Chapter 1 Integration of Gastrointestinal Function
Integration of Function
The digestive system includes the primary gastrointestinal (GI) tract (oropharynx, esophagus, stomach, intestine, and colon), exocrine pancreas, liver, and biliary tract. The role of the digestive system is to provide for nutrition, energy balance, intermediary metabolism, and a mechanism for excretion. To fulfill this role, the digestive system has evolved six major functions: (a) Motility—Motility permits the prehension of food and its transit from mouth to anus, while at the same time mixing and reducing the size of the food content. The rate at which food moves through the GI tract is regulated to optimize time for secretion, digestion, and absorption. (b) Secretion—Secretions from the salivary glands, stomach, intestine, pancreas, liver, and biliary tract add fluid, electrolytes, acid, bicarbonate, mucus, bile salts, and enzymes to the GI tract lumen. These serve to aid in the digestion and absorption of ingested nutrients. (c) Digestion—Ingested nutrients are progressively reduced in size primarily by luminal hydrolytic digestion with some fermentative digestion contributed by colonic bacteria. (d) Absorption—The intestine has a highly specialized epithelium, which mediates the absorption of nutrients, electrolytes, minerals, vitamins, and water. (e) Blood flow—The major function of the splanchnic circulation is to support the broad range of metabolic activities described above. The splanchnic circulation also serves as a storage site for a large volume of blood that can be mobilized during exercise. (f) Metabolism—The liver has a wide range of metabolic functions, including carbohydrate, protein, lipid, and nucleic acid metabolism; coagulation factor synthesis; bile secretion; porphyrin, metal, vitamin, glutathione, hormonal, and xenobiotic metabolism; and immune surveillance. The elements of this highly integrated model are outlined in Figure 1-1. Neural, endocrine, and paracrine control mechanisms serve to regulate and integrate these various functions.
Structural Organization
Primary Gastrointestinal Tract
The structural organization of the primary GI tract consists of a luminally oriented mucosa, submucosa, muscularis externa, and serosa (Fig. 1-2).1 The mucosa consists of a superficial epithelium, lamina propria, and muscularis mucosae. Epithelial cells carry out digestive, secretory, and absorptive functions, as well as immune surveillance. The lamina propria consists of connective tissue, blood, and lymphatic vasculature. The muscularis mucosae is made up of smooth muscle cells that function primarily to change the shape and surface area of the epithelial cell layer in response to luminal distention. The submucosa consists of collagen, elastin, secretory glands, and major blood vessels. Motility of the GI tract is provided by circular and longitudinal layers of smooth muscle, interposed between the submucosa and serosa. Two nerve plexuses, the submucosal plexus and myenteric plexus, contain the intrinsic nervous system of the GI tract. The submucosal plexus (Meissner’s plexus) lies between the submucosa and the circular smooth muscle, and regulates the overlying epithelium. The myenteric plexus lies between the circular and longitudinal muscle layers, and regulates their contraction. The serosa is a thin external membrane consisting of a layer of serous fluid-secreting cells. Serous secretions serve to reduce friction from the muscular motion of the GI tract. Each segment of the primary GI tract has unique features that promote one or more of the GI functions (Fig. 1-3A to 1-3D).
Pancreas
The pancreas contains exocrine and endocrine elements, which serve to regulate digestion and metabolism, respectively. The exocrine pancreas is a mixed tubuloalveolar or tubuloacinar gland. Acinar cells synthesize and secrete digestive enzyme into a ductal system for transport to the small intestine (Fig. 1-3E).2,3 Islet cells of the endocrine pancreas are interspersed between the acinar and duct cells. The exocrine pancreas has four major physiologic functions: (a) acinar cell secretion of digestive zymogens, which initiate protein, carbohydrate, and lipid digestion; (b) acinar cell secretion of antibacterial proteins, which serve to regulate the small intestinal bacterial flora; (c) ductal cell secretion of bicarbonate and water, which serves to hydrate and neutralize the duodenal pH; and (d) ductal cell secretion of pancreatic intrinsic factor, which facilitates cobalamin (vitamin B12) binding and absorption in the distal ileum.2,3 The exocrine pancreas does not have a direct arterial blood supply. Acinar cells are instead perfused by venous blood emanating from islet vasa efferentia via an islet–acinar portal system.4 Arterial blood first perfuses the islets, which secrete their hormones (e.g., insulin, glucagon) into the postcapillary venules and veins that then perfuse the acinar cells. The endocrine pancreas thereby autoregulates exocrine secretions and pancreatic growth. The efferent nerve supply to the pancreas includes both sympathetic and parasympathetic fibers. Sympathetic postganglionic fibers arise from the celiac and cranial mesenteric plexuses and accompany the arteries to the exocrine pancreas. Parasympathetic preganglionic fibers are distributed by branches of the vagi traversing the stomach and duodenum and terminate at the acini, islets, or intrinsic cholinergic nerves of the pancreas. In general, parasympathetic nerve fibers stimulate exocrine pancreatic secretion, and sympathetic nerve fibers inhibit exocrine pancreatic secretion.5
Liver
The hepatic lobule is the anatomic unit of the liver. In the anatomic model, liver lobules are organized into irregular polygons demarcated by connective tissue and composed of plates of hepatocytes radiating outward from the central vein to the portal triads (Fig. 1-3F). The hepatic acinus is the functional unit of the liver. In the functional model, hepatocytes are instead oriented around the afferent vascular system (portal veins and hepatic arteries) just as they anastomose into sinusoids (Fig. 1-3F), and the central vein is at the periphery of the acinus instead of centrally located as in the anatomic model. The acinus is divided into three contiguous zones (1-3) that correspond to distance from the arterial blood supply. Hepatocytes closest to the arterioles (zone 1) receive the greatest oxygen content, but are also the first to be affected by toxins transported from the gut to the portal vein. Zone 3 hepatocytes reside at the periphery of the acinus near the central vein, and zone 2 hepatocytes are interspersed between zones 1 and 3. The anatomic model is perhaps easier to understand, but the functional model serves as a better foundation for understanding liver pathology.6,7
In either model, portal venous and arterial blood flows centripetally, that is, toward the central vein, whereas bile flows centrifugally, that is, away from the central vein. Hepatocytes extract nutrients and oxygen from portal and arterial perfusion, respectively, and produce bile acids and other bile constituents that are transported from hepatocytes into bile canaliculi, ductules, and ducts.8 Hepatocytes account for 60% of the liver cell mass and contribute to the wide range of metabolic activities described in the preceding section. Hepatocytes are supported by other cell types, which account for 40% of the liver cell mass.
Biliary Epithelial Cells (Cholangiocytes)
These represent 7% to 10% of liver cell mass.9,10 They secrete water, bicarbonate, and cations into the bile in the physiologic state, but in some diseases they may participate in the immune response as antigen-presenting cells (APCs).
Kupffer Cells
These represent 2% to 5% of the liver cell mass, and serve as resident macrophages lining the sinusoids. Kupffer cells are phagocytic and may contribute to pathology through production of inflammatory mediators, activation of Toll-like receptors (TLRs), and elaboration of proinflammatory cytokines such as tumor necrosis factor (TNF)-α.7
Hepatic Stellate Cells
Formerly known as Ito cells, stellate cells represent 3% to 5% of liver cell mass and store lipid and vitamin A in the healthy liver. When activated in liver damage, stellate cells produce collagen, causing hepatic fibrosis that can progress to end-stage cirrhosis.11
Hepatic Endothelial Cells
Hepatic sinusoidal endothelial cells represent 20% of the liver cell mass and act as resident APCs in health, although they contribute to a heightened immune response in disease conditions.12
Innervation of the Gastrointestinal Tract
The autonomic nervous system (ANS) innervation of the GI tract has both extrinsic and intrinsic components (Fig. 1-4). The extrinsic component consists of the parasympathetic and sympathetic branches. The intrinsic component is the enteric nervous system that is contained within the submucosal and myenteric plexuses (Fig. 1-5).14 The enteric nervous system communicates extensively with the parasympathetic and sympathetic nervous systems.
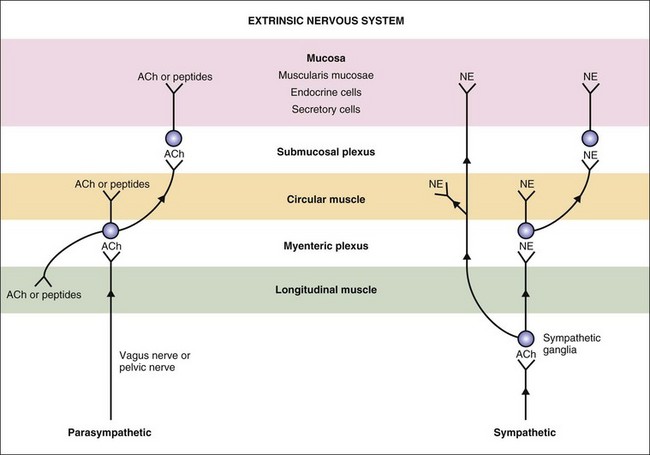
Figure 1-4 Extrinsic nervous system of the gastrointestinal tract. ACh, acetylcholine; NE, norepinephrine.
(From Costanzo L [ed]: Physiology, 4th ed. Philadelphia: Saunders, 2010.)
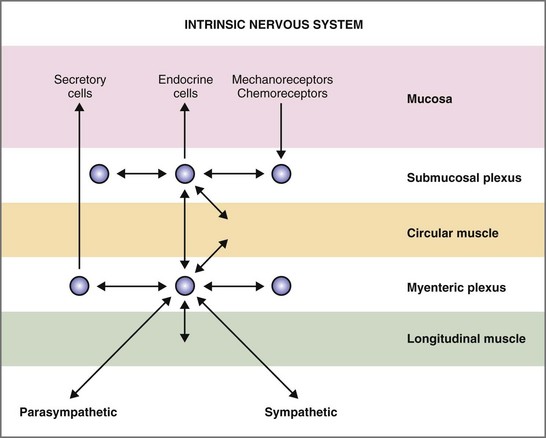
Figure 1-5 Intrinsic nervous system of the gastrointestinal tract.
(From Costanzo L (ed): Physiology, 4th ed. Philadelphia: Saunders, 2010.)
Intrinsic Innervation
The ganglia of the enteric nervous system are located in the submucosal and myenteric plexuses, and control the contractile, secretory, and endocrine functions of the gut.15,16 Enteric ganglia receive inputs from the parasympathetic and sympathetic nervous systems, which serve to modulate their activity. Enteric neurons are cholinergic, adrenergic, and/or peptidergic, and may secrete one or more neurotransmitters (Fig. 1-5).
Gastrointestinal Smooth Muscle
Significant structural and functional differences exist between skeletal and smooth muscle, from innervation and gap junctions to sarcomeric organization, nuclear density, spread of depolarization, regulatory proteins, sources of calcium, contractile patterns, slow waves, and bioenergetics (Tables 1-1 and 1-2).17
Table 1-1 Structural Differences Between Striated and Smooth Muscles
Feature | Skeletal Muscle | Smooth Muscle |
---|---|---|
Innervation | Somatic | Autonomic |
Nerve density | Each cell | Sparse (unitary) |
Appearance | Striations | Nonstriated (smooth) |
Nuclei | Multinucleated | Single nuclei |
Depolarization | T tubules | Caveolae |
Gap junctions | None | Many (unitary) |
Thin filaments | Troponin | Calmodulin |
Ca2+ source | Cai2+ | Cai2+, Cao2+ |
Table 1-2 Bioenergetic Differences Between Striated and Smooth Muscles
Parameter | Skeletal Muscle (Frog Sartorius) | Smooth Muscle (Hog Carotid Artery) |
---|---|---|
Stress | 250 mN/mm2 | 223 mN/mm2 |
T1/2 Pmax | 0.2 sec | 70.0 sec |
Velocity | 1.9 Lo/sec | 0.12 Lo/sec |
JATP | 50 µmol/g/min | 1.2 µmol/g/min |
Economy | 930 µmol ATP/min/g/mN/mm2 | 6.0 µmol ATP/min/g/mN/mm2 |
ATP, adenosine triphosphate; JATP, joules of ATP consumed. Lo/sec, optimal muscle lengths per second; T1/2 Pmax, time to reach half-maximal contraction.
Regulatory Proteins
Contraction of striated muscle is a thin-filament regulated process. Ca2+ binds to troponin C, one of the thin filament proteins, producing a conformational change in the troponin–tropomyosin complex. The conformational change permits binding of actin to the myosin heads, activation of myosin adenosine triphosphatase (ATPase), and cross-bridge cycling. Contraction of smooth muscle is instead a thick-filament regulated process. Troponin C is not expressed in smooth muscle, and Ca2+ instead binds to the calcium-binding protein, calmodulin. In turn Ca2+–calmodulin activates myosin light-chain kinase, permitting phosphorylation of the 20-kDa myosin light chains, activation of myosin ATPase, and cross-bridge cycling (Fig. 1-6).18
Sources of Calcium
Striated muscle cells derive calcium solely from internal stores, specifically the sarcoplasmic reticulum. Depolarization of the T-tubule system causes a conformational change in its voltage-sensitive dihydropyridine receptor, which, in turn, causes opening of the Ca2+-release channel in the adjacent sarcoplasmic reticulum. Many GI smooth muscles have a similar dependence on sarcoplasmic reticulum Ca2+ release, but most smooth muscles also have a major (or minor) dependence on influx of extracellular Ca2+ through voltage-gated, ligand-gated, and inositol triphosphate (IP3)-gated Ca2+ channels (Fig. 1-7).
Slow Waves
Action potentials in smooth muscle are triggered by oscillating depolarization and repolarization waves referred to as “slow waves.”19 Slow waves are a unique feature of the electrical activity of GI smooth muscle. The origin of the slow waves is still unsettled,20 but it has been proposed that slow waves originate in the interstitial cells of Cajal (ICC) in the myenteric plexus.19 Cyclic depolarizations and repolarizations of the ICC are postulated to rapidly spread to adjacent smooth muscle via low-resistance gap junctions. In this way the ICC could serve as “pacemakers” and set the slow wave frequency (from 3 to 12 slow waves per minute) along the GI tract. When slow waves depolarize the membrane potential to threshold, action potentials are superimposed on top of the slow waves giving rise to strong contractions.19
Bioenergetics
Smooth muscles contract much slower (Pmax t1/2 = 70 sec; shortening velocity = 0.12 L/sec) than striated muscle, but they nonetheless achieve the same overall force production (S
= 223 mN/mm2; see Table 1-2). Smooth muscles can develop and maintain this force at surprisingly low adenosine triphosphate (ATP) consumption (JATP = 1.2 µmol/g/min). Consequently smooth muscle contraction is more economical from a bioenergetics standpoint. It is this property (high-force output at low ATP consumption) that permits some smooth muscles, notably the GI tract sphincters, to maintain tone for prolonged periods of time.
Motility
Oropharynx and Esophagus
In the oropharyngeal phase of swallowing, the animal prehends food and water with teeth and tongue, masticates and mechanically reduces the size of the food particles, forms a bolus at the base of the tongue, and propels the bolus caudally to the cricoesophageal sphincter. The sphincter relaxes and the bolus passes into the cranial esophageal body. Oropharyngeal mechanoreceptors transmit mechanical forces through an afferent neural pathway to the brainstem and efferent neural pathway to induce cricopharyngeal relaxation, esophageal body contraction, and primary esophageal peristalsis. Following swallowing, the cricopharyngeus contracts, pharyngeal muscles relax, and the oropharyngeal phase is repeated until feeding is completed (Fig. 1-8).
Aside from fluid and electrolyte secretion, the major physiologic function of the esophagus is the transport of ingested liquids and solids from the oral cavity to the stomach. Anatomic structures that permit this function are the striated muscle of the cranial esophageal sphincter (cricopharyngeus), the striated and smooth muscle of the esophageal body, and the smooth muscle of the caudal esophageal (gastroesophageal) sphincter. An important species difference between the dog and cat is in the musculature of the esophageal body. The full length of the canine esophageal body is composed of striated muscle, whereas the distal one-third to one-half of the feline esophageal body is composed of smooth muscle. The striated muscle of the cranial esophageal sphincter and esophageal body are innervated by somatic branches (glossopharyngeal, pharyngeal, and recurrent laryngeal) of the vagus nerve arising from the brainstem nucleus ambiguus. The smooth muscle of the esophageal body and caudal esophageal sphincter are innervated by autonomic branches (esophageal) of the vagus nerve arising from the dorsal motor nucleus of the vagus.21,22
Primary peristaltic contractions associated with swallowing are reinforced by a secondary wave of contraction (secondary peristalsis) mediated physiologically by esophageal intraluminal distention. The gastroesophageal sphincter relaxes in advance of the propagated pressure wave to permit food to empty into the stomach. Once the bolus of food has passed into the stomach, the gastroesophageal sphincter resumes its high resting pressure.23,24
Stomach
Anatomically, the stomach is composed of five distinct components: cardia, fundus, corpus, antrum, and pylorus. Physiologically, the stomach behaves as a two-component structure: a proximal stomach (cardia, fundus, first one-third of the corpus) characterized by slow tonic contractions, and a distal stomach (distal two-thirds of the corpus and antrum) characterized by phasic propagating contractions (Fig. 1-9).25 Slow waves without action potentials give rise to the sustained tonic contractions of the proximal stomach. During swallowing, gastroesophageal sphincter and intragastric pressure decrease to accommodate emptying of solids and liquids. This phenomenon, referred to as receptive relaxation, takes place with each swallow, and as a consequence, large volumes can be accommodated with minimal increases in intragastric pressure. The proximal stomach becomes much less compliant with fundic disease or fundectomy.
A pacemaker site in the proximal fundus of the greater curvature generates action potentials and phasic contractions that propagate from the site of origin circumferentially and distally to the pylorus.26 During feeding, phasic contractions of the distal stomach trigger a repetitive cycle of propulsion, trituration, and retropulsion that progressively reduces the size of the ingesta. Thus, the peristaltic qualities of the distal stomach regulate the emptying of solid particles into the duodenum. Antral disease or antrectomy abolishes this physiologic effect resulting in a “dumping syndrome” as a result of accelerated gastric emptying, nutrient overload in the small intestine, and osmotic diarrhea.
Gastric emptying is regulated by several physiologic parameters, including (a) pyloric resistance and pressure differential between the stomach and duodenum; (b) water content—liquids are emptied more rapidly than solids; (c) nutrient composition—carbohydrates are emptied more rapidly than proteins which, in turn, are emptied more rapidly than lipids; (d) nutrient acidity—delayed at acid or alkaline pH; (e) nutrient osmolality—delayed at high osmolality; and (f) hot or cold temperatures. Duodenal, jejunal, and ileal braking mechanisms also feedback inhibit gastric emptying through activation of mucosal sensory receptors for fatty acids, tryptophan, osmolality, and acid. Intestinal braking mechanisms serve to prolong transit time and nutrient contact time.25
During the fasting state the stomach is ordinarily empty, aside from swallowed saliva, a small amount of mucus, and cellular debris that collects in the gastric lumen. In addition, there may be particles of indigestible solids left from the previous meal. A mechanism exists to empty this fasting content: the migrating motility complex (MMC). The ability of the MMC to completely empty the stomach of residue is so striking that it is sometimes referred to as the “interdigestive housekeeper” of the GI tract.25 The GI hormone, motilin, is involved in the regulation of the MMC. Cats and rabbits do not have an MMC, but instead have a less vigorous emptying pattern known as the migrating spike complex (MSC).27,28
Intestine
Contractions in the small intestine serve three general functions: mixing of the ingesta with digestive enzymes and other secretions; circulation of the intestinal contents to facilitate contact with the intestinal mucosa; and net caudal propulsion of the intestinal contents. Intestinal contractions are governed by four motility patterns: segmentation, peristalsis, intestinointestinal inhibition, and the MMC.29
Segmentation
If a contraction is not coordinated with activity above and below, intestinal contents are displaced both proximally and distally during the contraction and may, in fact, move cranially during the period of relaxation. Such contractions appear to divide the bowel into segments, which accounts for the term segmentation given to the process. Segmentation serves to mix and locally circulate the intestinal contents. Segmentation primarily involves circular smooth muscle contraction (Fig. 1-10).
Peristalsis
The small intestine is also capable of eliciting a highly coordinated contractile response that is propulsive in nature. When the bowel is distended by a bolus of food there is contraction cranial to and relaxation caudal to the point of distention. The neurotransmitters involved in the cranial contraction are ACh and SP, and the neurotransmitters involved in the caudal relaxation are VIP and nitric oxide (NO). These events tend to move the material caudally. Short-segment peristalsis of the bowel is the norm in dogs and cats (Fig. 1-10). If short-segment peristalsis occurs sequentially, it can propel a bolus the entire length of the gut in a short period of time. This peristaltic response, first characterized by Bayliss and Starling, is referred to as the law of the intestine, and is less frequent than short-segment peristalsis.
Intestinointestinal Inhibition
If an area of the bowel is grossly distended, contractile activity in the rest of the bowel is inhibited. This reflex prevents the movement of ingesta into more distal segments of intestine that have been severely distended or obstructed. This reflex is mediated by the extrinsic (autonomic) nervous system.30
Colon
The colon serves two important functions: (a) extraction of water and electrolytes from the luminal contents in the ascending and transverse colon and (b) storage of feces and control of defecation in the descending colon.31 This specialization of function has been attributed to regional differences in colonic motility patterns. Electrical slow-wave frequency and rhythmic phasic contractions are slower in the proximal colon (cecum and ascending colon), thus facilitating extraction of water from the fecal mass by diffusion and active transport. Retrograde giant contractions and anti-peristalsis further facilitate mixing of contents in the proximal colon. In contrast, motility of the distal colon (transverse and descending colon) is characterized primarily by migrating spike bursts and powerful giant migrating contractions that propagate the fecal mass toward the rectum (Fig. 1-11).31,32
Gallbladder
During fasting, the gallbladder is relaxed and stores bile. When feeding resumes, hormonal (cholecystokinin) and neural (acetylcholine) mechanisms stimulate simultaneous gallbladder contraction and sphincter of Oddi relaxation, thereby facilitating emptying of bile into the small intestine.33
Secretion
Salivary Gland
Dogs and cats both have four sets of salivary glands: parotid, mandibular, sublingual, and zygomatic, all under autonomic regulation. The structural unit of the salivary gland is the salivon, consisting of acinar cells, which secrete the initial fluid and mucin; intercalated ducts, which form a short connection to the striated ducts; striated ducts, which are composed of epithelial cells and which modify the final ionic composition; and myoepithelial cells, which contract and serve to expel salivary fluid into the oropharynx (Fig. 1-12).
Salivary secretions have five major functions: (a) hydration for mastication and deglutition; (b) evaporative cooling; (c) secretion of immunoglobulin (Ig) A; (d) HCO3− (bicarbonate) buffering prior to the initiation of digestion; and (e) release of R factor binding proteins for vitamin B12 transport.34,35 Salivary amylase, which initiates starch digestion in man, is not found in dog or cat saliva.34 Primary secretion from the acinus contains Na+, Cl−, and HCO3− actively transported from the blood to gland and duct. As saliva is transported down the collecting ducts, it is further modified by exchange of Na+ for H+ and K+ and active reabsorption of Na+ and Cl−. At high flow rates, the salivary composition will resemble the primary secretion because there is less time for modification along the ducts (Fig. 1-13).34
Stomach
As a secretory organ, the stomach has several major functions, including initiation of protein digestion; inactivation of ingested bacteria, viruses, and parasites; secretion and binding of intrinsic factor to vitamin B12; absorption of ferric iron; hormonal regulation of gastric and pancreatic secretions; mucous lubrication of the gastric contents; and protection of the gastric mucosa from the caustic effects of protons and pepsins.36 The stomach accomplishes these diverse functions through the secretions of the gastric pit, the structural unit of the gastric mucosa. Gastric pits are branched tubular invaginations bearing multiple cell types and having multiple secretory functions: (a) parietal or oxyntic cells secrete protons and intrinsic factor; (b) chief cells secrete pepsinogens; (c) endocrine cells secrete the hormones gastrin and ghrelin; (d) surface epithelial cells secrete HCO3− and prostanoids; (e) neck and surface mucous cells secrete mucus containing sulfated glycoproteins; and (f) enterochromaffin cells secrete histamine (Table 1-3, Fig. 1-14).36
Table 1-3 Cell Types, Secretions, and Functions in the Gastric Mucosa
Cell types | Secretion | Function |
---|---|---|
Parietal (oxyntic) cells | Protons | Activates pepsinogens |
Intrinsic factor | Binds vitamin B12 | |
Chief cells | Pepsinogen | Peptide bond hydrolysis |
Mucous cells | Sulfated glycoproteins | Unstirred layer |
Pepsinogen | Peptide bond hydrolysis | |
Endocrine cells | Gastrin | Stimulates H+ secretion |
Ghrelin | Stimulates feeding | |
Enterochromaffin cells | Histamine | Stimulates H+ secretion |
Epithelial cells | HCO3− | Neutralizes H+ |
Prostanoids | Gastric mucosal barrier |
Cell Biology of Gastric Acid Secretion
Secretion of hydrochloric acid (HCl) is the major function of the parietal cells. H+ ion secretion acidifies the gastric contents thereby permitting the conversion of inactive pepsinogens to active pepsins. Parietal cells generate protons through carbonic acid (H2CO3) synthesis and dissociation into H+ and HCO3−.37 Protons are actively secreted at the apical membrane along with Cl− into the lumen of the stomach in exchange for K+. An apical membrane H+,K+-ATPase actively transports H+ and K+ against their electrochemical gradients.37 HCO3− is absorbed from the parietal cell into the blood via a Cl−–HCO3− exchanger at the basolateral membrane. The absorbed HCO3− is responsible for the alkaline tide that is observed postfeeding. Eventually, this HCO3− will be resecreted into the GI tract in pancreatic and biliary secretions.
Physiology of Gastric Acid Secretion
The basal, cephalic, gastric, and intestinal phases of gastric acid secretion36 were first characterized by Pavlov in his now-classic work on conditioned reflexes in the dog at the turn of the last century. Basal phase secretion is that small amount of gastric acid secretion that takes place in the absence of anticipation or physiologic stimulus. Along with the MMC, basal acid secretion serves to moderate bacterial flora and to degrade indigestible solids in the interdigestive state. The cephalic phase of gastric acid secretion results from olfactory, visual, and auditory cues, as well as from the mastication and swallowing of food. The cephalic phase accounts for 20% to 30% of the total acid secretory load and is mediated by vagal postganglionic neurons that stimulate parietal secretion directly or indirectly via gastrin release. The gastric phase accounts for 50% to 60% of the total acid secretory load and is mediated by the direct and indirect effects of gastric distention and amino acids on parietal and antral G cells (Fig. 1-15). The intestinal phase accounts for up to 10% of gastric acid secretion and is mediated by the effects of intraduodenal amino acids.
Pharmacology of Gastric Acid Secretion
Parietal cells are stimulated directly and indirectly by neural (acetylcholine), endocrine (gastrin), and paracrine (histamine) mechanisms.36 In a neural mechanism, depolarization of vagal postganglionic nerve fibers releases ACh which then binds to the muscarinic M3 receptor on parietal cells. Binding of ACh to the M3 receptor activates the phospholipase C, IP3, and diacylglycerol (DAG) signal transduction pathway whose final pathway is the apical H+,K+-ATPase (Fig. 1-16). In a concurrent paracrine mechanism, enterochromaffin cells release histamine into the extracellular space where it binds to a histamine H2 receptor on parietal cells. Binding of histamine to the H2 receptor activates the adenylate cyclase, cyclic adenosine monophosphate (cAMP), protein kinase A signal transduction pathway, leading to activation of the final common pathway—the apical H+,K+-ATPase. Coincident with the neural and paracrine effects, gastrin is released from antral G cells in response to ACh, gastrin-releasing peptide, and amino acids. Gastrin circulates as a GI hormone having a primary effect of binding gastrin (or CCKB) receptors on gastric parietal cells. Like ACh, gastrin stimulates parietal cell H+ secretion through the IP3/DAG/Ca2+ second messenger system. Inhibition of parietal cell H+ secretion is mediated primarily via the paracrine effects of somatostatin, prostaglandins, and adenosine. All three paracrines inhibit parietal cell adenylate cyclase and cAMP production. Somatostatin has the additional effect of both inhibiting histamine release from enterochromaffin cells and gastrin release from antral G cells.
Gastric Pepsinogen Secretion
Chief cells and mucous cells secrete pepsinogen during the cephalic and gastric phases of gastric secretion.38 H+ ions secreted by the gastric parietal cells facilitate the conversion of inactive pepsinogen to active pepsin. Pepsin is optimally active at a pH of 1 to 2, and denatures at pH >5.0. Pepsin preferentially hydrolyzes peptide bonds containing aromatic amino acids such as phenylalanine, tryptophan, and tyrosine.
Gastric Intrinsic Factor Secretion
Intrinsic factor (IF) is a glycoprotein secreted by gastric parietal cells that serves to bind and transport vitamin B12 (cobalamin) to absorptive sites in the distal ileum. IF is produced by parietal cells in the dog, but not in the cat.39,40 Pancreatic ductal cells are an alternate source of IF synthesis in both species, exclusively so in the cat.40
Gastric Mucus Secretion and the Gastric Mucosal Barrier
Sulfated glycoproteins produced by neck and surface epithelial cells are incorporated into the unstirred layer adherent to the gastric mucosa. Mucus serves to trap H+ ion in this unstirred layer thereby preventing back-diffusion into the mucosa and submucosa. Other factors contributing to this gastric mucosal barrier include epithelial HCO3− secretion and acid neutralization, rapid epithelial migration to reconstitute the overlying epithelium, blood flow in the lamina propria to transport and buffer absorbed H+, and the multiple cytoprotective effects of endogenous prostaglandins.41
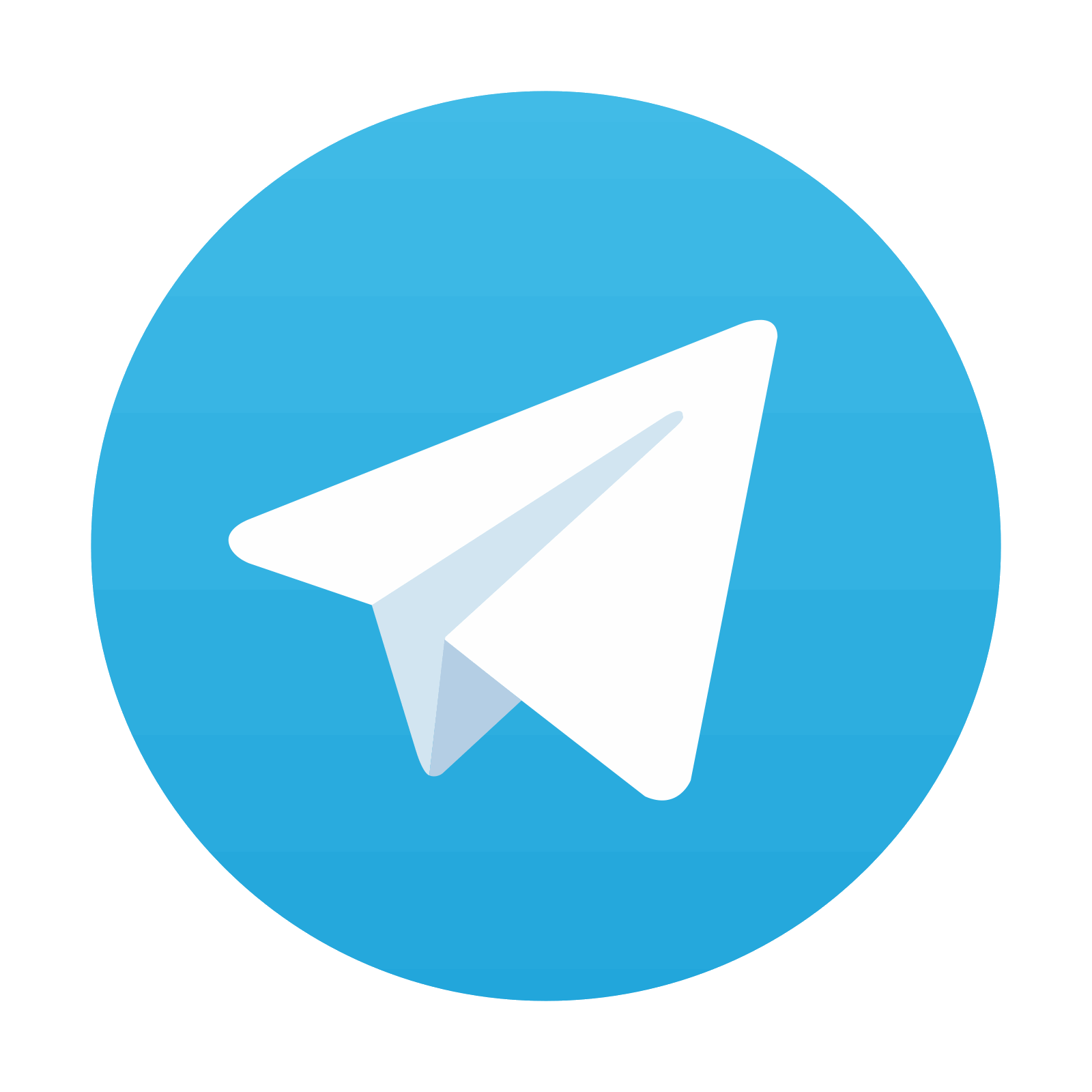
Stay updated, free articles. Join our Telegram channel
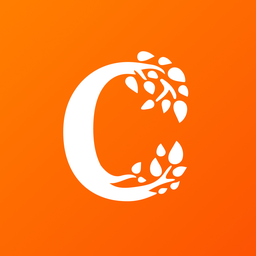
Full access? Get Clinical Tree
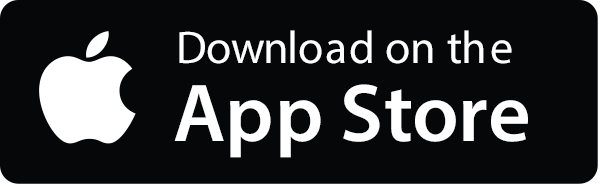
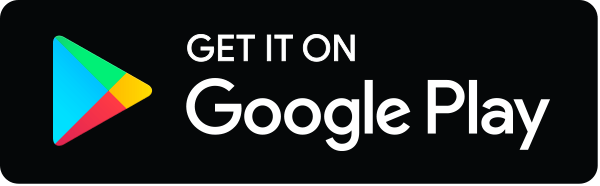