CHAPTER 13 The marrow cavity is interlaced with venous sinuses composed of a luminal layer of specialized endothelial cells and an abluminal layer of specialized fibroblasts known as adventitial reticular cells (Figs. 13-1 and 13-2). Hematopoiesis takes place between these venous sinuses in the so-called hematopoietic spaces. Sinusoidal endothelial cells function as a barrier and regulate traffic of chemicals and particles between the intravascular and extravascular spaces. Reticular cells extend cytoplasmic processes into the hematopoietic spaces, forming a scaffold that supports the hematopoietic cells. Osteoblasts, osteoclasts, and elongated flat cells line the endosteum. Stromal cells, presumably of fibroblast origin, provide structural support and produce extracellular matrix components such as proteoglycans, glycosaminoglycans, collagen, fibronectin, and others. Adipocytes, macrophages, lymphocytes, and plasma cells are also components of the hematopoietic microenvironment. • Hematopoietic tissue is highly proliferative. Billions of cells per kilogram of body weight are produced each day. • Pluripotent hematopoietic stem cells are a self-renewing population, giving rise to cells with committed differentiation programs, and are common ancestors of all blood cells. The process of hematopoietic differentiation is shown in Fig. 13-3. Fig. 13-3 Schematic illustration of the hematopoietic hierarchy in the bone marrow. • Hematopoietic cells undergo sequential divisions as they develop, so there are progressively higher numbers of cells as they mature. Cells also continue to mature after they have stopped dividing. Conceptually, it is helpful to consider cells in the bone marrow as belonging to mitotic and postmitotic compartments. Examples of developing hematopoietic cells are shown in Fig. 13-4. Fig. 13-4 Normal hematopoiesis, canine bone marrow aspirate. • Mature cells released into the blood circulation have different normal lifespans, varying from hours (neutrophils), to days (platelets), to months (erythrocytes), and to years (some lymphocytes). • The hematopoietic system is under exquisite local and systemic control and responds rapidly and predictably to various stimuli. • Production and turnover of blood cells are balanced so that numbers are maintained within normal ranges (steady-state kinetics) in healthy individuals. • Normally the bone marrow releases only mature cell types (and very low numbers of cells that are almost fully mature) into the circulation. In response to certain physiologic or pathologic stimuli, however, the bone marrow releases immature cells that are further back in the supply “pipeline.” The earliest erythroid precursor identifiable by routine light microscopy is the rubriblast, which undergoes maturational division to produce 8 to 32 progeny cells. Late-stage erythroid precursors known as metarubricytes extrude their nuclei and become reticulocytes, which are the cells at the differentiation stage immediately preceding the mature erythrocyte. Reticulocytes start maturing in the bone marrow and finish maturing in the peripheral blood circulation and the spleen. (Horses are an exception in that they do not release reticulocytes into circulation, even in situations of increased demand.) The normal transit time from rubriblast to mature erythrocyte is approximately 1 week. Unlike mature erythrocytes, which lack organelles, reticulocytes still contain ribosomes and mitochondria, mainly to support completion of hemoglobin synthesis. These remaining organelles impart a bluish-purple cast (polychromasia) to reticulocytes on routine blood smear examination and when stained with dye, such as new methylene blue, precipitate to form irregular aggregates of dark-staining material (Fig. 13-5). Cats also have a more mature form of reticulocyte in circulation, the punctate reticulocyte, which has a much finer staining pattern when stained with new methylene blue and does not appear bluish-purple (polychromatophilic) on routine blood smear examination (see the section Anemia). Fig. 13-5 Reticulocytosis, canine blood smears. One of the key properties of erythrocytes is deformability; they change shape as they move through the microvasculature. This deformability is a function of interactions between the cell membrane, the cytoskeleton, and intracellular contents. Mature mammalian erythrocytes lack nuclei and organelles and are thus incapable of transcription, translation, and oxidative metabolism. However, they do require energy for various functions, including maintenance of shape and deformability, active transport, and prevention of oxidative damage, and they generate this energy entirely through glycolysis (also known as the Embden-Meyerhof pathway). Erythrocyte antioxidant biochemical pathways are discussed in more detail in the section on Defense Mechanisms. Thrombopoiesis differs fundamentally from other forms of hematopoiesis. Platelets (sometimes called thrombocytes) are anucleate cells formed not by maturational division but by shedding of membrane-bound cytoplasmic fragments from precursor cells called megakaryocytes. (note: Birds and reptiles produce thrombocytes in the manner of other blood cells and their thrombocytes and erythrocytes are nucleated.) As the name suggests, megakaryocytes are very large cells, much larger than any other hematopoietic cells (Fig. 13-6). Megakaryocytes arise from a progenitor cell and undergo endomitosis to become polyploid (usually 8N-32N). As they mature, megakaryocytes increase in size and their cytoplasm becomes more abundant. They change in staining pattern (Wright’s stain of aspirates) from intensely basophilic to eosinophilic, and their nuclei change from round to highly lobulated. Megakaryocytes extend cytoplasmic processes into the lumina of the bone marrow venous sinusoids, where they shed platelets into circulation. The lifespan of a platelet in circulation is approximately 6 days in the dog. Fig. 13-6 Megakaryocyte, canine bone marrow aspirate. • Adhesion to exposed subendothelial collagen • Secretion of bioactive granule contents (including calcium, adenosine diphosphate [ADP], fibrinogen, vWF, histamine, serotonin, and others) • Aggregation with other platelets • Eventual fusion with other platelets to form a platelet plug Lymphopoiesis refers to the production of new lymphocytes. T lymphocytes and B lymphocytes are the key effectors of cell-mediated and humoral immunity, respectively. Lymphopoiesis is discussed in more detail in Chapter 5, but a brief overview is provided here. T lymphocytes originate in the bone marrow and migrate to the thymus, where they undergo differentiation, selection, and maturation processes before migrating to the peripheral lymphoid tissue as effector cells. B lymphocyte development occurs in two phases, first in an antigen-independent phase in the bone marrow and ileal Peyer’s patches (the site of B lymphocyte development in ruminants), then in an antigen-dependent phase in peripheral lymphoid tissues (such as spleen, lymph nodes, and mucosa-associated lymphoid tissue [MALT]). Trafficking of lymphocytes occurs under the direction of chemokine (chemoattractant cytokine) signals. Once they have migrated to the peripheral lymphoid tissue, lymphocytes may undergo clonal expansion in response to antigenic stimulation. Physiologic hemostasis involves coordinated processes: formation of a platelet plug at the site of vascular damage (primary hemostasis), development of a cross-linked network of insoluble fibrin that provides further clot stability (secondary hemostasis) and retraction and enzymatic degradation of the clot (tertiary hemostasis). The main components of these processes are summarized in Chapter 2 and Figs. 2-12 through 2-17. Signalment, history, and clinical signs are important in evaluating any patient with a suspected bleeding tendency, but laboratory testing is required to definitively identify specific defects in hemostasis. Routine assays for assessing hemostasis are summarized in Web Appendix 13-1. Blood Vessels: Blood vessels have numerous functions in hemostasis. Endothelial cells produce and metabolize many molecules involved in promoting and regulating hemostasis (see Fig. 2-16). For example, they produce prothrombotic molecules, such as vWF and thromboxane A2; antithrombotic molecules, such as prostacyclin (PGI2), thrombomodulin, and nitric oxide; and fibrinolytic molecules, such as tissue plasminogen activator. When a blood vessel is damaged, a rapid, transient neuromechanical vasoconstriction occurs in response. Collagen underlying the damaged endothelium provides structural support for platelet adhesion and clot formation, and also acts as a key biochemical activator of platelets and the intrinsic pathway of the coagulation system (see next section). Coagulation System: Stopping hemorrhage from a damaged blood vessel ultimately depends on the formation of a stable fibrin clot. This process depends on a number of plasma proteins, or clotting factors, that normally circulate in an inactive proenzyme state. These clotting factors, together with other molecules, including nonenzymatic proteins, calcium, and platelet phospholipids, are known collectively as the coagulation system. Activation of the coagulation system initiates a series of linked enzymatic reactions that result in the production of thrombin. Thrombin converts the soluble plasma protein fibrinogen to fibrin, which in turn is enzymatically cross-linked to form an insoluble clot. The coagulation system is conventionally considered to consist of three interrelated pathways for ease of understanding and interpretation of test results. These pathways—the intrinsic, extrinsic, and common pathways—are summarized in Fig. 2-13. Anticoagulant and fibrinolytic pathways provide critical “checks and balances.” These pathways are initiated simultaneously with activation of the coagulation system. Procoagulant and anticoagulant pathways are regulated at multiple levels and normally maintained in a balance that avoids hypercoagulable or hypocoagulable states. Some key regulators of hemostasis are listed in Web Box 13-1. Counterregulatory mechanisms are critical for the prevention of inappropriate or excessive clotting and the eventual dissolution of the clot (fibrinolysis) after it has formed. Fibrinolysis results from the action of plasmin on fibrin (see Figs. 2-14, 2-16, and 2-17). The inactive proenzyme plasminogen is converted to plasmin by several molecules, including tissue plasminogen activator, factor XII, and thrombin. Note that some of these molecules (e.g., factor XII and thrombin) have both procoagulant and anticoagulant effects. There are also regulators of fibrinolysis, including inhibitors of plasminogen and plasmin. Fibrinolysis results in production of fibrin degradation products (FDPs). D-dimer is a specific type of FDP that results from the degradation of insoluble cross-linked fibrin. Fibrinolytic products (FDPs and D-dimer) in the blood are often measured to detect inappropriate coagulation and are also of clinical importance because they can impair hemostasis by inhibiting fibrin polymerization and platelet function. Platelets: The basics of platelet function are described in the previous section on Thrombopoiesis. Platelets normally circulate in a quiescent state, as flattened disks. Activation of platelets is triggered by soluble agonists, such as thrombin, or insoluble agonists, such as collagen. Platelets express surface receptors for fibrinogen, vWF, collagen, and other ligands. Other key features of platelets include a network of membrane invaginations known as the open canalicular system (not present in cattle), which facilitates the expansion of surface area and release of granule contents in response to activation; specialized endoplasmic reticulum known as the dense tubular system that acts as a reservoir for calcium; and cytoskeletal components involved in mediating shape change, release of granule contents, receptor interactions, and clot retraction. When activated, platelets change to more spherical forms with pseudopodia and participate in a number of related processes at sites of vascular damage: • Adhesion to exposed subendothelial collagen • Secretion of bioactive granule contents (including calcium, adenosine diphosphate [ADP], fibrinogen, vWF, histamine, serotonin, and others) • Aggregation with other platelets • Eventual fusion with other platelets (a process known as viscous metamorphosis) to form a platelet plug Invading cells or microorganism gain access to the bone marrow or blood circulation either hematogenously or by trauma. Trauma may be as obvious as a gaping wound or as subtle as the bite of an insect. Portals of entry for the bone marrow are summarized in Box 13-1. The bone marrow is encased by a protective shell of cortical bone, and blood supply to the marrow provides access to systemic humoral and cellular defenses. Of course, leukocytes themselves function as an essential part of inflammation and immune function, as discussed briefly in the Granulopoiesis and Monocytopoiesis section and in greater detail in Chapters 3 and 5. The main mechanisms of disease in the bone marrow and blood cells are shown in Box 13-2, and are discussed in more detail here. Bone marrow responses to injury include abnormal patterns of proliferation of normal hematopoietic cell types, proliferations of abnormal hematopoietic cell types (neoplastic or nonneoplastic), replacement of normal hematopoietic tissue by abnormal cells or fibrous tissue, necrosis, and inflammation. Each of these is discussed further; the relationship between altered hematopoiesis and concentrations of blood cells in circulation is discussed in more detail in the next section on Blood Cells. Hypertrophy, an increase in cell size, is not a conventional description for hematopoietic cells or for bone marrow as an organ. Abnormally large hematopoietic cells are considered evidence of dysplasia, or altered cell formation (alterations to shape or organization of cells are also evidence of dysplasia). Dysplasia of hematopoietic cells, indicating dyshematopoiesis, may be primary (idiopathic) or secondary to a number of conditions, including infection, nutritional imbalance, and toxicosis. Unfortunately, the terminology is sometimes confusing. For example, myelodysplastic syndromes are clonal hematopoietic stem cell disorders that are in fact neoplastic (see the section on Hematopoietic Neoplasia). Splenic hypertrophy is usually referred to as splenomegaly and may reflect processes other than hyperplasia or cellular hypertrophy (e.g., congestion). Similarly, lymph node enlargement is typically referred to as lymphadenopathy or lymphadenomegaly and may reflect lymphoid hyperplasia or other processes (e.g., neoplasia, inflammation, or edema). Replacement of hematopoietic tissue in the bone marrow by abnormal tissue, usually fibrous tissue or malignant cells, is known as myelophthisis. The term metaplasia, describing the replacement of cells normally comprising a tissue with another well-differentiated cell type, is rarely used to describe hematopoietic tissues in veterinary medicine, although in humans, the terms myeloid metaplasia and extramedullary hematopoiesis are sometimes used interchangeably. Similarly, the term atrophy, which means a diminution in the size of a cell, tissue, organ, or part, is seldom used to describe bone marrow. Instead, a decrease in bone marrow hematopoietic tissue is typically referred to as hypoplasia, as discussed later in the section on cytopenias. The absence of bone marrow hematopoietic tissue of a particular lineage is typically referred to as aplasia or, if affecting all lineages, by the misleading term aplastic anemia (more aptly termed aplastic pancytopenia). An exception is “serous atrophy of fat,” a condition associated with cachexia or starvation in which fat is catabolized and bone marrow reticular cells produce a mucoid ground substance (Web Fig. 13-1). However, neither hematopoietic cells nor fat is necessarily absent from the bone marrow in this condition, and other names, such as gelatinous transformation, have been suggested to describe it. Neoplasia of bone marrow or lymphoid organs may be primary or secondary (metastatic). Neoplasia may arise from any hematopoietic lineage. Specific types of hematopoietic neoplasia (leukemias, lymphomas, and others) are covered later in this chapter. Web Fig. 13-1 Serous atrophy of fat (gelatinous transformation), bone marrow, calf. Abnormal Concentrations of Blood Cells: Cytopenia—from kytos (Gr., hollow vessel) and penia (Gr., poverty)—refers to a deficiency of blood cells in circulation, hence the terms neutropenia, thrombocytopenia, lymphopenia, and so on. Basic mechanisms causing cytopenias include decreased production (hypoplasia), increased destruction, blood loss, consumption, and altered anatomic distribution (e.g., shifts between marginated and circulating compartments in the blood, or between the spleen and the peripheral blood circulation). Most of these basic mechanisms may cause cytopenias of more than one lineage, and some processes affect multiple lineages simultaneously. Sustained decreased production of all three major bone marrow hematopoietic lineages results in pancytopenia (anemia, neutropenia, and thrombocytopenia). Pancytopenia may occur because of myelophthisis, in which normal bone marrow tissue is replaced by abnormal cells or tissue (as in the case of effacement by malignant cells or fibrous tissue) or because of an abnormality of hematopoietic cells themselves. Destruction of hematopoietic stem cells and progenitor cells causes a condition known as aplastic anemia, or aplastic pancytopenia, which is discussed further in the section on specific diseases. Anemia: Anemia refers to subnormal red blood cell mass or hemoglobin concentration. Anemia causes clinical signs referable to decreased oxygen-carrying capacity (pallor of mucous membranes, lethargy, weakness, exercise intolerance) and may also result in detectable abnormalities because of tissue hypoxia (e.g., increased liver enzyme activities as a result of hypoxia-induced damage to hepatocytes). Anemia also causes decreased viscosity of the blood, and in marked cases frequently causes heart murmurs as a result of a decrease in laminar blood flow. Classifying anemia as regenerative or nonregenerative is clinically useful because it provides information about the mechanism of disease (Table 13-1). The hallmark of regenerative anemias, except in horses, is reticulocytosis (i.e., increased numbers of circulating reticulocytes [immature erythrocytes]), which is evident as polychromasia on a routinely stained blood smear. In ruminants, reticulocytosis is often accompanied by basophilic stippling (Fig. 13-7). TABLE 13-1 Fig. 13-7 Basophilic stippling and polychromasia, bovine blood smear. Reticulocytosis indicates increased bone marrow erythropoiesis (Fig. 13-8) and release of erythrocytes before they are fully mature (further back in the production “pipeline”). Reticulocytosis is an appropriate response to anemia. A strong regenerative response may produce an increased mean cell volume (MCV) and decreased mean cell hemoglobin concentration (MCHC) on the complete blood count (CBC), because reticulocytes are larger and have a lower hemoglobin concentration than mature erythrocytes. Horses are an exception to this classification scheme because they do not release reticulocytes into circulation even when their marrow is producing increased numbers of erythrocytes. Horses with a regenerative response may have an increased MCV and red cell distribution width (an index of variation in cell size). But definitive determination of regeneration in a horse requires either bone marrow examination, in which case the evidence of regeneration is erythroid hyperplasia, or sequential CBCs, in which case the evidence of regeneration is an increase in red cell mass over time. Punctate reticulocytes in cats are evident when blood is stained with new methylene blue, but they are not counted as reticulocytes on the CBC and have the same appearance as mature erythrocytes on routine blood smear examination. Fig. 13-8 Hemopoietically active bone marrow, femur, calf. • Exposure of membrane components normally sequestered on the inner leaflet of the cell membrane, particularly phosphatidylserine (this mechanism is also important in apoptosis of other cell types). • Binding of IgG and/or complement. In hemolytic anemia, erythrocytes are destroyed at an increased rate. Whether the mechanism is intravascular or extravascular, or a combination, depends on the specific disease process (specific diseases are discussed later in this chapter). A classic sequela of hemolytic anemias in general is hyperbilirubinemia, which is an increase in the plasma concentration of bilirubin. Bilirubin is a yellow pigment, which explains why hyperbilirubinemia, if severe enough, causes icterus—the grossly visible yellowing of serum or tissue (Fig. 13-9). Icterus of mucous membranes, skin, and other tissue is known as jaundice. Icterus is usually detectable when the plasma bilirubin concentration exceeds 2 mg/dL. Hemolysis is a cause of prehepatic hyperbilirubinemia, but it is important to note that hyperbilirubinemia can also occur as the result of hepatic or posthepatic conditions causing impaired bile flow (cholestasis). Fig. 13-9 Icterus, immune-mediated hemolytic anemia (IMHA), subcutaneous fat, splenomegaly, spleen, dog. Laboratory findings and clinical observations may point to a specific mechanism of hemolysis. In patients with extravascular hemolytic anemia, increased destruction of erythrocytes by splenic macrophages often results in splenomegaly (Fig. 13-10). Splenomegaly may also occur because of other conditions, as discussed elsewhere in this chapter. Intravascular hemolysis is grossly evident as hemoglobinemia (pink-tinged plasma or serum), if the concentration of extracellular hemoglobin is greater than approximately 50 mg/dL. Haptoglobin is saturated with dimeric hemoglobin at a concentration of approximately 150 mg/dL. When haptoglobin is saturated, any remaining free hemoglobin has a low enough molecular weight to pass through the renal glomerular filter into the urine (hemoglobinuria), imparting a pink or red discoloration to the urine. Thus extracellular hemoglobin can cause gross discoloration of the plasma, where it is bound to haptoglobin, before becoming grossly visible in urine. The half-life of haptoglobin is markedly decreased when bound to hemoglobin, so when large amounts of haptoglobin-hemoglobin complex are formed, the concentration of haptoglobin in the blood decreases and hemoglobin can pass through the glomerulus at even lower concentrations. Hemoglobinuria is a contributing factor in the renal tubular necrosis (hemoglobinuric nephrosis) that often occurs in cases of acute intravascular hemolysis (see Chapter 11). A similar lesion occurs in the kidneys of individuals with marked muscle damage and resulting myoglobinuria. Fig. 13-10 Splenomegaly, fatal hemolytic anemia, Mycoplasma suis, pig. Extravascular hemolysis also often produces characteristic CBC abnormalities that may reflect the mechanism of disease. For example, spherocytosis and autoagglutination are hallmarks of IMHA. Spherocytes form when macrophages (mainly in the spleen) phagocytose part of an erythrocyte plasma membrane bound with autoantibody (Fig. 13-11). The remaining portion of the RBC assumes a spherical shape, thus preserving maximal volume. This change in shape results in decreased deformability of the cells. Erythrocytes must be extremely pliable to traverse the splenic red pulp and sinusoidal walls (Fig. 13-12); spherocytes therefore tend to be retained in the spleen in close association with macrophages with risk of further injury and eventual destruction. In the dog, spherocytes appear smaller than normal and have uniform staining (Fig. 13-13, A), in contrast to normal RBCs, which have a region of central pallor imparted by their biconcave shape. This difference in staining between spherocytes and normal RBCs is not consistently discernible in many other domestic animals (including horses, cattle, and cats), whose erythrocytes differ from those of the dog in that they are smaller and have less pronounced biconcavity and therefore less pronounced central pallor. Autoagglutination occurs because of cross-linking of antibodies bound to erythrocytes. Autoagglutination is evident microscopically as clusters of erythrocytes, and macroscopically as blood with a grainy consistency (Fig. 13-13, B). Autoagglutination may also result in a falsely increased MCV and decreased RBC concentration when clustered cells are mistakenly counted as single cells by automated hematology analyzers. Fig. 13-11 Schematic representation of the mechanisms of spherocytosis and extravascular hemolysis (EVH). Fig. 13-12 Schematic representation of a splenic sinusoid. Fig. 13-13 Immune-mediated hemolytic anemia (IMHA), canine blood, dog. Oxidative damage to erythrocytes occurs when normal antioxidative pathways that generate reducing agents (such as NADH, NADPH, and reduced glutathione) are compromised or overwhelmed and can result in hemolytic anemia, abnormal hemoglobin function, or both. Hemolysis caused by oxidative damage may be extravascular or intravascular, or a combination (predominant forms of hemolysis are discussed further in the section on specific diseases). Evidence of oxidative damage to erythrocytes may be apparent on examination of a blood smear, or even on gross physical examination. Heinz bodies are foci of denatured globin that interact with the erythrocyte membrane. They are usually subtly evident on routine Wright’s-stained blood smears as pale circular inclusions or blunt, rounded protrusions of the cell margin and are readily discernible on smears stained with new methylene blue (Fig. 13-14). Cats are particularly susceptible to Heinz body formation and may have low numbers of small Heinz bodies normally. There is no unanimity of opinion, but some clinical pathologists believe that the presence of Heinz bodies in up to 10% of all RBCs in cats is within normal limits. This predisposition is believed to reflect unique features of the feline erythrocyte, whose hemoglobin has more sulfhydryl groups (preferential sites for oxidative damage) than do erythrocytes of other species and may also have lower intrinsic reducing capacity. It is also possible that the feline spleen, which is nonsinusoidal, does not have as efficient a “pitting” function as does a sinusoidal spleen, such as that of the dog (splenic structure and function are discussed in more detail later in this chapter). Eccentrocytes, evident as erythrocytes in which one side of the cell has increased pallor, are another manifestation of oxidative damage. They form because of cross-linking of membrane proteins, with adhesion of opposing areas of the cell’s inner membrane leaflet, and displacement of most of the hemoglobin toward the other side (Fig. 13-15). Oxidative insult may also result in conversion of hemoglobin (iron in the Fe2+ state) to methemoglobin (iron in the Fe3+ state), which is incapable of binding oxygen. Methemoglobin is produced normally in small amounts but reduced back to oxyhemoglobin by the enzyme cytochrome-b5 reductase (also known as methemoglobin reductase). Methemoglobinemia results when methemoglobin is produced in excessive amounts (because of oxidative insult) or when the normal pathways for maintaining hemoglobin in the Fe2+ state are impaired (as in cytochrome-b5 reductase deficiency). When present in sufficiently high concentration (approximately 10% of total hemoglobin), methemoglobin imparts a grossly discernible chocolate color to the blood. Fig. 13-14 Heinz bodies, blood smears. Fig. 13-15 Common erythrocyte morphologic abnormalities. Nonregenerative anemias are characterized by a lack of reticulocytosis on the CBC; however, reticulocytosis does not occur in horses even in the context of regeneration. Most often this is a result of decreased production in the marrow (i.e., erythroid hypoplasia). Erythrocytes circulate for a long time, so anemias caused by decreased production tend to develop slowly. The most common form of nonregenerative anemia is known as anemia of inflammation or anemia of chronic disease. In this form of anemia, RBCs are decreased in number but are typically normal in size and hemoglobin concentration (so-called normocytic, normochromic, anemia). It has long been known that patients with inflammatory or other chronic disease often become anemic and that this condition is associated with increased iron stores in the bone marrow. Sequestration of iron may be a bacteriostatic evolutionary adaptation because many bacteria require iron as a cofactor for growth. In recent years, investigators have begun to elucidate the molecular mechanisms underlying anemia of inflammation. Hepcidin, an acute phase protein synthesized in the liver that was first identified as an antimicrobial peptide, is a key mediator that acts by limiting iron availability. Hepcidin expression increases with inflammation, infection, or iron overload and decreases with anemia or hypoxia. Hepcidin exerts its effects by causing functional iron deficiency. It binds to and causes the degradation of the cell surface iron efflux molecule, ferroportin, thus inhibiting both absorption of dietary iron from the intestinal epithelium and export of iron from macrophages and hepatocytes into the plasma (Fig. 13-16). Fig. 13-16 Mechanism of action of hepcidin. Other causes of decreased erythropoiesis include the following: • Decreased hormonal stimulation (e.g., chronic renal failure) • Infection of erythropoietic cells • Toxic insult to the bone marrow • Other disease involving the bone marrow • Immune-mediated destruction of erythroid precursors Neutropenia: Neutropenia refers to a decrease in the concentration of neutrophils in circulating blood. Neutropenia may be caused by decreased production, increased destruction, altered distribution, demand for neutrophils in inflamed tissue that exceeds the rate of granulopoiesis, or inherited disease. Decreased production is evident on bone marrow examination as granulocytic hypoplasia. This usually results from an insult that affects multiple hematopoietic lineages, such as chemical insult, radiation, neoplasia, infection, and fibrosis, but may also be caused by a process that preferentially targets granulopoiesis. Immune-mediated neutropenia is a rare but recognized condition in domestic animals. Bone marrow findings range from granulocytic aplasia or hypoplasia to hyperplasia, depending on where the cells under immune attack are in their differentiation programs. Neutropenia with no evidence of decreased production and in which other causes of neutropenia have been excluded may be a result of destruction of neutrophils before they leave the bone marrow, a condition known as ineffective granulopoiesis. Like other forms of ineffective hematopoiesis, this condition is often presumed to be immune mediated; in cats this condition may occur as a result of infection of hematopoietic cells with the feline leukemia virus (FeLV). Eosinopenia/Basopenia: In many laboratories, CBC reference values for eosinophils and basophils are as low as zero cells per microliter, precluding detection of eosinopenia or basopenia. When detectable, eosinopenia is often part of a stress (glucocorticoid-mediated) leukogram. Thrombocytopenia: Thrombocytopenia refers to a decrease in the concentration of circulating platelets. Mechanisms of thrombocytopenia include decreased production, increased destruction, increased consumption, altered distribution, and hemorrhage. Decreased production may occur because of a condition affecting cells of multiple hematopoietic lineages, including platelets, or to one specifically depressing thrombopoiesis. In either case, decreased thrombopoiesis is evident on examination of a bone marrow sample as megakaryocytic hypoplasia. General causes of decreased hematopoiesis outlined earlier in the sections on anemia and neutropenia also apply to thrombocytopenia. Specific diseases causing thrombocytopenia are covered later in this chapter. Immune-mediated thrombocytopenia is a fairly common disease in dogs and may also occur in other species. Increased consumption of platelets is a hallmark of disseminated intravascular coagulation (DIC), a syndrome in which hypercoagulability leads to increased consumption of both platelets and coagulation factors in the plasma, with subsequent hypocoagulability and susceptibility to bleeding. The spleen normally contains a significant proportion of total platelet mass (up to one-third in some species), and abnormalities involving the spleen may result in changes in the number of circulating platelets. For example, splenic congestion may result in thrombocytopenia, and splenic contraction may result in thrombocytosis. Acute hemorrhage may result in mild to moderate thrombocytopenia. Potential mechanisms of thrombocytopenia caused by hemorrhage include loss and consumption. Of course, thrombocytopenia can also be a cause of hemorrhage. In the absence of other complicating factors, marked to severe thrombocytopenia (less than 50,000 platelets/µL) is more likely to be the cause of rather than the result of bleeding. Megakaryocytic hyperplasia on examination of a bone marrow sample is evidence of a regenerative thrombopoietic response, and an increase in the mean platelet volume (MPV) value on the CBC often accompanies such a response. Lymphopenia: Lymphopenia refers to a decrease in the concentration of lymphocytes in blood circulation. It is a common CBC finding in sick animals. Usually the precise mechanism of lymphopenia is not clear. It is often presumed to be mediated at least in part by endogenous glucocorticoid excess. Lymphopenia may occur because of various mechanisms, including altered distribution of lymphocytes (increased trafficking of lymphocytes to, and decreased egress from, lymphoid tissues), lymphotoxicity (direct damage to lymphocytes or suppression of lymphopoiesis) of therapeutic or infectious agents, loss of lymphocyte-rich lymphatic fluid, or congenital disorders. Normal lymphocyte trafficking may be altered because of disruption of the normal architecture of lymphoid tissue (e.g., because of neoplasia or inflammation), or in response to cytokine signals. Glucocorticoid excess may cause lymphopenia via redistribution from the blood to lymphoid tissue, or via direct lymphotoxic effects. Anticancer treatments (chemotherapy or radiotherapy) and immunosuppressive drugs may also be lymphotoxic. Some hereditary immunodeficiencies (such as severe combined immunodeficiency or thymic aplasia) can cause lymphopenia. Erythrocytosis: An increase in the measured red cell mass above the normal range (i.e., the opposite of anemia) is known as erythrocytosis. The term polycythemia is often used interchangeably with erythrocytosis, but technically and for the purposes of this chapter, polycythemia refers to a specific type of leukemia called primary erythrocytosis or polycythemia vera. Relative erythrocytosis occurs most frequently because of dehydration, when the decreased proportion of water in the blood results in hemoconcentration. A similar situation may occur as a result of epinephrine-mediated splenic contraction. Erythrocytosis caused by splenic contraction occurs to the most pronounced degree in the horse and may occur to a lesser extent in other species. In both of these cases, the total RBC mass is not in fact increased but appears to be so because of other factors. Secondary erythrocytosis refers to a true, Epo-mediated increase in red cell mass, either as an appropriate response to hypoxemia (such as may be seen in patients with cardiopulmonary disease and severely impaired oxygenation, or cardiovascular defects causing right-to-left shunting) or in rare cases an Epo-secreting tumor (inappropriate secondary erythrocytosis). Polycythemia vera is diagnosed based on a marked increase in red cell mass (hematocrit in normally hydrated dogs ranges from 65% to >80%), absence of hypoxemia, absence of other tumors, and normal or decreased concentration of plasma erythropoietin concentration. Absolute erythrocytosis, whether primary or secondary, causes increased viscosity of the blood and resulting impairment of blood flow and distention of the microvasculature. Affected individuals are at increased risk of tissue hypoxia and thrombosis or hemorrhage. Related clinical signs (hyperviscosity syndrome) may include erythematous mucous membranes (Fig. 13-17) and prolonged capillary refill time, prominent scleral vessels, evidence of thrombosis or hemorrhage, and secondary signs (e.g., neurologic and cardiovascular) related to specific organ systems affected. Fig. 13-17 Absolute erythrocytosis, hyperviscosity syndrome, erythematous mucous membranes, cat. Neutrophilia: Neutrophilia occurs in response to a number of different stimuli, which are not mutually exclusive. Major mechanisms of neutrophilia are shown in Fig. 13-18. Understanding the CBC findings characteristic of these responses is an important part of clinical veterinary medicine. Inflammation can result in neutropenia, as discussed earlier, or neutrophilia, as discussed next. However, before moving on to a discussion of inflammatory neutrophilia and the so-called left shift, it is important to mention two other common causes of neutrophilia. Fig. 13-18 Schematic illustration of the mechanisms of neutrophilic leukocytosis. • Increased release of mature neutrophils from the bone marrow storage pool. • Decreased margination of neutrophils within the vasculature, with a resulting increase in the circulating pool. • Decreased migration of neutrophils from the bloodstream into tissues.
Bone Marrow, Blood Cells, and the Lymphatic System*
Bone Marrow and Blood Cells
The bone marrow consists of (1) stem cells, pluripotent cells capable of self-renewal; (2) committed progenitor cells (myeloid and lymphoid progenitor cells); and (3) maturing cells. Maturing cells develop from cells called colony-forming units (CFUs). The myeloid stem cell gives rise to CFUs responsible for the regeneration of red blood cells (erythroid CFUs), platelets (megakaryocyte CFUs), basophils (basophil CFUs), and eosinophils (eosinophil CFUs). Monocytes and neutrophils are derived from a common committed progenitor cell (granulocyte-macrophage CFU). The lymphoid progenitor cell generates the B lymphocyte progeny in the bone marrow and T lymphocyte progeny in the thymus. (Adapted from Kierszenbaum AL: Histology and cell biology: an introduction to pathology, St Louis, 2002, Mosby.)
Wright’s stain. ESE, Early stage erythroid; ESM, early stage myeloid; LSE, late stage erythroid; LSM, late stage myeloid. (Courtesy Dr. M.M. Fry, College of Veterinary Medicine, University of Tennessee.)
Erythropoiesis
A, Reticulocytes (arrows) appear polychromatophilic with routine staining. Wright’s stain. B, Reticulocytes. Precipitated aggregates of RNA are stained blue (arrows) with new methylene blue. (Courtesy Dr. M.M. Fry, College of Veterinary Medicine, University of Tennessee.)
Thrombopoiesis
Note the cell’s very large size, lobulated nucleus, and abundant granular cytoplasm. Wright’s stain. (Courtesy Dr. M.M. Fry, College of Veterinary Medicine, University of Tennessee.)
Lymphopoiesis
Hemostasis (Coagulation And Platelet Function)
Portals of Entry
Defense Mechanisms
Responses to Injury
Bone Marrow
Serous atrophy of fat is caused by starvation and/or cachexia and is characterized by replacement of normal fat and hematopoietic elements by a gelatinous extracellular matrix. (Courtesy Department of Pathobiology, College of Veterinary Medicine, University of Tennessee).
Blood Cells
Erythrocytes from this cow with regenerative anemia include several cells with basophilic stippling (arrow) and two polychromatophilic cells (reticulocytes) (arrowheads). Wright’s stain. (Courtesy Dr. M.M. Fry, College of Veterinary Medicine, University of Tennessee.)
Note that the bone marrow has a uniform consistency and is red to dark red. These responses are characteristic of hemopoietically active bone marrow. (Courtesy Dr. Ramos, Autonomous University of Barcelona; and Noah’s Arkive, College of Veterinary Medicine, University of Georgia.)
The marked yellow discoloration of tissues, most strikingly visible in the subcutaneous fat, is from high concentrations of serum bilirubin produced as a result of the hemolytic anemia. (Courtesy Dr. J.A. Ramos-Vara, College of Veterinary Medicine, Michigan State University; and Noah’s Arkive, College of Veterinary Medicine, The University of Georgia.)
The spleen is extremely enlarged, meaty, and congested. (Courtesy College of Veterinary Medicine, University of Illinois.)
Hereditary spherocytosis occurs in humans (as shown here) because of mutations that weaken the connections between cytoskeletal and membrane proteins. In cases of immune-mediated hemolytic anemia (IMHA), the most frequent cause of spherocytes in animals, the underlying disease mechanism is different—spherocytes form when portions of the erythrocyte membrane bound with autoantibody are phagocytosed by macrophages—but the net result (spherocytosis and EVH) is similar to what is shown here. GP, Glycoprotein. (From Kumar V, Abbas AK, Fausto N, et al: Robbins & Cotran pathologic basis of disease, ed 8, Philadelphia, 2009, Saunders.)
A red blood cell (RBC) is in the process of squeezing from the red pulp cords into the sinus lumen. Note the degree of deformability required for RBCs to pass through the wall of the sinus. (From Kumar V, Abbas AK, Fausto N: Robbins & Cotran pathologic basis of disease, ed 7, Philadelphia, 2005, Saunders.)
A, Spherocytosis. Numerous spherocytes (arrows) and several polychromatophilic cells, one of which (arrowhead) contains a nuclear remnant known as a Howell-Jolly body, are visible. Wright’s stain. B, Autoagglutination. Note the grossly visible agglutination. C, Spherocytosis and agglutination. Note the spherocytes (arrows) and microscopic agglutination of erythrocytes in a dog that received a blood transfusion. These abnormalities presumably indicate immune-mediated destruction of the donor erythrocytes. Wright’s stain. (A courtesy Dr. M.M. Fry, College of Veterinary Medicine, University of Tennessee. B and C from Harvey JW: Atlas of veterinary hematology: blood and bone marrow of domestic animals, Philadelphia, 2001, Saunders.)
A, Feline blood smear. With routine staining, Heinz bodies appear as pale circular intraerythrocytic inclusions that may protrude (arrows) from the margin of the cell. Wright’s stain. B, Canine blood smear. Using a supravital stain, Heinz bodies are blue inclusions (arrows) and easier to see. New methylene blue stain. (Courtesy Dr. M.M. Fry, College of Veterinary Medicine, University of Tennessee.)
A, Blood from a dog with a microcytic hypochromic iron-deficiency anemia was mixed with an equal volume of blood from a normal dog before blood smear was prepared. Because the hypochromic (pale-staining) cells are leptocytes shown in F, they have diameters similar to normal cells even though they are microcytic cells. Wright-Giemsa stain. B, Echinocytes appear as erythrocytes with scalloped borders; consequently, the old term “crenation” from Latin meaning “notched” is used. Wright-Giemsa stain. C, Three acanthocytes with irregularly spaced, variably sized spicules in blood from a dog with hemangiosarcoma. Wright-Giemsa stain. D, A keratocyte, exhibiting what appears to be a ruptured “vesicle” in blood from a cat with hepatic lipidosis. Wright-Giemsa stain. E, A schistocyte (left), discocyte (top), and echinocyte (bottom) in blood from a dog with disseminated intravascular hemolysis. Wright-Giemsa stain. F, Two thin flat hypochromic-appearing erythrocytes (leptocytes), with increased membrane-to-volume ratios, are present in blood from a dog with severe iron-deficiency anemia. The bottom leptocyte is folded. Wright-Giemsa stain. G, Three codocytes in blood from a Cairn terrier with a regenerative anemia and hepatic hemochromatosis secondary to pyruvate kinase deficiency. These erythrocytes exhibit a central density or “bull’s-eye” and are often referred to as target cells. Wright-Giemsa stain. H, Three eccentrocytes and a discocyte (left) in blood from a dog with oxidant injury induced by the administration of acetaminophen. The cell at top center appears spherical with a small tag of cytoplasm and may be referred to as a pyknocyte. Wright-Giemsa stain. (From Harvey JW: Atlas of veterinary hematology: blood and bone marrow of domestic animals, Philadelphia, 2001, Saunders.)
Binding of hepcidin to ferroportin causes its internalization and degradation, thus inhibiting efflux of iron into the plasma. (Courtesy Dr. J.F. Zachary, College of Veterinary Medicine, University of Illinois.)
Erythema of mucous membranes is one of the signs associated with hyperviscosity syndrome. In this case, the oral mucous membranes are deeper red (arrows) than normal because of an abnormally high concentration of erythrocytes and associated sludging of blood. Hyperviscosity syndrome may also occur as the result of increased plasma immunoglobulin concentration. (Courtesy Dr. C. Patrick Ryan, Veterinary Public Health, Los Angeles Department of Health Services; and Noah’s Arkive, College of Veterinary Medicine, University of Georgia.)
Neutrophils and their precursors are distributed in five pools: a bone marrow precursor pool, which includes progenitor cells and more committed, mitotically active precursors; a bone marrow storage pool, consisting of mitotically inactive mature and slightly immature neutrophils (band forms); a peripheral blood marginating pool (Marg. pool); a peripheral blood circulating pool (Circ. pool); and a tissue pool. The relative size of each pool is represented by the size of its corresponding box. The peripheral blood neutrophil count measures only the circulating peripheral blood pool, which can be enlarged by increased release of cells from the marrow storage pool, increased demargination, diminished extravasation into tissue, or expansion of the marrow precursor cell pool. (Modified from Finch SC: Hematology, ed 3, New York, 1983, McGraw-Hill.)
Stay updated, free articles. Join our Telegram channel
Full access? Get Clinical Tree
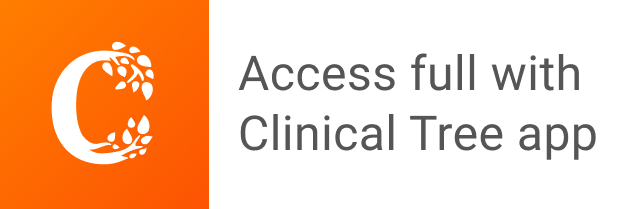