CHAPTER 8 The classic functional subunit of the liver is the hepatic lobule, a hexagonal structure, 1 to 2 mm wide. At the center, the lobule has a central vein (also termed the terminal hepatic venule), which is a tributary of the hepatic vein, and at the angles of the hexagon, it has portal tracts (Fig. 8-1). The portal tracts contain bile ducts, branches of the portal vein, the hepatic artery, nerves, and lymph vessels, all supported by a collagenous stroma (Fig. 8-2). The limiting plate, a discontinuous border of hepatocytes, forms the outer boundary of the portal tract. Blood flows into the sinusoids from the terminal distributing branches of the hepatic artery and portal veins that leave the portal tracts and form an outer perimeter of the lobule (see Figs. 8-1 and 8-2). Portal blood and hepatic arterial blood mix in the sinusoids. Blood drains from the sinusoids into the central veins and to progressively larger sublobular veins and then into the hepatic veins. Fig. 8-1 Schematic views of the microscopic and functional organization of the liver. Fig. 8-2 Liver, hepatic lobules, normal dog. Alternatively, when the liver is viewed as a bile-secreting gland, the acinus is the anatomic subunit of the hepatic parenchyma. Terminal afferent branches (penetrating vessels) of the portal vein and hepatic artery project into the parenchyma, like branches from the trunk of a tree, forming the long axis of the diamond-shaped acinus. Thus terminal afferent branches of the portal vein and hepatic artery are at the center of the acinus and the terminal hepatic venule is located at the periphery. Each terminal hepatic venule (central vein) receives blood from several acini. There are three zones within the acinus. Zone 1 is closest to the afferent blood coming from the hepatic artery and the portal vein. Zone 2 is peripheral to zone 1, and zone 3 borders the terminal hepatic venule (see Fig. 8-1). In this anatomic unit, bile flow begins in the canaliculi of the hepatocytes in zone 3 and flows through zones 2 and 1 then into the interlobular bile ducts in the portal areas. The ultrastructural appearance of hepatocytes reflects the cell’s active metabolism, bile secretion, and close contact with the plasma (Web Fig. 8-1). The surface of the hepatocyte that faces the lumen of the sinusoids contains an abundance of microvilli, which increase the hepatocytic surface area and facilitate uptake of plasma-borne substances, such as bilirubin and amino acids, and the secretion of products of hepatic metabolism, such as lipoproteins and clotting factors. Basolateral aspects of hepatocytes are characterized by the presence of canaliculi, modified portions of the cell membrane in two adjacent hepatocytes, which form a lumen for bile secretion. The cytoplasm contains glycogen and a variety of organelles, including numerous mitochondria, lysosomes, and abundant smooth and rough endoplasmic reticulum. Web Fig. 8-1 Hepatocyte, ultrastructure, liver, normal dog. Within the liver, hepatocytes are arranged in one-cell-thick branching plates, which extend radially from the terminal hepatic venule. Hepatic plates are separated by vascular sinusoids. Blood from the terminal afferent branches of the hepatic artery and portal vein mixes in the hepatic sinusoids and flows to the terminal hepatic venule. Hepatic sinusoids differ from capillaries in that they are lined by discontinuous endothelial cells that lack a typical basement membrane (Fig. 8-3), whereas capillaries have a continuous endothelial lining and are ensheathed in the basement membrane. The sinusoids are critical for appropriate hepatic function. The architecture of the sinusoids enables efficient uptake of plasma constituents by hepatocytes and facilitates hepatocellular secretion. A fine scaffold of electron lucent basement membrane that contains collagen types III, IV, and XVIII, and other extracellular matrix (ECM) components supports the sinusoidal endothelial cells (Web Fig. 8-2; see Fig. 8-3). These elements collectively make up the “reticulin” of the liver (Fig. 8-4). Fig. 8-3 Schematic diagram of the hepatic sinusoid. Fig. 8-4 Reticulin fibers (reticulin stain), hepatic extracellular matrix, liver, normal dog. Web Fig. 8-2 Hepatic sinusoids, liver, normal dog. Although blood cells are normally excluded from the space of Disse because they are too large to pass through endothelial gaps, the modified endothelial cells and basement membrane permit plasma to pass freely into a gap between the endothelial cells and the hepatocytes (see Fig. 8-3). This critical anatomic feature of the liver is termed the space of Disse. Within this space, plasma constituents come into contact with the luminal surface of the hepatocytes. This surface of the hepatocytes is characterized by the presence of numerous microvilli, which increase the surface area of the hepatocytes and facilitate uptake of a variety of plasma-borne substances, as well secretion of synthesized products. Any damage to this area has significant impact on hepatic function. The lumen of the sinusoids contains hepatic macrophages, termed Kupffer cells (Fig. 8-5). These cells are members of the monocyte-macrophage system, and they clear infectious agents and senescent cells, such as erythrocytes, particulate material, endotoxin, and other substances, from the sinusoidal blood. They are mobile and able to migrate along the sinusoids and into areas of tissue injury and regional lymph nodes. Kupffer cells are involved in cytokine-driven interactions with hepatocytes, endothelial cells, and the stellate cells discussed later. They can express class II histocompatibility antigens and function as antigen-presenting cells, although they are not as efficient as the macrophages in other tissue. Phagocytosis and clearance of immune complexes are the primary roles of Kupffer cells. Kupffer cells are derived from in situ replication and recruitment of blood-borne monocytes. Fig. 8-5 Kupffer cells, carbon particle uptake, liver, normal calf. Hepatic stellate cells (also termed lipocytes or Ito cells) are found within the space of Disse and between hepatocytes at the edge of the space of Disse (Web Fig. 8-3). Normally, hepatic stellate cells are primarily responsible for storing vitamin A in their characteristic cytoplasmic vacuoles. During hepatic injury, hepatic stellate cells alter their morphology and their function. These activated hepatic stellate cells lose their vitamin A content and synthesize collagen and other ECM components that lead to hepatic fibrosis. Web Fig. 8-3 Liver, normal dog. The liver performs many critical functions, including the following: Bilirubin Metabolism: Excretion of bile is the main exocrine function of the liver. Bile is composed of water, cholesterol, bile acids, bilirubin, inorganic ions, and other constituents. Bile formation is continuous, but the rate of secretion can vary significantly. There are three major purposes for bile synthesis. The first purpose is excretory; many of the body’s waste products, such as surplus cholesterol, bilirubin, and metabolized xenobiotics, are eliminated in bile. The second purpose is the facilitation of digestion; bile acids secreted into the intestine aid in the digestion of lipids within the intestine. The third is to provide buffers to neutralize the acid pH of the ingesta. Bilirubin, a major component of bile, is produced from the metabolic degradation of hemoglobin, and to a lesser extent, other heme proteins including myoglobin and the hepatic hemoproteins, such as cytochromes (Fig. 8-6). The majority of bilirubin is derived from normal extrahepatic breakdown of senescent erythrocytes in cells of the monocyte-macrophage phagocytic cell series. Senescent erythrocytes normally are phagocytosed by macrophages of the spleen, bone marrow, and liver. Within the phagocyte, the globin portion is degraded and the constituents are returned to the amino acid pool. The heme iron is transferred to iron-binding proteins, such as transferrin, for recycling. The remaining portion of heme is first oxidized by heme oxygenase to biliverdin. In the next metabolic step, biliverdin reductase converts biliverdin to bilirubin. Subsequently, the bilirubin, which is poorly soluble in an aqueous medium, is then released into the blood in its unconjugated form and bound to albumin to increase its solubility in plasma. Fig. 8-6 Schematic diagram of bilirubin metabolism and elimination (as depicted in human beings). Bile Acid Metabolism: The three principal functions of bile acids, important constituents of bile, are maintenance of cholesterol homeostasis, stimulation of bile flow and digestion, and absorption of fats and fat-soluble vitamins. Bile acids are synthesized in the liver from cholesterol and are conjugated to glycine or taurine to facilitate their interaction with other components of bile and to prevent precipitation into calculi when they are secreted into the bile. The major bile acids are cholic acid and chenodeoxycholic acid, but there are various types and proportions of bile acids found in different species. Bile acids are actively secreted into the bile canaliculi from the hepatocyte cytoplasm by specific intramembranous molecular pumps against a concentration gradient, which creates an osmotic gradient, stimulating the inflow of water and solutes into the bile canaliculi. Conjugated bile acids are therefore the principal physiologic stimulus for bile production through a process termed bile acid–dependent flow. Bile acids are effective detergents that assist in the digestion of lipids within the intestine and increasing the solubility of lipids secreted into the bile. The quantities of bile acids required far exceed the liver’s capacity to produce them. For this reason, bile acids are avidly reabsorbed from the ileum, extracted from the portal blood, and resecreted into bile via a process known as enterohepatic circulation. This is a very efficient system. As much as 95% of secreted bile acids are recycled, and the proportion of reabsorbed bile acids in the liver greatly exceeds that of recently synthesized bile acids; bile acids may be recycled 15 times a day. Interruption of this process results in fat malabsorption and a deficiency of fat-soluble vitamins. Carbohydrate Metabolism: The liver has an important role in the regulation of plasma glucose concentrations. After eating, the liver removes carbohydrates (i.e., glucose, fructose) from the plasma and stores them as glycogen or fatty acids. In periods of need, energy balance is maintained by glycolysis of stored glycogen or by gluconeogenesis. Production of energy by oxidative phosphorylation and β-oxidation of fatty acids in hepatic mitochondria is used to sustain the activities of the hepatocyte. Lipid Metabolism: The liver plays a central role in lipid metabolism. It is involved in the production and degradation of plasma lipids such as cholesterol, triglycerides, phospholipids, and lipoproteins. Cholesterol is synthesized, secreted, and degraded by hepatocytes. Hepatocytes can synthesize fatty acids when energy levels are high, and they can oxidize fatty acids as an energy source when necessary. Xenobiotic Metabolism: Foreign substances (xenobiotics), such as many therapeutic drugs, insecticides, and endogenous substances—such as steroids that are lipophilic—require conversion to water-soluble forms for elimination from the body. The cytochrome p450 enzymes of the smooth endoplasmic reticulum of the hepatocytes serve as the major site of metabolism of these substances in preparation for excretion in bile or urine. This process is discussed in detail in the section on toxic liver injury. Protein Synthesis: Synthesis of the majority of plasma proteins, mainly within the rough endoplasmic reticulum, is a principal function of the liver. Proteins produced in the liver include plasma proteins, such as albumin; a variety of transport proteins; lipoproteins; clotting factors II, V, and VII to XIII; fibrinolysis proteins; some acute phase proteins; and components of the complement system. The liver is responsible for synthesis of approximately 15% of body proteins. Immune Function: The liver has a significant immune function. It is involved in systemic, local, and mucosal immunity. Hepatocytes participate in the response to systemic inflammation through the synthesis and release of acute phase proteins. Approximately 10% of the cells in the liver belong to the adaptive immune system (T and B lymphocytes) or the innate immune system (Kupffer cells, natural killer lymphocytes, and natural killer T lymphocytes). Compared with other organs, the liver is particularly enriched with cells of the innate immune system, likely a result of the fact it is the site where foreign antigens from the gastrointestinal tract first encounter the innate immune system defenses. The liver contains the largest pool of mononuclear phagocytes and natural killer cells in the body in most species. The Kupffer cells lining the sinusoids provide the first line of defense against infectious agents, endotoxin, and foreign material absorbed from the intestines before they gain access to the systemic circulation. Most blood-borne foreign material is cleared by Kupffer cells in all domestic species, except members of the Order Artiodactyla (pigs, goats, and cattle), in which this function is performed by intravascular macrophages in the pulmonary alveolar capillaries. The liver is also involved in transport of secretory immunoglobulin A (IgA), the primary immunoglobulin of the mucosal surfaces, from plasma cells and recirculation into the biliary tree and intestine. Classic apoptosis is triggered by an interaction between tumor necrosis factor-α (TNF-α) or Fas ligand and specific receptors on the cell membrane leading to caspase activation, although other pathways, including those involving mitochondrial cytochrome-c, have been identified. Apoptosis is recognized by the formation of acidophilic bodies, which are brightly eosinophilic, homogeneous, round structures that can be found between hepatocytes, within the lumen of sinusoids, or within macrophages or hepatocytes. A detailed review of cell death is beyond the scope of this section but is covered in Chapter 1. However, recent evidence reveals that there may some overlap between necrosis and apoptosis, depending on the cell type and the type and dose of injurious agent. Thus both hepatic necrosis and apoptosis can be produced by the same agent and can occur in the same liver. Patterns of Hepatocellular Degeneration and Necrosis: Although the liver is subjected to a wide variety of different insults, the cellular degeneration and/or necrosis that results invariably occurs in one of following three morphologic patterns: • Random hepatocellular degeneration and/or necrosis • Zonal hepatocellular degeneration and/or necrosis Random Hepatocellular Degeneration: Random hepatocellular degeneration and/or necrosis is characterized by the presence either of single cell necrosis throughout the liver or multifocal areas of necrotic hepatocytes. These areas are scattered randomly throughout the liver; there is no predictable location within a lobule. This pattern is typical of many infectious agents, including viruses, bacteria, and certain protozoa. Lesions may be obvious grossly as discrete, pale, or less often, dark red foci that are sharply delineated from the adjacent parenchyma (Fig. 8-7, A). The size of such foci is variable, ranging from tiny (<1 mm) to several millimeters. Hepatocytes in affected areas are either degenerated or necrotic because of the injurious effects of the infectious agents and the stage of the process (Fig. 8-7, B). Fig. 8-7 Random hepatocellular injury, liver. Zonal Hepatocellular Degeneration and/or Necrosis: Zonal hepatocellular degeneration and/or necrosis or as it is more simply termed, zonal change, affects hepatocytes within defined areas of the hepatic lobule. The zones are centrilobular (periacinar), midzonal (between centrilobular and periportal areas), or periportal (centroacinar) areas. Extensive zonal change within the liver, regardless of location within the lobule, typically produces a liver that is pale and modestly enlarged with rounded margins, has increased friability, and characteristically has an enhanced lobular pattern on the capsular and cut surface of the organ (Fig. 8-8). Degenerated hepatocytes swell and when the majority of hepatocytes in a zone are affected, that portion of the lobule appears pale. In contrast, once the hepatocytes in a particular zone of the lobule have become necrotic, this results in dilation and congestion of sinusoids so that the affected zone appears red. Although zonal change typically produces an enhanced lobular pattern, microscopic examination is usually required to determine the type of zonal change. Specific forms of zonal change are described next. Fig. 8-8 Zonal hepatocellular injury, liver, horse. Centrilobular degeneration and necrosis: Centrilobular degeneration and necrosis of hepatocytes is particularly common (Fig. 8-9), as this portion of the lobule receives the least oxygenated blood and is therefore susceptible to hypoxia, and it has the greatest enzymatic activity (mixed-function oxidases) capable of activating compounds into toxic forms. Centrilobular necrosis can result from a precipitous and severe anemia or right side heart failure. Similarly, passive congestion of the liver results in hypoxia as a result of stasis of blood and produces atrophy of centrilobular hepatocytes. Fig. 8-9 Centrilobular necrosis, zonal hepatocellular injury, liver, pig. Paracentral (periacinar) cellular degeneration: Paracentral (periacinar) cellular degeneration involves only a wedge around the central vein because only the periphery of one acinus is affected, typically reflecting the action of a direct-acting toxin that requires bioactivation (Fig. 8-10) or severe, acute anemia. As several acini border on a single central vein (terminal hepatic venule), changes induced by hypoxia may not be present equally in all acini, and thus hepatocytes at the periphery of one acinus can have more severe change than those in adjacent acini. Fig. 8-10 Paracentral degeneration and necrosis, zonal hepatocellular injury, liver, cow. Midzonal degeneration and necrosis: Midzonal degeneration and necrosis are unusual lesions in domestic animals but have been reported in pigs and horses with aflatoxicosis and cats exposed to hexachlorophene (Fig. 8-11). Fig. 8-11 Midzonal necrosis, zonal hepatocellular injury, liver, horse. Periportal degeneration and necrosis: Periportal degeneration and necrosis are also uncommon but may occur following exposure to toxins, such as phosphorus, that do not require metabolism by mixed function oxidases (most active in the centrilobular hepatocytes) to cause injury (Fig. 8-12). Some of these compounds may be metabolized to injurious intermediates by cytoplasmic enzymes found in periportal hepatocytes. Alternatively, some of these toxins may not require metabolism and produce hepatocyte injury in the first hepatocytes that they encounter as they flow from the portal areas. Fig. 8-12 Periportal necrosis, zonal hepatocellular injury, liver, horse. Bridging necrosis: Bridging necrosis is the result of confluence of areas of necrosis. Bridging may link centrilobular areas (central bridging) or centrilobular areas to periportal areas (Fig. 8-13). Fig. 8-13 Bridging necrosis, zonal hepatocellular injury, liver. Massive Necrosis: Massive necrosis is not necessarily, as the name might be taken to imply, necrosis of the entire liver, but rather the term describes necrosis of an entire hepatic lobule or contiguous lobules (Fig. 8-14, A). All hepatocytes within affected lobules are necrotic. The gross appearance of the liver varies with the maturity of the lesion. If, in acute cases, the majority of the parenchyma is affected, the liver may initially be modestly increased in size with a smooth external surface and dark parenchyma because of extensive congestion. At first, necrotic hepatocytes lyse and the residual stroma becomes condensed. Regeneration does not occur because virtually all hepatocytes in the lobule are affected. Microscopically, affected areas consist of blood-filled spaces within a connective tissue stroma devoid of hepatocytes (Fig. 8-14, B). Later in the course of the process, stellate cells or other ECM–producing cells from the portal and centrilobular areas that may survive or migrate to the site of injury contribute new collagen (collagen I, in particular). The final result is collapse of the lobule and replacement of the lost hepatic parenchyma with a scar consisting of condensed stroma, including variable amounts and types of collagen. Grossly the liver may be smaller than normal with a wrinkled capsule. Partial involvement of the liver is characterized by depressed areas of parenchymal necrosis and vascular congestion scattered throughout the organ. Fig. 8-14 Massive necrosis, liver. Hepatic injury is frequently manifested as an increased concentration of conjugated or unconjugated bilirubin in blood called hyperbilirubinemia. High concentrations of bilirubin (>approximately 2 mg/dL) can produce jaundice (icterus), a yellow discoloration of tissue that is especially evident in tissue rich in elastin such as the aorta and sclera (Fig. 8-15). This concentration is within the reference range for horses, so horses may not be hyperbilirubinemic at this level. However, in other species, hyperbilirubinemia can occur once the concentration exceeds 0.5 mg/dL (dog) and therefore the patient is hyperbilirubinemic, but icterus will not be detected until it exceeds 2 mg/dL. Maximal accumulation of bilirubin in tissues takes about 2 days and explains why some animals with acute hepatic failure may have only slight icterus. Fig. 8-15 Icterus, dog. The causes of hyperbilirubinemia include the following: 1. Overproduction of bilirubin as a consequence of hemolysis, particularly severe intravascular hemolysis, which overwhelms the liver’s capacity to remove bilirubin from the plasma and to secrete conjugated bilirubin into bile. The destruction of damaged red blood cells by extravascular hemolysis can also increase the burden of bilirubin presented to the liver. Hypoxia secondary to anemia may also play a role. Decreased uptake, conjugation, or secretion of bilirubin by hepatocytes arising as a consequence of severe, diffuse hepatic disease, whether acute or chronic. 2. Reduced outflow of bile (cholestasis). Cholestasis is defined as a defect in bile secretory mechanisms that leads to an accumulation in the blood of substances normally excreted into the bile. Cholestasis occurs as a consequence of either obstruction of the biliary ducts (extrahepatic cholestasis) or impairment of bile flow within canaliculi (intrahepatic cholestasis). Cholestasis can be divided into two types: intrahepatic and extrahepatic. Intrahepatic cholestasis can result from (1) a wide spectrum of liver injury affecting the ability of hepatocytes to metabolize and excrete bile; (2) hemolysis, which produces an abundance of bilirubin for excretion and diminishes the supply of oxygen for hepatocyte metabolism; or (3) inherited abnormalities of bile synthesis that inhibit the excretion of bile. Extrahepatic cholestasis is produced by obstruction of the extrahepatic bile ducts. This can occur by intraluminal obstruction (calculi or possibly parasites) or extraluminal means, including neoplasia or adjacent inflammation, often involving the pancreas. Cholestasis, if sufficiently severe, can produce a greenish brown discoloration to the liver (Fig. 8-16, A). Fig. 8-16 Hepatic bilirubin retention, icterus, liver. Histologically, acute intrahepatic cholestasis is characterized by formation of bile plugs within canaliculi (Fig. 8-16, B). As intrahepatic cholestasis becomes more chronic, bile that has been released from hepatocytes is taken up by Kupffer cells and can be detected within their cytoplasm. Acute extrahepatic obstruction is characterized by edema of the portal areas, a mild neutrophilic inflammatory cell infiltrate, and a proliferative reaction by the biliary epithelium of the bile ducts. In chronic extrahepatic biliary obstruction, portal areas are enlarged by deposition of fibrosis, and there is a prominent laminar, circumferential fibrosis of bile ducts (Fig. 8-17). Biliary hyperplasia characterized by proliferation of small-caliber bile ducts is often prominent. Pigmented macrophages, containing bile, and mixed inflammatory infiltrates are also present. In severe cases, bridging fibrosis connecting portal tracts may develop. Fig. 8-17 Chronic extrahepatic cholestasis, cholelithiasis, liver, horse.
Hepatobiliary System and Exocrine Pancreas
Liver and Intrahepatic Biliary System
Macroscopic and Microscopic Structure
A, Microscopic organization of the liver. A central vein is located in the center of the lobule with plates of hepatocytes arranged radially. Branches of the portal vein and hepatic artery are located on the periphery of the lobule, and blood from both perfuses the sinusoids. Peripherally located bile ducts drain the bile canaliculi that run between hepatocytes. B, Functional organization of the liver. Both the lobule and the acinus are represented. The lobule is a hexagonal unit with portal areas at the margin and a terminal hepatic vein (central vein) at the center. The lobule is divided into the periportal, midzonal, and centrilobular areas. The acinus is a diamond-shaped structure with the distributing branches of the vessels from the portal areas as the center of the structure. Zone 1 of the acinus is closest to the afferent blood supply, and zone 3 is at the tip of the diamond-shaped structure, close to the terminal hepatic vein. Zone 2 is between Zones 1 and 3. (A from McCance KL, Huether SE: Pathophysiology: the biologic basis for diseases in adults and children, ed 6, St Louis, 2010, Mosby. B from Kumar V, Abbas AK, Fausto N: Robbins & Cotran pathologic basis of disease, ed 7, Philadelphia, 2005, Saunders.)
A, Low magnification. A central vein (C) is located in the center of the lobule. Branches of the portal vein, hepatic artery, bile duct, and lymphatic vessels are located on the periphery of the lobule in portal tracts (P) (also Fig. 8-2, C). H&E stain. B, Higher magnification. Plates of hepatocytes arranged radially between portal tracts (P) to a central vein (C). H&E stain. C, Higher magnification, portal tract. The normal portal tract contains the hepatic artery (HA), bile duct (BD), portal vein (PV), and several lymphatic vessels (LV). These structures are surrounded by a collagenous extracellular matrix that forms an abrupt border with a circumferential row of hepatocytes, termed the limiting plate (LP—dotted line). Note that the profile of the portal vein is typically larger than those of the hepatic artery and bile duct. H&E stain. (A and C courtesy Dr. J.M. Cullen, College of Veterinary Medicine, North Carolina State University. B courtesy Dr. J.F. Zachary, College of Veterinary Medicine, University of Illinois.)
A, Features to note are the nucleus (N), mitochondria (M), secondary lysosomes (2L), glycogen (G), rough endoplasmic reticulum (RER), Golgi (arrow), bile canaliculus (BC), and free ribosomes (R). Note desmosomes on both sides of the bile canaliculus (arrowheads). TEM. Uranyl citrate and lead acetate stain. B, Higher magnification of bile canaliculus (BC). Note the microvilli projecting into the lumen of the canaliculus. TCM uranyl citrate and lead acetate stain. (A and B courtesy Dr. V. Meador, Covance Inc.)
The vascular lumen is lined by discontinuous capillaries. Kupffer cells rest on the endothelial cells and project into the sinusoid. Between the endothelial cells and the hepatocytes is a gap called the space of Disse. Microvilli extending from the luminal aspect of the hepatocytes are found in this space. Hepatic stellate cells are situated within the space of Disse and extend between hepatocytes. (Schematic based on concepts in Friedman SL: J Biol Chem 275:2247-2250, 2000; and Crawford JM: Curr Op Gastroenterol 13:175-185, 1997.)
This stain reveals “reticulin” (black), composed of extracellular matrix found within the space of Disse that forms the scaffolding of the hepatic parenchyma. Note the radial arrangements of the hepatic plates and the single hepatocyte thickness of the plates. A central vein is evident in the center of the image. Gordon and Sweet’s reticulin stain with a nuclear fast red counterstain. (Courtesy Dr. M.D. McGavin, College of Veterinary Medicine, University of Tennessee.)
A sinusoid containing erythrocytes (E) can be seen in the upper right-hand portion of the figure. The margins of the sinusoid are lined with discontinuous capillaries (arrowheads). The space of Disse (double-headed arrows) lies between the endothelial cells and the hepatocytes. Microvilli (MV) project from the hepatocytes into the space of Disse. Rough endoplasmic reticulum (RER) and lipid droplets (L) can be seen in the hepatocyte cytoplasm. TEM. Uranyl citrate and lead acetate stain. (Courtesy Dr. V. Meador, Covance Inc.)
Carbon particles injected into the portal vein have been phagocytosed by Kupffer cells (arrows), making them more easily detectable along the sinusoids of the liver. Nuclear fast red stain. (Courtesy Dr. M.D. McGavin, College of Veterinary Medicine, University of Tennessee.)
A hepatic stellate cell (S) with its characteristic cytoplasmic lipid vacuoles (L) is found adjacent to hepatocytes (H) and within the space of Disse. Bundles of collagen (C) are found at the margins of the cells. Erythrocytes (E) are within the sinusoidal lumen. TEM. Uranyl citrate and lead acetate stain. (Courtesy Dr. V. Meador, Covance Inc.)
Normal Function
1, Normal bilirubin production from heme (0.2 to 0.3 g per day) is derived primarily from the breakdown of senescent circulating erythrocytes, with a minor contribution from degradation of tissue heme-containing proteins. 2, Extrahepatic bilirubin is bound to serum albumin and delivered to the liver. 3, Hepatocellular uptake. 4, Glucuronidation in the endoplasmic reticulum generates bilirubin monoglucuronides and diglucuronides, which are water soluble and readily excreted into bile. 5, Gut bacteria deconjugate the bilirubin and degrade it to colorless urobilinogens. The urobilinogens and the residue of intact pigments are excreted in the feces, with some reabsorption and excretion into urine. Residual urobilinogen is metabolized by bacteria into the brown pigment stercobilin, imparting the typical color to feces.
Response of the Liver to Injury
A, Equine herpes virus infection, foal. Random foci of viral-induced lytic necrosis. B, Salmonellosis, focal necrosis and inflammation, pig. The random pattern of hepatocellular necrosis and inflammation (arrows) caused by septicemic Salmonella spp. can also be seen within the hepatic lobules. H&E stain. (A courtesy Drs. J. King and L. Roth, College of Veterinary Medicine, Cornell University. B courtesy Dr. J.M. Cullen, College of Veterinary Medicine, North Carolina State University.)
Accentuation of the normal lobular pattern is evident on the capsular surface of the liver. This is not a specific change, as it may be associated with zonal hepatocellular degeneration and/or necrosis (regardless of lobular location), passive congestion, or diffuse cellular infiltration of the portal and periportal areas (often reflecting hepatic involvement of hematopoietic neoplasms, such as lymphoma and myeloproliferative disorders). (Courtesy Dr. J. King, College of Veterinary Medicine, Cornell University.)
Centrilobular necrosis (periacinar or zone 3) is characterized by a circumferential zone of hepatocellular necrosis surrounding the terminal hepatic venule (central vein [C]). H&E stain. (Courtesy Dr. M.D. McGavin, College of Veterinary Medicine, University of Tennessee.)
Rather than a pattern of complete circumferential necrosis, a wedge-shaped area of hepatocytes is damaged. In this case, the paracentral lesion consists of necrotic hepatocytes to the left and other hepatocytes with hydropic degeneration. This wedge is the apex of the diamond-shaped liver acinus (zone 3) and reflects the partitioning of the lobule based on the inflow of blood from each of the individual portal tracts that surround the lobule. This change can be seen as an early manifestation of hepatic hypoxia in animals with anemia or right-sided heart failure and precedes centrilobular necrosis. C, Central vein. H&E stain. (Courtesy Dr. M.D. McGavin, College of Veterinary Medicine, University of Tennessee.)
Midzonal necrosis is the least common pattern of hepatic injury. Hepatocytes in the middle portion of the lobule (zone 2) are affected and hepatocytes in the other regions are spared. C, Central vein; P, portal vein. H&E stain. (Courtesy Dr. M.D. McGavin, College of Veterinary Medicine, University of Tennessee.)
Periportal (or zone 1) necrosis is an uncommon pattern of hepatocellular injury. Hepatocytes surrounding the portal tracts (P) are affected. H&E stain. (Courtesy Dr. M.D. McGavin, College of Veterinary Medicine, University of Tennessee.)
Bridging necrosis refers to a pattern characterized by connection of areas of necrosis between different lobules. Three patterns of bridging necrosis are recognized: central to central, as seen here; portal to portal; and central to portal. P, Portal area. H&E stain. (Courtesy Dr. M.D. McGavin, College of Veterinary Medicine, University of Tennessee.)
A, Pig, cut surface. Massive necrosis refers to a pattern of necrosis that involves an entire hepatic lobule, as seen here. B, Dog. The entire population of hepatocytes within the lobule has undergone necrosis. P, Portal area. H&E stain. (A courtesy Dr. D. Cho, College of Veterinary Medicine, Louisiana State University; and Noah’s Arkive, College of Veterinary Medicine, The University of Georgia. B courtesy Dr. J.M. Cullen, College of Veterinary Medicine, North Carolina State University.)
Disturbances of Bile Flow and Icterus
Icterus and jaundice are terms that refer to the yellow discoloration of tissue, by bilirubin, in this case evident in the fat and serosa. (Courtesy Dr. M.D. McGavin, College of Veterinary Medicine, University of Tennessee.)
A, Cat. The liver is markedly yellowed by retained bilirubin. B, Canalicular bilirubin, acute hemolytic anemia, calf. Acute hemolysis caused by babesiosis has led to a dramatic increase in bilirubin production and distention of canaliculi, clearly demonstrating the location of canaliculi between hepatocytes. H&E stain. (A courtesy College of Veterinary Medicine, University of Illinois. B courtesy Dr. M.D. McGavin, College of Veterinary Medicine, University of Tennessee.)
There is reduplication of bile ducts (arrows) and extensive fibrosis (F) throughout the portal tract (biliary fibrosis) as a consequence of prolonged stasis and subsequent leakage of bile. H&E stain. (Courtesy Dr. J.M. Cullen, College of Veterinary Medicine, North Carolina State University.)Stay updated, free articles. Join our Telegram channel
Full access? Get Clinical Tree
Hepatobiliary System and Exocrine Pancreas
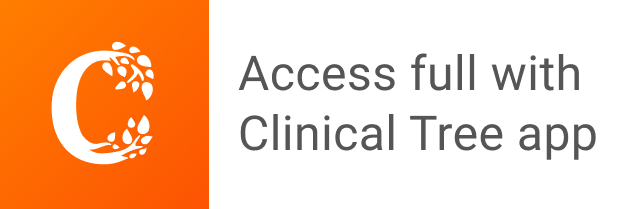