. Diabetes insipidus is an uncommon condition characterized by polyuria and polydipsia without glucosuria. It can be congenital or acquired (Harb et al., 1996) and results from decreased secretion of ADH by the hypothalamus (central diabetes insipidus) or from insensitivity of kidney cells to the effects of ADH. See the reviews in Cohen and Post (2002) and Neiger and Hagemoser (1985). Tests of water deprivation are based on the fact that sudden or progressive withholding of water produces progressive dehydration. The resulting increase in extracellular fluid osmolality triggers the release of ADH, thus an increase of U-osmolality and U-SG in healthy subjects. The concentrating ability after exogenous ADH administration can be measured to test whether the absence of urine concentration results from a central defect of ADH secretion or from peripheral resistance to ADH. See the review in Finco (1995a). Protocols for test combination have been proposed (Mulnix et al., 1976), as well as for ADH measurement, but they have not gained wide acceptance (Biewenga et al., 1987). In dogs as in humans, urinary excretion of aquaporin-2 occurs in parallel to ADH action and can be used as a marker of collecting duct responsiveness (van Vonderen et al., 2004). Water deprivation tests may be hazardous, so should only be used to investigate polydipsia-polyuria once significant kidney damage has been ruled out (MacDougall, 1981). They are contraindicated in dehydrated, azotemic, or hypercalcemic dogs or cats (Barsanti et al., 2000). Water restriction in 10-month-old beagles produced maximum U-SG = 1.070 after up to 23 h of water deprivation (Balazs et al., 1971). In adult dogs, maximum U-osmolality was 2738 mOsm/kg with corresponding U-SG of 1.076 after 72 h and a weight loss of 16% (Hardy and Osborne, 1979). In horses, water deprivation for 72 h led to an average loss of weight of 8%, and an increase in U-SG (mean ~1.050), the hematocrit, and P-Proteins (Genetzky et al., 1987; Rumbaugh et al., 1982). a. Fractional Excretion Many electrolytes are intensely reabsorbed after filtration, mainly in the proximal tubule; their excretion is thus increased when tubule dysfunction occurs. Urine electrolyte concentrations also depend on the alimentary supply as the homeostatic mechanisms aimed to stabilize plasma concentration modulate tubule reabsorption. They can thus greatly differ as a function of the diet in all species and on the proximity of meals in monogastric animals. The most meaningful information would be obtained from daily urine excretion, which is often impossible to obtain because of the difficulties associated with urine collection. Expressing the urinary elimination of a solute (X) as the ratio of the filtered load that is found in urine has been proposed, whence the name fractional excretion (FE): FE × = amount in urine/amount filtered in which U-X and P-X are the urine and plasma concentration of X respectively. Such spot measurements are often well correlated with daily elimination. See the reviews in Coffman (1980) and King (1994). However, spot determinations in cats are highly variable compared with 72-h values, and should thus be interpreted with caution (Finco et al., 1997). FENa and FEK are poor indicators of the daily excretion of these ions in animals with renal failure (Adams et al., 1991). FEs and standard clearances are highly correlated in horses for sodium, potassium, and phosphates (Traver et al., 1977) (e.g., spot measurements of FEPi are within 0.1% of the calculated 24 h value) (Lane and Merritt, 1983). In sheep FENa, FEK, FECl, and FEPi are highly correlated with the respective daily urine excretion of these ions (Garry et al., 1990c). b. Preanalytical Factors of Variation of Ion Excretion • Diet: Daily elimination and FENa, FEK, FECa, and FEMg are higher in fed than in nonfed healthy dogs, whereas the excretion of phosphate is unchanged (Lulich et al., 1991). Diuresis and urine mineral composition differ according to food composition and intake in dogs (Zentek et al., 1994) and cats (Sauer et al., 1985a, 1985b) (e.g., cats fed a low K diet had lower FEK and higher FECl) (Dow et al. 1990); cats supplemented with magnesium showed a four-fold increase of FEMg (Norris et al., 1999a), and FECa was increased in phosphate-depleted dogs (Goldfarb et al., 1977). FEPi is lower in dogs fed low-phosphate diets (Polzin et al., 1991) and low-protein diets (Polzin and Osborne, 1988). In cattle (see a review in Lunn and McGuirk [1990]), the composition of the diet greatly influences electrolyte balance, especially for calcium and magnesium (Gray et al., 1988): FEMg was about three times higher in cows receiving oral magnesium hydroxide than in controls (Kasari et al., 1990) and is mainly used to test magnesium status (Sutherland et al., 1986). In cows receiving a high mineral diet, FECa, FEMg, and FEPi were increased (Hartmann et al., 2001). In sheep artificially loaded with NaCl by oral or intraruminal route, GFR, FENa, and FEK increased, whereas P-Sodium remained stable (Meintjes and Engelbrecht, 1993). In cattle, sodium bicarbonate loading increased FENa and FEHCO3, decreased FEMg and FECa, but had no effect on FEK, FECl, and FEPi (Roby et al., 1987). In mares, FECa and FEPi are dependent on the diet (e.g., FEPi ranged from almost 0% to 20% when mares fed on pasture alone or with a high-P mineral supplement) (Caple et al., 1982a, 1982b). FECa, FEMg, and FEPi were increased in cows receiving a high-mineral diet (Hartmann et al., 2001). • Meals: In cats, urine excretion of phosphates and magnesium is moderately higher in the postprandial period (Finco et al., 1986). Postprandial variations of FENa and FEPi were observed in cats fed a high-phosphate high-sodium diet (Finco et al., 1989). FEs were altered after large meals in ponies (Clarke et al., 1990) • Physical exercise: In horses, FEK, FENa, and FECl were decreased by training (McKeever et al., 2002). FENa was increased, whereas FECl was decreased, and FEK remained unchanged during submaximal exercise (McKeever et al., 1991) or transiently decreased immediately after effort under anaerobic conditions (Schott et al., 1991). • Drugs and fluid therapy: In dogs, FEK is increased in volume expansion by NaCl infusion (Massry et al., 1969). FEK and FECl but not FENa are increased after medetomidine administration in dogs (Burton et al., 1998). In horses, I.V. infusions of glucose or saline solutions increased FENa, FECl, and FEPi, and FEK was also increased after glucose infusion (Roussel et al., 1993). In ponies, administration of xylazine increased FEK, FENa, and FECl (Trim and Hanson, 1986). c. Analytical Factors of Variation Ion concentrations in urine are usually measured with the same techniques as in plasma with ad hoc dilutions when necessary, but techniques have not been validated in animal species. An interfering substance in the urine of sheep, cattle, horses, and cats, but not dogs, causes falsely low results for potassium but not sodium with ion-selective electrodes (Brooks et al., 1988). d. Reference Intervals and Physiological Factors of Variation Mean values of FEs collected from the literature are summarized in Fig. 16-8. It should be remembered, as emphasized in horses (Morris et al., 1984), that interindividual variations are large and the values observed in individuals may be outside the limits of each group. • Age: In puppies, FENa increased during the first 6 months of life, whereas FEK peaked at about 4 months, and FECl, FECa, and FEPi were little changed (Lane et al., 2000). • Pregnancy-lactation: In cows, significant changes in FENa, FEK, FECl, FECa, and FEPi were observed prepartum and during lactation (Ulutas et al., 2003). FENa, FEK, and FECl were unchanged with the stage of lactation, whereas FEPi, FECa, and FEMg differed (Fleming et al., 1992). • Biological rhythms: In fasted dogs, FENa and FECa were higher in the morning and FEPi in early afternoon (Hartenbower et al., 1974). In cows, FENa, FEK, FECl, FECa, FEPi, and FEMg did not vary significantly over 24 h but most showed great interindividual variability (Fleming et al., 1991). Other authors have observed significant changes in Pi, Na, and K total excretion and FEs with maximal values at the middle of the day, which did not depend on age or production category (high or low) (Fleming et al., 1992). FIGURE 16-8 Mean values of FE of sodium, potassium, chloride, and inorganic phosphates. Each point is the result of an original study in dogs (D), cats (C), horse (H), bovine (B), and sheep (S). The y-axis has a logarithmic scale. FENa *Dog: Burton et al., 1998; Clarke et al., 1990; Deguchi and Akuzawa, 1997; Finco et al., 1997; Fleming et al., 1991; Gagnon et al., 1982. *Cat: Gans, 1975; Garry et al., 1990a, 1990b; Gelsa, 1979. *Horse: Izzat and Rosborough, 1989; Kohn and Strasser, 1986; Lobetti and Joubert, 2000; Lobetti and Lambrechts, 2000; Lulich et al., 1991; McKeever et al., 1991; Morris et al., 1984; Neiger and Hagemoser, 1985. *Sheep: Roussel et al., 1993. *Cattle: Adams et al., 1991; Bickhardt and Dungelhoef, 1994; Buranakarl et al., 2004. FEK *Cattle: Adams et al., 1992; Beech et al., 1993; Bickhardt and Dungelhoef, 1994. *Dog: Buranakarl et al., 2004; Burton et al., 1998; Clarke et al., 1990. *Cat: Clarke et al., 1990; Deguchi and Akuzawa, 1997; Dow et al., 1990; Edwards, 1989; Finco et al., 1997; Fleming et al., 1991. *Horse: Gans, 1975; Garry et al., 1990a, 1990b; Gelsa, 1979; Lulich et al., 1991; McKeever et al., 1991; Morris et al., 1984; Neiger and Hagemoser, 1985. *Sheep: Roussel et al., 1993; Russo et al., 1986; Toribio et al., 2005; Traver et al., 1977; Ulutas et al., 2003. FECl *Cattle: Buranakarl et al., 2004; Burton et al., 1998; Dow et al., 1990. *Dog: Edwards, 1989. *Cat: Fleming et al., 1991; Garry et al., 1990a, 1990b. *Horse: Gelsa, 1979; Kohn and Strasser, 1986; McKeever et al., 1991; Morris et al., 1984; Neiger and Hagemoser, 1985; Roussel et al., 1993; Russo et al., 1986. *Sheep: Toribio et al., 2005; Ulutas et al., 2003. FEPi. *Cattle: Bickhardt and Dungelhoef, 1994; Caple et al., 1982. *Dog: Caple et al., 1982; Edwards, 1989. *Cat: Finco et al., 1997. *Horse: Fleming et al., 1991; Garry et al., 1990a, 1990b; Gelsa, 1979; Kohn and Strasser, 1986; Lane and Merritt, 1983; Lulich et al., 1991; Neiger and Hagemoser, 1985; Roussel et al., 1993; Russo et al., 1986. *Sheep: Toribio et al., 2005; Traver et al., 1977; Ulutas et al., 2003. e. Pathological Factors of Variation The main difficulty when interpreting FEs in the diagnosis of renal disease is that they are greatly influenced by all extrarenal factors involved in the regulation of plasma electrolyte balance, mainly by dietary supply (Finco and Barsanti, 1989; Finco et al., 1992a). As a result, the interpretation of increased FEs in terms of tubular dysfunction is often hypothetical. Only repeated measurements under well-controlled conditions (e.g., experimental settings) may offer some relevance. In cats with severe CRF, the FEs of ions were normal in most animals, and their measurement did not seem to improve diagnosis (Filippich, 1992). In dogs, it was shown that FEPi was a less accurate indicator of CRF than P-Creatinine (Gleadhill, 1994). FEs are also changed in nonrenal diseases. In diabetic cats, FEMg was about 20 times higher than in controls and could be responsible for the frequent hypomagnesemia observed in diabetes mellitus (Norris et al., 1999b). In the cat, a case of increased FEPi with normal P-Phosphates is described, probably because of deficient reabsorption and resulting in rickets-like symptoms (Henik et al., 1999). In horses, FEK is moderately but insignificantly lower during rhabdomyolysis (Beech et al., 1993) Proteinuria is one of the most frequent abnormalities in routine urinalysis and was observed in about 43% and 50% of canine and feline samples submitted to a university hospital (Barlough et al., 1981). See the review on human proteinuria in Waller et al. (1989). Although glomerular damage is the cause of the most intense proteinurias, it is not the only one: these can also originate from the tubules and their cause may be pre- or postrenal (Table 16-2). See the consensus statement on canine proteinuria in Lees et al. (2005). The following systematic approach is required in cases of confirmed proteinuria: (1) check that it is persistent, (2) evaluate the magnitude, and (3) localize the origin. See the reviews in Hurley and Vaden (1995), Kunze et al. (2006), and Lees et al. (2005). a. Origin of Urinary Proteins The characteristics of the glomerular filtration slit (see Section I.B) are such that almost no or very little plasma protein is filtered. The MW of albumin is closest to the filtration threshold, so this is the first plasma protein to escape into urine in the case of glomerular disturbance. See the review in Mathieson (2004). Filtered proteins are almost totally reabsorbed in the tubule, and the remaining molecules are substantially degraded before excretion in human or rat urine; this may lead to underestimation of U-Proteins with certain techniques but has not been documented in domestic animals (Greive et al., 2001). TABLE 16-2 Categories of Causes of Proteinuria Based on the Site or Mechanism of the Underlying Abnormality Prerenal (Definition: due to abnormal plasma content of proteins that traverse glomerular capillary walls having normal permselectivity properties.) Normal proteins that are not normally present free in the plasma (e.g., hemoglobin or myoglobin). Abnormal proteins (e.g., immunoglobulin light chains) (Bence-Jones proteins). Renal (Definition: due to abnormal renal handling of normal plasma proteins.) Functional (Definition: proteinuria that is due to altered renal physiology during or in response to certain transient phenomena [e.g., strenuous exercise, fever, and so on].) The key distinction here is that the proteinuria is not attributable to presence of renal lesions. The hallmarks of this type of proteinuria are that it is mild and transient—that is, it promptly resolves when the condition that is generating it resolves. Pathological (Definition: proteinuria that is attributable to structural or functional lesions within the kidneys, regardless of their magnitude or duration.) Glomerular (Definition: due to lesions altering the permselectivity properties of the glomerular capillary wall.) Tubular (Definition: due to lesions that impair the tubular recovery of plasma proteins that ordinarily traverse glomerular capillary walls having normal permselectivity properties.) These plasma proteins traffic into the urine from glomerular capillaries. They consist mainly of low-molecular-weight proteins but may also include small amounts of moderate molecular weight proteins (e.g., albumin). Interstitial (Definition: due to inflammatory lesions or disease processes [i.e., acute interstitial nephritis] causing exudation of proteins into the urinary space.) These proteins traffic into the urine from peritubular capillaries. Postrenal (Definition: due to entry of protein into the urine after it enters the renal pelvis.) Urinary (Definition: due to entry of proteins derived from hemorrhagic or exudative processes affecting the walls of the urine excretory pathway, renal pelvis, ureter, urinary bladder, and urethra [including into the urethra from the prostate gland in males].) Extraurinary (Definition: due to entry of proteins derived from secretions or from hemorrhagic and/or exudative processes affecting the genital tract and/or external genitalia during voiding or in the process of collecting urine for analysis.) Reproduced from Lees et al. (2005) with permission of JVIM.
I. INTRODUCTION
II. KIDNEY MORPHOLOGY AND FUNCTION
A. General Structure of the Kidneys
B. Glomerulus and Filtration
C. Tubule: Reabsorption and Secretion
1. Parts of the tubule
2. Functions of the tubule
. They are reabsorbed in the proximal tubule by a sodium cotransporter, which is inhibited by PTH, thus increasing phosphodiuresis. Some newly identified peptides may also decrease tubular phosphate reabsorption without any alteration of glucose and amino acid reabsorption and thus lead to renal loss of phosphates. See the reviews in Laroche and Boyer (2005)and Ritz et al. (2003). The Tm for phosphate reabsorption is higher in ruminants than in other species (Summerill and Lee, 1985; Symonds and Manston, 1974).
) buffer system is the most efficient extracellular buffer system. It is based on the low dissociation of carbonic acid (pKa ~6.1) at the pH of the extracellular/intravascular compartment and the fact that it is an open system eliminating CO2 in the lungs. Its efficiency is enhanced by the action of carbonic anhydrase, which accelerates the hydration of carbonic anhydride (CO2) into carbonic acid. High concentrations of carbonic anhydrase occur in many tissues including the kidney tubule. The relationship between CO2, H2CO3, and HCO3 is expressed in the following equation:
III. TESTS OF KIDNEY FUNCTION
A. Indirect Tests of Glomerular Function
1. Creatinine
2. Urea
3. Cystatin C
B. Direct Tests of Glomerular Function
1. Determination of GFR
2. Variations of Results of GFR Determination According to Procedure
C. Tests of Tubule Function
1. Concentrating ability
2. Tests of water deprivation
3. Urine excretion of Ions
= (U-X × U-Volume)/(P-X × GFR)
IV. TESTS OF KIDNEY DAMAGE
A. Glomerular Damage
1. Proteinuria
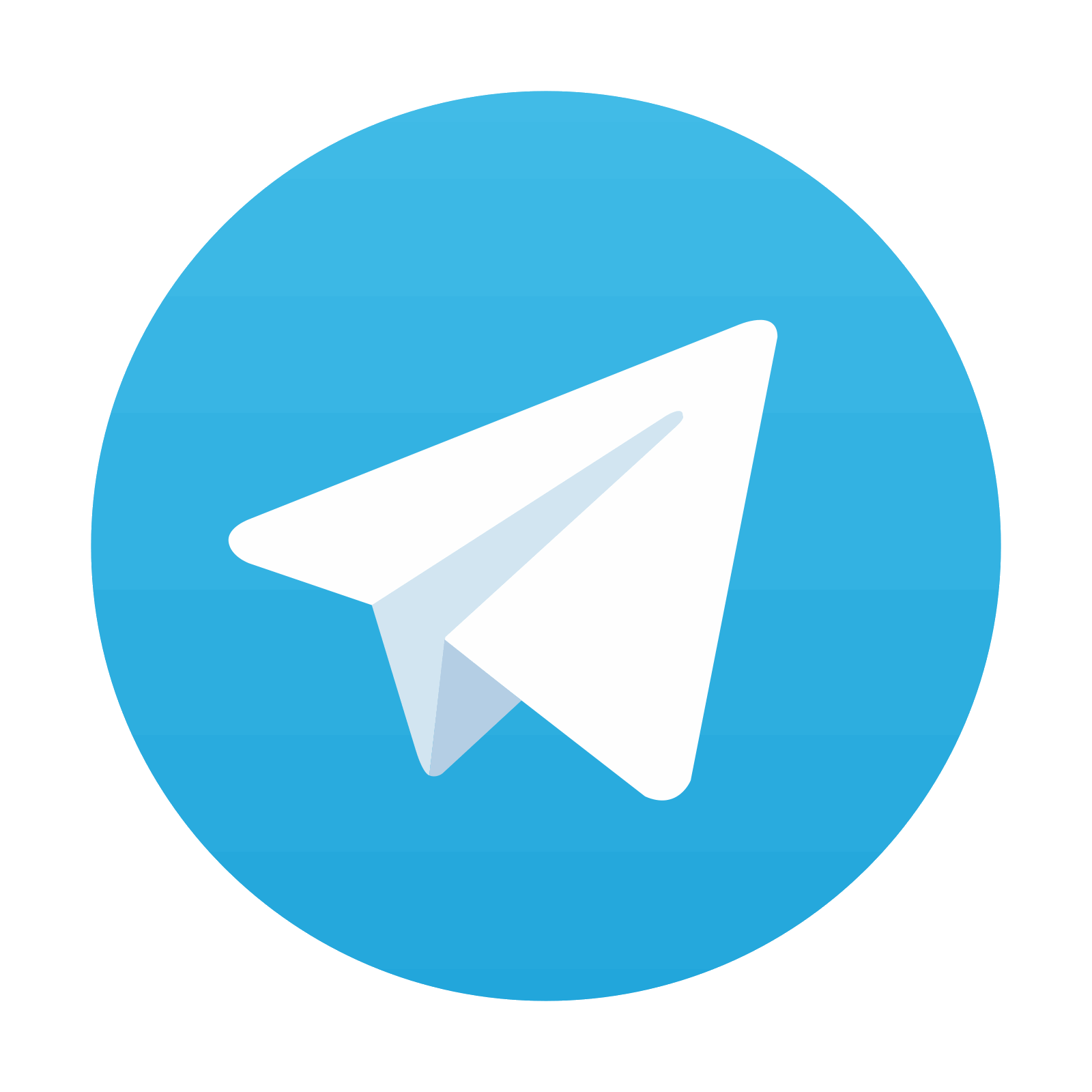
Stay updated, free articles. Join our Telegram channel
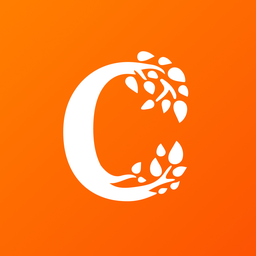
Full access? Get Clinical Tree
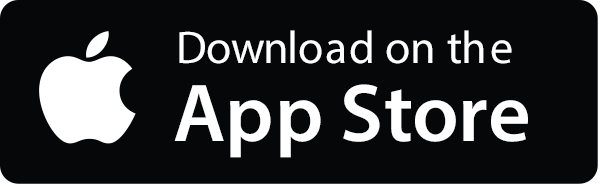
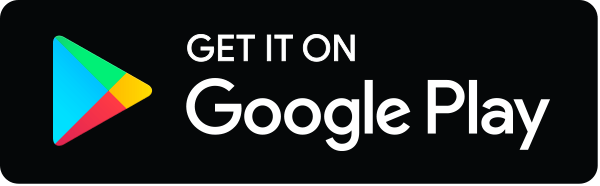