Chapter 13 Hepatic Function III. CLINICAL MANIFESTATIONS OF HEPATIC INSUFFICIENCY IV. LABORATORY ASSESSMENT OF HEPATIC FUNCTION The liver has an essential role in nutrient metabolism including the control and maintenance of the blood glucose level; in detoxification and excretion of hydrophobic metabolites and xenobiotics; in the synthesis of most plasma proteins; and in digestion through synthesis, biliary secretion, and conservation of bile acids that are essential both for digestion and intestinal absorption of fats and other lipids including fat soluble vitamins. The clinical manifestations of hepatic disease are directly attributable to alterations in the metabolic, excretory, synthetic, and digestive functions of the liver. The liver has great reserve, and signs of hepatic failure often do not develop until 70% or more of functional capacity is lost. Importantly, even when a major fraction of the hepatocellular mass has been lost following acute injury, recovery is possible because of the unique regenerative capacity of the liver. Since Dr. Charles E. Cornelius wrote this chapter for earlier editions of this textbook (Cornelius, 1970), remarkable advances have been made in our understanding of the pathophysiological, biochemical, and molecular mechanisms responsible for hepatic disease. Our current knowledge of veterinary hepatology is the result of the collective work of individuals from a variety of disciplines, including practicing veterinarians, biomedical scientists, and, more recently, molecular and cell biologists and molecular geneticists. No one individual had a more important or sustained impact than Dr. Cornelius, and no one was more active in maintaining a comparative perspective on the subject (Cornelius, 1993). In this chapter, we describe the biochemical mechanisms responsible for the cardinal clinical manifestations of hepatic insufficiency and the biochemical tests used in the clinical diagnosis of liver disease and to assess hepatic function. As in previous editions, the goal is to provide students of veterinary medicine at all stages of career development with information useful for managing the diseases of animal patients. During embryological development, the liver arises as an outgrowth of the primitive gut and is located cranial to the abdominal viscera and other abdominal organs between the splanchnic and the peripheral (systemic) circulatory systems. Unlike other mammalian organs, afferent blood to the liver is derived from two sources, the hepatic artery and the hepatic portal vein. Efferent blood leaves the liver by the hepatic vein and enters the systemic circulation via the caudal vena cava. Twenty to 30% of afferent blood comes from the hepatic artery, and the remainder from the hepatic portal vein, which drains the pancreas, spleen, stomach, small intestine, and all but the most terminal portion of the large intestine. The peripheral border of the classical liver lobule is formed by the most peripheral row of hepatocytes (the terminal plate) and by two, three, or more portal tracks (portal triads) that contain preterminal branches of the hepatic artery, the hepatic portal vein, and bile ductules. Lymphatic vessels also are present in the portal tracts, but because of their small size and thin walls they are difficult to recognize morphologically. Although the classical lobular architecture of the liver remains in use for the morphological description of pathological lesions of the liver, most analyses indicate that the functional unit of the liver is the hepatic acinus in which blood flows from terminal hepatic artery and terminal portal vein of the portal tracts toward two, three, or more terminal collecting veins (central veins). Significant structural and functional heterogeneity has been demonstrated between hepatocytes of the periphery of the hepatic acinus (zone 1), the midzonal hepatocytes (zone 2), and perivenous hepatocytes (zone 3) (Jungermann and Katz, 1989). The functional significance of some of these differences will be discussed in this chapter. Hepatocytes are the principal cell type of the liver and make up approximately 70% of the total volume. Kupffer cells are the macrophages of the liver located in the sinusoids with pseudopodia that are attached to endothelial cells and hepatocytes. The Kupffer cells and other perisinusoidal cells are responsible for local inflammatory and other immune responses. The stellate cells or Ito cells are found in the space of Disse where they are identified are the vitamin A-containing vacuoles in the cytoplasm. When activated by Kupffer cells, the stellate cells transform into myofibroblasts and are responsible for the production of collagen in liver diseases that are associated with fibrosis or cirrhosis. The hepatocytes are arranged in single-cell plates separated by sinusoids lined by vascular endothelial cells. Blood from the terminal branches of the hepatic artery and the hepatic portal vein enters and mixes in the periphery of the liver acinus and percolates through the sinusoids to the terminal hepatic vein. The vascular endothelium lining the hepatic sinusoids differs from that of other capillaries in two ways. Under normal conditions, hepatocytes do not rest on a basement membrane but are separated from endothelial cells by the perisinusoidal space of Disse. Second, dynamic fenestrations of the sinusoidal endothelium are responsible for formation of hepatic lymph that has a protein content much higher than that of the lymph formed in other tissues, which is an ultrafiltrate of plasma with a characteristically low protein content. Blood exits the liver through branches of the hepatic vein and obstruction of hepatic vein outflow increases the formation of hepatic lymph that is rich in protein. This may occur in congestive heart failure, in mechanical obstruction of hepatic vein outflow (Budd-Chisari syndrome), and in the early stages of hepatic fibrosis. In advanced hepatic cirrhosis, dense intracellular matrix forms in the space of Disse. “Capillarization” of the sinusoids develops reducing the fenestrations of the sinusoidal endothelium, and in formation of hepatic lymph that is typically low in protein, closely resembling the lymph produced by other normal tissues (see Section III.D). Bile is secreted via the microvillous membrane of bile canaliculus located in the apical cell membrane of the hepatocyte and flows in the direction of the portal tracts in channels formed by the canaliculi of 2, 3, or more adjacent hepatocytes. These channels converge near the portal tracts forming the canals of Herring through which bile drains into the bile ductules of the portal tracts and finally into the ducts of the biliary tree. Hepatic lymph formed in the space of Disse flows in a similar direction and exits the liver via lymphatics vessels located in the portal tracts. Hepatic lymph leaves the liver primarily via the hilar lymphatic, hilar lymph nodes, and the thoracic duct. Hepatic lymph also leaves the liver by lymph vessels associated with the hepatic vein. Cells of the periportal Zone 1 of the acinus are more likely to divide than other hepatocytes (Grisham, 1959). Mitochondria are larger and more numerous in Zone 1 hepatocytes than are those of Zone 3 (Loud, 1968; Uchiyama and Asari, 1984). Fenestrae of Zone 1 sinusoidal endothelial cells are larger than those of the Zone 3 region, and this may account for selective uptake of large, more complex molecules such as remnants of chylomicrons by Zone 1 hepatocytes (Wisse et al., 1985). A significant oxygen gradient has been demonstrated between sinusoids of Zones 1 and 3. The concentrations of glucose and amino acids that arrive primarily from the hepatic portal vein are higher in zone 1 sinusoids during digestion. Such metabolic differences are associated with important functional differences between Zones 1 and 3. The enzymes of glycolysis, gluconeogenesis, and glycogen metabolism have different activities within zones. Glucose-6-phosphatase, phosphoenolpyruvate carboxykinase, and fructose-1,6-diphosphatase activities are higher in periportal hepatocytes, whereas glucokinase and pyruvate kinase activities are higher in pericentral hepatocytes (Zakim, 1996). Glycogen appears to be uniformly distributed within the cells of the acinus during steady-state conditions, but during fasting, glycogen of periportal hepatocytes is utilized more rapidly and, during feeding, is replaced more rapidly. Two plasma membrane transporters for glucose are expressed in the liver. Glut-2 is the primary glucose transporter of the liver and is expressed in plasma membranes of all hepatocytes. The Km of Glut-2 for glucose is 15 to 20mM, a concentration of glucose that can be reached or exceeded in the hepatic portal vein during and immediately after feeding. Under these conditions, glucose is transported into hepatocytes for the synthesis of glycogen, amino acids, and triglycerides. Between meals, the glucose concentration in the portal vein is approximately 5 mM and is similar to that in the peripheral circulation. During the interdigestive period, the concentration of glucose in hepatocytes is high relative to that of sinusoidal blood. Glut-2 also facilitates transport of glucose from the cytoplasm of hepatocytes into the sinusoid. Glucose reaching the systemic circulation, maintains the peripheral blood glucose concentration, and contribute to meeting systemic energy requirements. The Glut-1 transporter is present only in the plasma membranes of Zone 3 (Tal et al., 1990). The affinity of Glut-1 for glucose is much higher (Km 1–2 mM) than that of Glut-2. The Glut-1 gene is transcribed and translated by all hepatocytes of the acinus; however, by means of a post-translational control mechanism, Glut-1 is inserted into the plasma membrane of only pericentral hepatocytes (Bilir et al., 1993). The liver plays a critical role in the removal of ammonia from the blood. Two separate reactions within the liver acinus are involved. The concentration of ammonia in the Zone 1 sinusoids is high compared to that of the Zone 3 sinusoids. Most of the ammonia that enters the liver diffuses into the hepatocytes of Zones 1 and 2, and a relatively small amount of ammonia reaches the hepatocytes of Zone 3. Hepatocytes of Zones 1 and 2 contain carbamoyl phosphate synthase and other enzymes of the urea cycle that are responsible for conversion of ammonia to urea. The activity of glutamine synthase is confined to the hepatocytes located adjacent to the terminal hepatic vein, and in these perivenous hepatocytes, glutamate synthase is responsible for the use of ammonia in the catalytic amination of glutamate forming glutamine. The Km of carbamoyl phosphate synthase for ammonia is approximately 1.2 mM, whereas that of glutamine synthase for ammonia is 0.3 mM (Gumucio and Berkowitz, 1992; Gumucio et al., 1994). Synthesis of urea from ammonia in periportal hepatocytes and of glutamine from ammonia in pericentral hepatocytes represents complementary enzymatic processes. Ammonia is first seen by hepatocytes that synthesize urea utilizing a low-affinity, high-capacity system. At the end of passage through the hepatic sinusoid, the small amount of ammonia remaining is removed for glutamine synthesis using a relatively high-affinity, low-capacity mechanism. Zone 1 and 2 hepatocytes are responsible primarily for the bile salt-dependent fraction of bile formation and appear to be the primary site of bile salt synthesis. The enzymes necessary for fatty acid synthesis, CoA carboxylase and fatty acid synthase, are located primarily in Zone 3 hepatocytes. Drug-metabolizing enzymes of the cytochrome P-450 family are located predominantly in Zone 2 and 3 hepatocytes, explaining hepatocellular damage and fatty metamorphosis preferentially in Zone 3 hepatocytes (Jungermann and Katz, 1989) after exposure to toxins such as carbon tetrachloride. Bilirubin is a yellow pigment produced by the enzymatic degradation of heme. Approximately 80% of the bilirubin produced normally by mammals is derived from the removal of senescent erythrocytes from the circulation by the reticuloendothelial systems (Landau and Winchell, 1970; Robinson et al., 1966). Degradation of heme from other sources (myoglobin, cytochromes, peroxidase, catalase, guanylate cyclase) accounts for production of the remaining bilirubin. Significant amounts of microsomal cytochromes (P-450, b5) are present in the liver and are the most important non-erythroid source of bilirubin. The initial step in bilirubin formation is the opening of the heme (ferriprotoporphyrin) ring at the α-methene bridge (Fig. 13-1). This reaction is catalyzed by microsomal heme oxygenase (Tenhunen et al., 1968, 1969, 1970a, 1970b). Cytochrome P450 serves as the terminal oxidase and requires reduced nicotinamide adenine dinucleotide phosphate (NADPH) and molecular oxygen. The products of heme degradation are equimolar amounts of iron, biliverdin, and carbon monoxide (Sassa, 2004; Tenhunen et al., 1968, 1969). It has been estimated that more than 86% of endogenously produced carbon monoxide is derived from the enzymatic breakdown of heme (Ryter et al., 2006), and quantitation of respiratory carbon monoxide has been used as an indirect measurement of bilirubin production (Landaw and Winchell, 1970). A minor amount of carbon monoxide arises from other metabolic processes including peroxidation of lipids (Archakov et al., 2002; Vreman et al., 1998). In primary hepatocyte cultures, degradation of hepatic heme also has been shown to use pathways that do not yield carbon monoxide (Bissell and Guzelian, 1980). FIGURE 13-1 Enzymatic degradation of heme (ferriprotoporphyrin) and formation of bilirubin. Heme oxygenase is most active in the tissues that remove erythrocytes and degrade heme. The spleen is the most important in this regard, followed by the liver and the bone marrow (Tenhunen et al., 1968, 1969, 1970a). The kidney normally plays a minor role in heme degradation but in hemolytic disorders associated with hemoglobinuria, the kidney has a quantitatively more important role in heme degradation. When intravascular hemolysis begins, glomerular filtration of hemoglobin is initially prevented by the binding of hemoglobin to the plasma protein haptoglobin. When the haptoglobin binding capacity is exceeded, however, glomerular filtration of hemoglobin occurs. Depending on the amount filtered, some hemoglobin is reabsorbed by renal tubular epithelium. This induces formation of heme oxygenase and production of bilirubin by the kidney (de Schepper and Van Der Stock, 1972a, 1972b; Pimstone et al., 1971). Degradation of hemoglobin in this manner may have a homeostatic function that conserves iron and diminishes renal injury associated with hemoglobinuria. Bilirubin is not soluble in aqueous solution and is transported in plasma from sites of synthesis to the liver bound to albumin. Bilirubin dissociates from albumin (Listowsky et al., 1978), and hepatocellular uptake is facilitated across the basolateral (sinusoidal) plasma membrane by the organic anion transport polypeptide (OATP), a sodium- and energy-independent process. OATP also has a role in the hepatic uptake of a wide range of other organic anions (e.g., certain drugs, other xenobiotics, sulfobromophlalein [BSP]) and in the sodium-independent hepatic uptake of bile salts (Hata et al., 2003). The primary mechanism for the hepatic uptake of conjugated bile salts is sodium dependent and utilizes the sodium taurocholate cotransporting polypeptide (NTCP) (Hata et al., 2003; Jacquemin et al., 1991, 1994; Wolkoff et al., 1985). Localization of OATP in the sinusoidal plasma membrane of the hepatocyte is determined by PDZ1, a 70-kDa PDZ binding motif that is present in the liver and kidney. (PDZ is an acronym that combines the first letters of the three proteins in which its binding domain was first identified; Hung and Sheng [2002].) PDZ domains function in protein targeting and in the assembly of specific protein-protein complexes involved in signal transduction or transport. In PDZK1 knockout mice, the expression of OATP1a1 in the liver is almost normal, but OATP1a1 is present only in the cytoplasm and is absent from its normal position in the plasma membrane. BSP clearance is diminished in PDZK1 knockout mice, suggesting that oligomerization of OATP1a1 with PDZK1 determines the subcellular localization and the function of OATP1a1 (Wang et al., 2005). Upon entry into the hepatocyte, bilirubin binds to ligandin, a major cytosolic protein that has both transport and detoxification functions. Ligandin is a glutathione S-transferase that catalyzes conjugation of reduced glutathione with a variety of endogenous substrates and xenobiotics including porphyrins and certain steroid hormones including cortisol, BSP, and indocyanine green (Habig et al., 1974; Kaplowitz et al., 1973). By binding bilirubin and inhibiting the efflux of pigment back into the plasma, ligandin serves as a driving force for initial hepatic uptake (Listowsky et al., 1978). Conjugation of bilirubin with glucuronic acid is catalyzed by bilirubin uridine-diphosphate glucuronosyltransferase-1 (BUGT1) in a reaction in which one or both propionic acid side chains of bilirubin IX are esterified. BUGT1 is a microsomal isoenzyme that produces water-soluble bilirubin mono- and diglucuronide. The UGT family of enzymes is among the most important mammalian detoxification mechanisms. Bilirubin diglucuronide represents more than 80% of the total bile pigment in healthy adult human bile. Glucuronic acid esters of bilirubin have been identified in the bile of a variety of species including the dog (Talafant, 1956), rat (Grodsky and Carbone, 1957), guinea pig (Schmid, 1956), and in the bile of the horse, pig, cat, sheep, and cattle (Cornelius et al., 1960). Gordon et al. (1976) have shown that the diglucuronide is the major bilirubin conjugate excreted in canine bile. There is evidence, however, that the bile of dogs (Fevery et al., 1971; Heirwegh et al., 1975; Noir, 1976) and other species (Cornelius et al., 1975a, 1975b) also contains bilirubin conjugates of glucose and xylose. When the activity of bilirubin-UGT in the liver is diminished, production of bilirubin mono- and diglucuronide decreases. Reduction of conjugating enzyme activity to approximately 30% of normal results in a modest increase in the serum bilirubin concentration. Greater deficiency of bilirubin-UGT leads to ineffective esterification of bilirubin and to more severe unconjugated hyperbilirubinemia and icterus. Bilirubin conjugation in the liver of neonatal animals is relatively low compared to the adult. Coupled with the high rate of bilirubin production related to accelerated neonatal erythrocyte turnover, the risk of hyperbilirubinemia and icterus may be increased in the neonate. Defective bilirubin conjugation as a result of inherited UDP-glucuronosyltransferase deficiency causes the severe unconjugated hyperbilirubinemia of the Crigler-Najjar syndrome of humans and the Gunn rat. Gilbert’s disease is a more benign, inherited form of UDP-glucuronosyltransferase deficiency in humans that is characterized by intermittent unconjugated hyperbilirubinemia and icterus. A similar enzyme deficiency may explain the benign unconjugated hyperbilirubinemia observed in some horses. The final step in hepatic excretion is the transport of conjugated bilirubin across the bile canaliculus and into the biliary system. The experimental intravenous infusion of unconjugated bilirubin at a rate that exceeds the maximal hepatic excretory capacity results in accumulation of conjugated bilirubin in plasma. This indicates that under normal conditions, the rate-limiting step in the transfer of bilirubin from plasma to bile is canalicular transport rather than either hepatic uptake or conjugation (Arias et al., 1961). The concentration of bilirubin glucuronide in bile is 150-fold greater than in hepatocytes. The unidirectional transport of bilirubin conjugates from the cytoplasm across the canalicular plasma membrane into bile is mediated by the multidrug resistance-associated protein 2 (Mrp2; ABCC2; canalicular multispecific organic anion transporter, cMOAT), a member of the ATP-binding cassette (ABC) superfamily, and an integral part of the bile canaliculus (Chowdhury et al., 1994; Nies and Keppler, 2007). The Mrp2 transport mechanism is functionally distinct from the ATP-dependent, bile salt export pump of the canaliculus described later in the section on bile acids (Bsep; Alpert et al., 1969; Arias et al., 1993; Muller et al., 1991; Stieger et al., 2007). Although mechanistically separate from the Mrp2 system, bile salt excretion enhances bile flow and increases the maximum transport capacity for bilirubin and other organic anions (Goresky et al., 1974). Mutations of the Mrp2 gene are responsible for the Dubin-Johnson syndrome of humans, a benign, inherited hepatic disease associated with conjugated hyperbilirubinemia and accumulation of melanin like pigment in hepatocytes. A similar disease has been observed in two separate strains of rats. In the GY/TR(-) rat (Jansen et al., 1985; Paulusma et al., 1996) and in the similar Eisai hyperbilirubinemic rat (EHBR; Ito et al., 1997, 2001; Takikawa et al., 1991), genetic sequence variants lead to premature stop codons and to the absence of the Mrp2 gene product in the canaliculus. A similar genetic abnormality is likely in Corriedale sheep, which develop an inherited disease that is clinically and biochemically identical to the Dubin-Johnson syndrome of humans (Cornelius et al., 1965a, 1968b) and rats (Kitamura et al., 1990). From the bile, conjugated bilirubin enters the intestine. Conjugated bilirubin, a polar compound, is poorly absorbed in the small intestine and passes to the large intestine where it is reduced to a series of colorless derivatives collectively called urobilinogens (stercobilinogens). Reduction is catalyzed by dehydrogenases of anaerobic colonic bacteria. In germ-free animals that lack intestinal microorganisms, bilirubin passes unaltered into the feces and urobilinogen is not produced (Gustafsson and Lanke, 1960). Most of the urobilinogen formed in the colon is passed in the feces, but some is absorbed into the portal circulation, transported to the liver, and most of that is excreted in the bile. A small fraction (1% to 5%) of absorbed urobilinogen, however, passes into the general circulation and is excreted by the kidney. In the dog, urobilinogen is excreted by both glomerular filtration and tubular secretion, the latter being enhanced in acid urine (Levy et al., 1968). Although the liver is the principal site of bilirubin conjugation and excretion, alternate pathways have been demonstrated. In normal animals, these alternate mechanisms are of minor significance but may become quantitatively more important in liver disease. After total hepatectomy, dogs have been shown to develop moderate hyperbilirubinemia and bilirubinuria. In addition to unconjugated bilirubin, the plasma of hepatectomized dogs contains the monoglucuronide conjugate (Hoffman et al., 1960) and, in some studies, the diglucuronide of bilirubin (Royer et al., 1965). The kidney and intestine both have been shown experimentally to be sites with the capacity to conjugate bilirubin (Royer et al., 1974). Differences in extrahepatic metabolism of bilirubin may explain some of the remarkable differences between species in the bilirubin levels reached after bile duct obstruction (see the following section). The clinical sign of icterus or jaundice develops when the yellow pigment bilirubin accumulates in plasma and other tissues. Yellow discoloration of tissues can first be noted by careful observation when the plasma bilirubin value exceeds 2 to 3 mg/dl and can be appreciated even by an untrained observer when the concentration exceeds 3 to 4 mg/dl. The correlation between the plasma bilirubin concentration and the degree of clinical icterus is not, however, perfect. Elevated plasma bilirubin values are usually present for one or more days before clinical icterus is apparent, and there may be a delay between the time plasma bilirubin returns to normal and the clearance of the yellow discoloration of tissues. Conjugated bilirubin is said to have a greater affinity for connective tissue than the unconjugated pigment, possibly because conjugated pigment is less avidly bound to albumin (With, 1968). Visible yellow discoloration of tissues is readily recognized in animals in the unpigmented sclera. The normal red color of the visible mucous membranes makes detection of a slight yellow cast more difficult. It is possible to apply pressure to the mucous membranes and temporarily reduce blood flow to the area, so that the underlying discoloration of the tissue can be better assessed. The color of plasma (icteric index) may be useful clinically in the evaluation of icterus. Normal canine, feline, and ovine plasma is often water clear and free of yellow color. The finding of yellow plasma in these species is highly suggestive of hyperbilirubinemia. Cattle absorb and transport significant quantities of carotene in plasma. Because in cattle the icteric index varies with the dietary intake of carotene, measurement has limited use in this species. Equine plasma normally has a high icteric index, which in part is due to a plasma bilirubin concentration that normally is higher than that of other domestic species. There are other, as yet uncharacterized, noncarotene pigments, however, that may contribute to the color of equine plasma. Notable species differences occur in the frequency with which icterus is observed in association with liver disease. In sheep and cattle with terminal hepatic insufficiency, there usually is a significant biochemical elevation in plasma bilirubin, but elevation may be insufficient to result in clinical icterus (Finn and Tennant, 1974; Hjerpe et al., 1971). This is due possibly to the residual capacity of the liver to excrete bilirubin or to extrahepatic mechanisms for bilirubin excretion or degradation. Clinical icterus in ruminants is often associated with hemolytic anemia in which production of bilirubin exceeds excretory capacity (e.g., anaplasmosis in cattle and copper poisoning in sheep). In severe fatty liver, cattle that are critically ill may exhibit some degree of clinical icterus. The assessment of clinical icterus in the horse is somewhat more complicated than in other species. The sclera and visible mucous membranes of most normal horses do not appear icteric, but in 10% to 15% of normal horses, a slight but definite yellow discoloration of the sclera or oral mucous membranes can be detected (Tennant et al., 1975). Scleral icterus of a moderate degree may also be observed in horses with a variety of illnesses that do not involve the liver directly (e.g., pneumonia, impaction of the large intestine, enteritis). Reduction in food intake is a common factor in such disorders, and fasting in the horse causes a rapid increase in plasma bilirubin concentration. In both hemolytic anemia and hepatic failure in the horse, the degree of icterus is usually remarkably greater than that seen under physiological conditions or that is associated with reduced food intake. In the horse, severe clinical icterus is almost invariably present in acute hepatic necrosis (Tennant et al., 1975; Thomsett, 1971). However, in chronic hepatic disease, icterus may be a more variable sign. In a series of 34 cases of hepatic cirrhosis in the horse, significant icterus was a presenting sign in 70% (Tennant et al., 1975). Icterus was even less frequent (40%) in another series of horses with cirrhosis (Gibbons et al., 1950). The dog and cat appear to be intermediate between ruminants and the horse in the propensity to develop clinical icterus. Hemolytic disease, severe hepatocellular dysfunction, and extrahepatic bile duct obstruction are characteristically associated with icterus in dogs and cats. In experimental extrahepatic bile duct obstruction in the dog, the plasma bilirubin increases at once following obstruction and clinical icterus is observed within 1 to 3 days. After 2 to 3 weeks, however, the plasma bilirubin of some dogs declines. As in sheep and cattle, this may be related to adaptation of extrahepatic mechanisms of bilirubin excretion, particularly the kidney. The kidney of the dog is capable of adapting so that the rate of renal excretion of bilirubin equals the rate of formation. In cats with complete extrahepatic bile duct obstruction, however, no such decrease is observed, and persistent hyperbilirubinemia and deep icterus are characteristic. Hepatic encephalopathy is the syndrome of disturbances in cerebral function that is caused by hepatic insufficiency or hepatic failure. Severity of neurological signs may vary from subtle and intermittent changes in behavior associated with lethargy or stupor, to bizarre, belligerent behavior, mania, convulsions, and hepatic coma. Typically, such signs are attributable to severe acute or chronic liver disease or in dogs to congenital malformation of the portal vein (portosystemic shunt). Hepatic encephalopathy is a prominent clinical feature of hepatic failure in the horse. In one series of cases, 82% of horses presenting with acute hepatitis and 32% with cirrhosis had prominent neurological abnormalities (Tennant et al., 1973). Varying degrees of CNS derangement may be observed. Some horses stand quietly with the feet apart and the head lowered, nodding the head occasionally and appearing somnolent. Pupillary response to light may be normal or moderately sluggish, but, in some cases, vision is lost. Compulsive walking in a circle or in a single direction may be observed, and affected individuals may appear oblivious to their surroundings, walking over or through objects in their path (“walking disease”; Rose et al., 1957). In fulminant cases, horses may become delirious with the head pressed forcibly against a wall for long periods of time, or they may assume a variety of other unusual positions or fall suddenly to the ground. Numerous unproductive attempts to rise can be followed by violent thrashing. When successful in rising, horses with hepatic encephalopathy may be completely uncontrollable, lunging forward violently and becoming a menace to attending personnel and equipment. The syndrome of hepatic encephalopathy in cattle may have an abrupt onset and characteristically represents a terminal manifestation of chronic liver disease (Fowler, 1968; Pearson, 1977). Affected calves initially may be dull, anorectic, and stand apart from other calves in a herd. Behavioral abnormalities may include unprovoked, violent charging or unusual and unrestrained bawling. Progressive dysmetria and ataxia are followed by recumbency and affected cattle may be unable to rise or even to assume a sternal position. Tenesmus has been reported to be a conspicuous clinical feature associated with prolapse of the rectal mucosa and dribbling of urine. Characteristically, death occurs within 2 days after onset of CNS signs (Finn and Tennant, 1974). Hepatic encephalopathy is observed frequently in dogs with congenital or acquired portosystemic vascular shunts and may be one of the most prominent presenting clinical features of this form of liver disease (Audell et al., 1974; Barrett et al., 1976; Cornelius et al., 1975a, 1975b; Ewing et al., 1974; Maddison, 1992; Schermerhorn et al., 1996). Neurological signs associated with portosystemic shunts often are episodic and may be present for some months before recognition of the underlying hepatic disturbance. Depression and stupor with amaurotic blindness are observed in approximately half the cases of congenital portacaval shunts in dogs, with circling, head pressing, and intermittent seizures observed less frequently. Hepatic encephalopathy also has been associated with other primary diseases of the canine liver (Center, 1996; Oliver, 1965; Strombeck et al., 1975b). Inherited diseases involving the urea cycle are recognized in domestic animals in which failure of urea synthesis results in significant elevations in blood ammonia and in signs of encephalopathy. Two cases of encephalopathy in dogs have been reported that were linked to a deficiency of argininosuccinate synthetase (Strombeck et al., 1975a). Inherited argininosuccinate synthetase deficiency has been described in neonatal cattle in which rapidly progressive and fatal encephalopathy was associated with hyperammonemia and citrullinemia (Harper et al., 1986, 1988, 1989). The argininosuccinate synthetase gene of cattle has been cloned, sequenced, and the mutation of affected Holstein calves described (Dennis et al., 1989). Suspected ornithine transcarbamylase deficiency has been described in a cat (Washizu et al., 2004), and encephalopathy in two Morgan foals associated with elevated blood ammonia has been recognized and described as being similar to the human syndrome of hyperornithinemia, hyperammonemia, and homocitrullinuria (McCornico et al., 1997). A syndrome of hyperammonemia and encephalopathy associated with acute gastrointestinal disease has been described in adult horses (Gilliam et al., 2007; Hasel et al., 1999; Peek et al., 1997; Stickle et al., 2006). The pathogenesis of this syndrome has not been fully explained, but the evidence suggests that increased production and absorption of ammonia from the colon are sufficient to exceed the detoxifying capacity of the liver (Stickle et al., 2006). Hepatic encephalopathy must be differentiated clinically from primary inflammatory, degenerative, or neoplastic diseases of the brain, and this can be accomplished by demonstrating the existence of underlying severe hepatic disease. In the horse with acute hepatitis, clinical icterus almost always is present at the time neurological signs are observed. In the dog and in cattle, frank clinical icterus is observed variably in animals with hepatic encephalopathy so that other tests of hepatic function or liver biopsy are required. In cases of primary hyperammonemia resulting from deficiency of urea cycle enzymes, conventional hepatic function tests are not expected to be abnormal (Strombeck et al., 1975a). Factors responsible for encephalopathy associated with hepatic failure are not completely understood. Ammonia is present in normal peripheral blood at a concentration of 2 to 5 mM/l. In portal venous blood, the concentration may be five times higher. Normally, most of the ammonia in the hepatic portal vein is removed by the normal liver to form urea, with only a small fraction passing into the systemic circulation. In hepatic failure, synthesis of urea is reduced, and in the horse (Cornelius et al., 1965a, 1965b; Tennant et al., 1975) and dog (Barrett et al., 1976; Strombeck, 1975b), significant elevations of blood ammonia have been demonstrated. Ammonia has potent neurotoxic effects, and many of the neurological signs of hepatic encephalopathy can be produced when toxic doses of ammonium salts are administered intravenously (Hooper, 1972). The reactions of blood ammonia are determined by the physicochemical principles that apply to gases in solution and to the dissociation of weak bases. The ammonia:ammonium ion buffer system of blood can be described by the Henderson-Hasselbalch equation: The pKa for this system in the dog is approximately 9.1 (Bromberg et al., 1960), meaning that at physiological pH (7.4), almost all of the ammonia of blood is ionized to form ( Because of its influence on acid-base parameters, potassium status may be an important determinant of NH3 toxicity. Potassium deficiency ultimately favors the development of metabolic alkalosis that, in turn, causes a shift in the NH3/ Blood ammonia ultimately is derived from dietary nitrogen. The gastrointestinal tract is the major source of blood NH3, but other tissues also produce NH3 (e.g., muscle and kidney). Renal ammonia is produced from glutamine and to a lesser extent from other amino acids. Synthesis of ammonium ion by the kidney represents a normal physiological mechanism for H+ excretion. The renal excretion of The gastrointestinal tract is the major source of blood NH3 based on the high concentration of NH3 found in portal blood compared to peripheral venous blood. Part of the ammonia in the hepatic portal vein is derived from the action of bacterial enzymes on dietary amino and amide nitrogen and part is derived from urea, which is present in alimentary tract secretions and is hydrolyzed by bacterial urease of the gastrointestinal tract. The question of whether gastrointestinal urease is produced in part by mammalian cells or is entirely of bacterial origin was the subject of controversy for many years. Using germ-free rats, it was demonstrated that gastrointestinal urease was exclusively of bacteria in origin (Levenson and Tennant, 1963), and this observation has been confirmed in germ-free dogs (Nance et al., 1974). The relative importance of NH3 produced by gastrointestinal bacteria and that produced from nonbacterial sources is still not fully known. Nance et al. (1971, 1974) and Nance and Kline (1971) have demonstrated that germ-free dogs with Eck fistulae develop encephalopathy associated with hyperammonemia. This suggests that at least with vascular shunts, endogenous sources of NH3 contribute to encephalopathy. The intestine metabolizes significant quantities of glutamine independent of intestinal bacteria, and at least 30% of the glutamine nitrogen that reaches the intestine appears in the portal blood as NH3/ The liver plays a critical role in maintenance of the blood glucose concentration, and marked hypoglycemia is sometimes associated with liver failure. In fulminant hepatic failure in the horse, the blood glucose has been reported in some cases to be as low as 20 mg/dl or less (Hjerpe, 1964; Tennant et al., 1975). Hypoglycemia also has been reported in dogs with hepatic insufficiency associated with vascular shunts (Cornelius et al., 1975b; Ewing et al., 1974). Other neurotoxic substances may be involved in the pathogenesis of hepatic encephalopathy, and the role of these factors has been reviewed (Center, 1996; Maddison, 1992). Indole and indolyl derivatives that are formed from tryptophan by intestinal bacteria have been suggested as encephalotoxic compounds capable of inducing coma (Zieve et al., 1974). Other studies have incriminated short chain fatty acids and, in experimental models of hepatic failure, total volatile fatty acids (VFA) increase significantly before death (Zieve et al., 1968). Increased plasma VFA concentrations also have been observed in spontaneous hepatic encephalopathy and, when infused intravenously into experimental animals, VFA produces cerebral depression followed by coma. The etiological role of ammonia in hepatic encephalopathy is generally recognized, and there is convincing evidence that cerebral edema is important in pathogenesis (Ahboucha and Butterworth, 2007; Butterworth, 1994). Astrocytes are recognized as a target of ammonia in the CNS (Albrecht and Norenberg, 2006), but how ammonia brings about astrocyte swelling, brain edema, and cerebral hypertension is not fully understood. It has been suggested that as a result of ammonia detoxification, glutamine accumulates in astrocytes and the resulting osmotic force of glutamine leads to cell swelling. In experimentally induced hepatic encephalopathy, accumulation of cerebral ammonia was associated with increased cerebral glutamine, with the development of Alzheimer type II astrocytes, and with a significant increase in the level of water in the brain (Jover et al., 2006). Based on studies of cultured astrocytes, Jayakumar et al. (2006) concluded, however, that glutamine was not acting as an osmolyte but rather that ammonia evoked oxidative stress or abnormalities of mitochondrial function (mitochondrial permeability transition) that caused injury and swelling of astrocytes (Albrecht and Norenberg, 2006; Norenberg et al., 2005, 2007). The management of hepatic encephalopathy involves restriction of protein intake and provision of a readily digestible dairy or vegetable protein source. Further control is directed at the reduction of the production and absorption of ammonia and other neurotoxic substances from the intestine. One approach is the oral administration of a nonabsorbable disaccharide such as lactulose, which upon reaching the colon is fermented by resident bacteria to produce short chain fatty acids and which has a cathartic effect. By decreasing colonic pH, the equilibrium between ammonium ion and the freebase is shifted to poorly absorbed ammonium ion. An alternative approach is the administration of a nonabsorbable, broad spectrum antibiotic. The antibiotic inhibits production of ammonia and other organic toxins produced by bacteria in the colon (Festi et al., 2006; Rothenber and Keeffe, 2005). Photosensitivity is the result of hypersensitivity to sunlight induced by the presence of exogenous or of endogenously produced photodynamic substances. Clinical signs develop when photosensitive animals are exposed to light and are caused by inflammation and necrosis of unpigmented skin (photodermatitis). A distinction is made between sunburn and photosensitization. Animals with unpigmented skin that are maintained for long periods of time indoors and are abruptly exposed to sunlight may sustain sunburn, which is a direct response of the unpigmented and unprotected skin to ultraviolet radiation (320 μM). Photosensitization is, characteristically, a more severe reaction caused by the interaction of a photodynamic substance and solar radiation. The effective wavelength of light causing photosensitization is determined by the absorption spectrum of the photosensitizing substance, which may extend into the visible region of light. Sunburn and photosensitization also differ in that sunburn apparently can develop in the absence of molecular oxygen, whereas photosensitization occurs only in the presence of molecular oxygen (Cook and Blum, 1959; Schothorst et al., 1970). With sunburn, there is a characteristic delay between exposure to light and the development of erythema of the skin, soreness, or pruritus. With photosensitization, initial clinical signs may be noted within minutes after exposure to sunlight. In domestic animals, three forms of photosensitization are recognized. Photosensitivity may occur when a photodynamic substance not present normally in the diet is ingested and absorbed. Examples are the ingestion of the poisonous plants Hypericum perforatum (St. John’s wort, Klamath weed) and Fagopyrum esculentum (buckwheat). Photosensitization caused by administration of the parasiticide phenothiazine also is a form of primary photosensitization. The photosensitizing compound is phenothiazine sulfoxide, which accumulates in the skin and in the aqueous humor inducing keratitis. A second form of photosensitization is caused by photosensitizing compounds that are produced endogenously. Congenital porphyria (pink tooth) of cattle is one of the best characterized examples in which a marked increase in production of uroporphyrin I results in deposition of the porphyrin in the teeth and bones, and large quantities are excreted in the urine. The teeth and urine readily fluoresce when exposed to ultraviolet light. The photodermatitis and hemolytic anemia associated with the disease are directly related to the photodynamic effects of endogenous porphyrins (Kaneko et al., 1971; Scott et al., 1979). Other inherited forms of porphyria in domestic animals (reviewed by Tennant [1998]) are described in Chapter 8. A third group of diseases associated with photosensitivity are those that are secondary to hepatic disease (hepatic photosensitization). Photodermatitis associated with both acute and chronic liver disease is recognized primarily in herbivorous animals. The photodynamic agent responsible for hepatic photosensitivity is phylloerythrin, a porphyrin derivative from chlorophyll (Rimington and Quin et al., 1934). Chlorophyll is converted to phylloerythrin by microorganisms of the rumen or large intestine that remove the magnesium atom from the chlorophyll molecule and hydrolyze the phytyl and carboxy methoxy side chains leaving the porphyrin nucleus of chlorophyll intact. Phylloerythrin produced in the alimentary tract is excreted primarily in the feces. A small fraction of the relatively nonpolar phylloerythrin is absorbed into the portal circulation. In normal animals, phylloerythrin is quantitatively removed by the liver and excreted in the bile and does not reach the peripheral circulation. Phylloerythrin may be found in the bile and feces of herbivores that are consuming chlorophyll-containing diets and may be demonstrated in other species that ingest chlorophyll. The comparatively large amount excreted by ruminants is attributed to their frequently high chlorophyll intake and to the favorable conditions for microbial production of phylloerythrin within the gastrointestinal tract. In hepatic insufficiency, phylloerythrin is incompletely cleared from the hepatic portal circulation, enters the systemic circulation, and ultimately accumulates in the skin. In the superficial layers of the unpigmented skin, phylloerythrin absorbs solar energy resulting in formation of free radicals. Reactive oxygen species cause peroxidation of cellular lipids and cellular components (e.g., lysosomes). Inflammation and necrosis of the skin are the result of direct oxidative injury and the secondary action of lysosomal enzymes (Slater and Riley, 1966). The critical range of wavelengths (action spectrum) that result in photodermatitis in hepatic photosensitivity was shown in geeldikkop to be between 380 and 650 μm (Riemershmid and Quin, 1941) and in facial eczema between 400 to 620 σm, ranges that are consistent with the known absorption spectrum of phylloerythrin. The types of hepatic disease of ruminants and horses that are associated with photosensitivity vary considerably, but the effects attributable to the photodynamic action of phylloerythrin are similar. The nature and severity of the cutaneous lesions depend on the amount of phylloerythrin in the skin and on the intensity and the duration of light exposure. The most common site of photodermatitis in the horse is the muzzle, which has a sparse protective covering of hair and often is unpigmented. Unpigmented areas of the distal extremities also are frequently affected (Fowler, 1965; Tennant et al., 1973). In cattle, unpigmented areas of the muzzle, back, escutcheon, and the lateral aspects of teats are especially susceptible to photodynamic injury. The areas of skin affected in sheep are those that receive the greatest exposure to light and that lack protection provided by black pigment or wool and include the ears, eyelids, face, lips, and coronets (Riemerschmid and Quin, 1941). The first clinical signs of photodermatitis in sheep may be apparent restlessness with shaking of the head or rubbing of affected parts. Individual animals may seek relief in the shade. Erythema and edema are the first cutaneous manifestations of photosensitization. Swelling of the lips, ears, and face have led to the descriptive terms “big head” and “facial eczema.” Following edema, serum may ooze from damaged skin. Ultimately, second- or third-degree burns may develop, and the morbidity and mortality attributable to lesions of the skin may be more important than any other aspect of the underlying liver disease (Riemerschmid and Quin, 1941). In the early stages of the disease, Southdown sheep with congenital photosensitivity have no morphological abnormalities of the liver, but the photodermatitis that is observed as soon as affected lambs begin to consume green plants is associated with significant biochemical defects in hepatic organic anion excretion (Cornelius and Gronwall, 1968). The clinical sign of ascites is the result of abnormal accumulation of fluid in the peritoneal cavity. In normal animals, there is significant bidirectional movement of fluid, electrolytes, and, to a lesser degree, protein across mesenteric capillaries, through the interstitial space, and across the peritoneal mesothelium into the abdominal cavity. Such movements are determined by osmotic and hydrostatic forces that are described by Starling’s equation: plasma colloidal osmotic pressure minus ascitic fluid colloidal osmotic pressure equals portal capillary pressure minus intra-abdominal hydrostatic pressure. Normal portal capillary pressure on the arterial side of the capillary bed favors formation of an ultrafiltrate of plasma, which is nearly protein free. On the venous side of the capillary bed, reabsorption of interstitial fluid occurs because the hydrostatic pressure is below that of the colloidal osmotic pressure primarily exerted by plasma proteins within the capillary bed. Under normal conditions, only a small volume of free fluid is present in the peritoneal cavity. During investigation of the mechanical factors that influence formation of lymph, Starling (1894) observed that obstruction of hepatic venous flow by ligation of the thoracic vena cava cranial to the site of entry of the hepatic veins produced significant increase in lymph flow through the thoracic duct, and the lymph was high in protein. Obstruction of the hepatic portal vein as it entered the liver also increased the flow of thoracic duct lymph, but the protein content was found to be low. Starling concluded that the increased flow of thoracic duct lymph following ligation of the thoracic vena cava arose from hepatic lymph, and after ligation of the portal vein, increased thoracic duct lymph was derived from mesenteric capillaries. Hepatic lymph is produced primarily in the sinusoids and contributes 25% to 50% of lymph flow in the thoracic duct (Brauer, 1963). In the dog and other species, hepatic lymph has a much higher protein content than lymph from other tissues because hepatocytes are not associated with a conventional basement membrane and because of the unique permeability of the sinusoids to plasma proteins because of fenestrations between the endothelial cells that line the sinusoids (Bissel and Maher, 1996). In experimental cirrhosis in the dog and other species, the flow rate of thoracic duct lymph is increased two to five times and the protein content is higher than that of lymph derived from other tissues (Nix et al., 1951a, 1951b). Ascites caused by cirrhosis of the liver may be associated with increased portal vein pressure (portal hypertension). Experimental ligation of the portal vein before it enters the liver, however, results only in minimal and transient ascites or no ascites (Schilling et al., 1952; Volwiler et al., 1950). In the dog, ligation of either the hepatic vein (Orloff and Snyder, 1961a, 1961b; Orloff et al., 1963, 1964a, 1964b, 1966) or the caudal vena cava at a site cranial to entry of the hepatic vein (Berman and Hull, 1952; Schilling et al., 1952; Witte et al., 1968, 1969a, 1969b) produces prompt and intractable ascites. Lymph fluid has been observed to form droplets said to “weep” from the surface of the liver following experimental obstruction of hepatic vein outflow (Hyatt et al., 1955). Because of its origin as hepatic lymph, the protein content of such ascitic fluid may be 3.0 to 3.5 g/dl or higher. This observation is consistent with the finding that the protein content of ascitic fluid in the initial stages of cirrhosis may be higher than that of conventional transudates (a modified transudate) because of its hepatic origin. As progressive fibrosis and “capillarization” of the hepatic sinusoids develop (Bissell and Maher, 1996), the protein content of hepatic lymph decreases and correspondingly the protein content of ascitic fluid decreases. It is probable in cases of cirrhosis that there is increased production of both hepatic lymph and mesenteric lymph and that ascites develops when lymph from both sources fails to return to the systemic venous circulation (Witte et al., 1971a, 1971b). The protein content of ascitic fluid is influenced not only by the relative proportions of mesenteric and hepatic lymph but by the protein concentration of plasma. In advanced cirrhosis, when hypoalbuminemia may be present, the protein content of ascitic fluid can be expected to be proportionately low. Although experimental portal vein obstruction per se does not result in ascites, only transient portal hypertension is actually produced by this procedure. When persistent portal hypertension is produced experimentally by aortic-portal anastomosis or when such anastomoses occur congenitally, the ascitic fluid is characteristically low in protein content because of its origin in mesenteric capillaries. Serum albumin is synthesized exclusively in the liver and is the major determinant of plasma and tissue fluid oncotic pressure. Hypoalbuminemia associated with chronic liver disease has been considered a factor contributing to the development of ascites, but current evidence suggests the role is not primary. The intravascular and total body albumin pools may not be greatly diminished in cirrhosis, although the concentrations of albumin in plasma often are decreased (Rothschild et al., 1973; Witte et al., 1971a, 1971b). For ascites to develop, total body sodium and water must expand. Excessive sodium chloride intake greatly enhances development of ascites (Berman and Hull, 1952), and ascites is preceded by increased sodium retention by the kidney. Aldosterone levels have been shown to be significantly increased in dogs with ascites caused by hepatic vein obstruction (Howards et al., 1968; Orloff et al., 1965). When associated with liver disease, ascites indicates a chronic process and characteristically presents with cirrhosis. There are important species differences in the occurrence of ascites in chronic liver disease. In dogs with advanced hepatic cirrhosis, ascites is a relatively common sign. Ascites is almost never observed in horses with cirrhosis (Tennant et al., 1975), and conspicuous ascites is unusual in cattle with cirrhosis (Finn and Tennant, 1974; Whitlock and Brown, 1969) but may be observed at necropsy (Pearson, 1977). Ascites has been observed in cattle with thrombosis of the caudal vena cava secondary to liver abscess (Braun et al., 1995), and, in such cases, marked hepatomegaly was characteristic (Breeze et al., 1976; Selman et al., 1974) and due apparently to obstruction of hepatic vein outflow. In sheep, ascites has been observed in cirrhosis but is unusual in cases of severe sclerosing cholangitis associated with fascioliasis (Hjerpe et al., 1971). It is important clinically to differentiate between ascites caused by liver disease and ascites caused by other primary diseases. Biochemical and cytological examination of ascitic fluid may be useful but alone is seldom diagnostic. The protein concentration of ascitic fluid associated with clinical cirrhosis may be variable depending on the stage of the disease (Center, 1996). In early stages, the protein content may be expected to exceed 2 to 2.5 g/dl because it is a reflection of the high protein content of hepatic lymph but later, when the serum albumin has decreased and when sinusoidal fibrosis and capillarization have developed, the protein content of ascitic fluid will be correspondingly lower (1 to 1.5 g/dl). The ascitic fluid associated with peritonitis is high, whereas in neoplastic diseases of the abdomen, the protein concentration is generally below 1.5 to 2.0 g/dl. Pembleton-Corbett et al. (2000) examined the serum albumin/peritoneal effusion albumin (SA/EA) gradient in dogs with ascites. They found the gradient was significantly higher in dogs with liver disease than in those with ascites of other causes. In 25 dogs with abdominal effusions associated with hepatic disease, 23 had serum albumin-effusion albumin (SA/EA) gradients equal to or greater than 1.1 and the median SA/EA gradient was 1.4 (range 0.7 to 3.1). In human patients, SA/EA gradients of 1.1 or greater are considered to indicate the presence of portal hypertension. The clinical observations of Pembleton-Corbett (2000) suggested that portal hypertension is a significant factor in the pathogenesis of ascites in dogs with hepatobiliary disease. Total nucleated cell counts in ascitic fluid from dogs with cirrhosis are seldom greater than 1000 to 2000 per μl. Bloody or turbid fluid typically results from inflammatory or neoplastic processes (e.g., feline infectious peritonitis, bacterial peritonitis, neoplasia), and nucleated cell counts are elevated. “Bile acites” or bile peritonitis is recognized most frequently in the dog and cat associated with abdominal trauma. In this case, the concentration of bilirubin in peritoneal fluid exceeds that of plasma. The pathogenesis of the hepatic diseases of domestic animal species is remarkably complex, involving acute and chronic forms of hepatitis, cirrhosis, bile duct obstruction, intrahepatic forms of cholestasis, neoplasia, and disorders of hepatic vasculature. The frequency of these diseases varies with species, breed, age, and, in some cases, by environment (diet, geographical location). The differential diagnosis of hepatic disease involves the evaluation of clinical history, physical examination, biochemical tests, hepatic imaging, and histopathological examination of hepatic biopsies. The following section describes the biochemical tests used to assess hepatic disease. There are several diagnostic categories with which the clinician dealing with problems of liver disease must be concerned. In clinical patients with a history and signs suggestive of hepatic disease, laboratory tests are used for confirmation. Laboratory tests are used to assess the severity of liver injury, to establish prognosis, to define treatable complications of hepatic insufficiency (e.g., ascites, encephalopathy), and to monitor clinical progress. Finally, biochemical tests of hepatic function may be performed on clinically healthy patients that are known to be at high risk of developing liver disease (e.g., exposure to infectious agents that cause hepatitis, or a familial history of chronic liver disease that requires screening for inherited diseases of the liver). The presence of liver disease often is recognized on the basis of elevated serum activities of enzymes of hepatic origin. Although they are sometimes referred to as “liver function tests,” serum enzymes do not measure hepatic function directly but indicate alteration in the integrity of the cell membrane of the hepatocyte, necrosis of hepatocytes or biliary epithelium, impeded bile formation or bile flow (cholestasis), or the induction of enzyme synthesis (Center, 2007). The serum enzyme activities that increase when hepatic necrosis is present are alanine aminotransferase (ALT), aspartate aminotransferase (AST), ornithine carbamoyltransferase (OCT), glutamic dehydrogenase (GD), sorbitol dehydrogenase (SDH), and arginase. Elevated serum activities of AP, GGT, and 5′ nucleotidase (5′-ND) are considered to indicate either intrahepatic or extrahepatic cholestasis. Because of its location between the splanchnic and systemic circulation, the liver is exposed to a wide variety of toxins, drugs and drug metabolites, bacterial toxins, and to infectious agents that may influence the serum activity of enzymes from the liver. The clinical assessment of aberrations in liver enzymes should consider the type of enzyme change (hepatocellular versus cholestatic), the degree of increase in serum enzyme activity, the rate at which the increase or decrease in serum activity occurs, and whether fluctuations in enzyme activity occur over time or if there is a unidirectional pattern of change in enzyme activity. The reference range is characteristically established as that within + /–2 standard deviations of the mean value observed in a “normal” animal population. By definition, this means that up to 2.5% of individuals from a “normal” population can be expected to have values above such a reference range. The serum activity of the aminotransferases, AST and ALT, are measured to detect hepatocellular injury. These enzymes catalyze the transfer of the α-amino nitrogen of aspartate or alanine to α-ketoglutaric acid resulting in formation of glutamate. AST and ALT have key roles in gluconeogenesis and in formation of urea. In the liver, ALT catalyzes the transfer of the α-amino nitrogen of alanine to α-ketoglutarate forming pyruvate, which can be utilized in gluconeogenesis. In muscle, ALT transaminates pyruvate to form alanine, which then transports non-ionized nitrogen from muscle to the liver for processing (glucose-alanine cycle). The activity of ALT is higher in the liver than in other tissues and in the dog, hepatic ALT is 10,000-fold higher than in plasma. Hepatic ALT activity is also high in cats, humans, and experimental rodent species in which measurement of serum ALT is used routinely in the assessment of hepatocellular injury. Hepatic ALT activity is lower in horses, cattle, sheep, and swine, and in these species, serum ALT is not measured routinely. The activity of AST is high in the liver of all domestic species and the serum activity is used routinely in all for evaluation of liver cell injury. However, AST activity also is high in the kidney, heart, and skeletal muscle, so elevations in serum AST are considered less specific for liver disease than elevations in serum ALT. There are differences in the intracellular distribution of ALT and AST within the hepatocyte. In the dog, most hepatic ALT and AST activity resides within the cytosol. An important fraction of AST (20%) and a lesser component of ALT are present within mitochondria (Keller, 1981). Distribution of the transaminases within the zones of the acinus also differs. ALT has the highest activity in Zone 1 hepatocytes, and AST has the highest activity in Zone 3 hepatocytes and the relative activity of ALT or AST in serum may reflect the acinar zone in which liver injury occurs (Rej, 1989). The largest increases in serum ALT are observed with hepatocellular inflammation and necrosis. In such conditions, progressive decreases in ALT activity may be a sign of recovery, and a 50% or greater reduction in serum ALT activity over several days is considered a favorable prognostic sign. Some animals with severe hepatic disease, however, may have normal serum ALT activity, and declining serum ALT activity may represent a significant reduction in viable hepatocytes or reduction in transaminase synthesis (e.g., microcystins, aflatoxin). Following severe, acute hepatocellular necrosis, in the dog serum ALT activity may increase by more than 100-fold within 24 to 48h, peaking during the first 5 postinjury days. If the source of the injury is eliminated, ALT activity will return more gradually to normal within 2 to 3 weeks. Hepatotoxicity induced by acetaminophen is associated with marked increases in serum ALT followed by a return to near normal values within 72h (Hjelle and Grauer, 1986; Ortega et al., 1985). Acute hepatocellular necrosis associated with infectious canine hepatitis (adenovirus) results in increased plasma ALT activity of 30-fold, peaking within 4 days (Wigton et al., 1976). Thereafter, a sustained increase in ALT activity may indicate development of chronic hepatitis. In all domestic species, the activity of AST is high in the liver and serum activity characteristically is increased in acute and chronic liver injury. Because AST activity also is high in the muscle, kidney, pancreas, and erythrocytes, when cells of these tissues are damaged, the AST activity of serum also can be expected to be elevated. There is no simple, specific, or direct method for determining the origin of increased serum AST activity, but additional laboratory tests may be useful. When AST is increased because of skeletal muscle disease including trauma (e.g., intramuscular injections) or degenerative disease, it may be useful to measure serum creatine kinase (CK). In muscle disease, AST and CK both are expected to be elevated. In acute muscle injury, elevation in the CK activity of serum may occur before AST is maximally elevated, and CK activity characteristically decreases before AST activity fully declines. When coincidental myopathy and liver disease occur in dogs and cats, measurement of serum ALT may be useful, but severe primary muscle disease is sometimes associated with increased serum ALT activity. Transaminase activity is known to increase following vigorous exercise in dogs (Valentine et al., 1990), but the origin of the enzyme is not clear (Bolter and Critz, 1974; Loegering and Critz, 1971). A 1.4- to 2-fold increase in plasma AST in dogs associated with increases in CK and lactic dehydrogenase has been observed after short-term exercise and similar increases in plasma AST and lactic dehydrogenase activity were detected following electrophysiological stimulation of hind limb muscles (Heffron et al., 1976). The results of measurement of the half-life of transaminases following intravenous injection of hepatic homogenates have varied widely. In one study, three dogs injected with a 20% liver homogenate and sampled over 3 days, the average T½ for AST was 263 min and for ALT was 149 min (Zinkl et al., 1971). In another study, seven dogs received the supernatant of a liver homogenate intravenously, and a much longer time period was required for clearance with the T½ for ALT determined to be 59 ± 9 h and for AST 22 ± 1.6 h (Dossin et al., 2005). The plasma T½ of AST in the cat has been estimated to be 77 min (Nilkumhang and Thornton, 1979). Sustained elevations in transaminase in acute liver disease may be the result of delayed clearance. Catabolism of plasma transaminases, in part, is the result of endocytosis by hepatocytes, and enzyme clearance may be delayed because of the underlying hepatic disease (acquired portosystemic shunting, nodular regeneration, hepatic fibrosis; Horuichi et al., 1985; Kamimoto et al., 1985). The major value of serum AST and ALT measurements is in detecting hepatocellular injury and monitoring clinical progress (Table 13-1). Because both enzymes are increased in a variety of hepatic diseases, they are of limited value for differential diagnosis. Although elevations of the aminotransferases are generally considered indicative of hepatocellular injury, in severe forms of liver disease, both hepatocellular and cholestatic forms of hepatic injury often coexist. The highest aminotransferase levels are associated with acute hepatitic injury, but more modest increases in aminotransferase activity are seen in chronic liver disease including chronic hepatocellular disease, cirrhosis, parasitic hepatopathy, and primary or metastatic neoplasia.
I. INTRODUCTION
II. FUNCTIONAL ANATOMY
III. CLINICAL MANIFESTATIONS OF HEPATIC INSUFFICIENCY
A. Icterus
1. Formation of Bile Pigments
2. Hepatic Excretion of Bilirubin
3. Extrahepatic Metabolism of Bilirubin
4. Icterus
B. Hepatic Encephalopathy
). As blood pH increases, the relative amount of free ammonia (NH3) increases, and as pH decreases, NH3 decreases. Cells are almost impermeable to
but are readily permeable to NH3, passing through the plasma membrane by nonionic diffusion (Castell and Moore, 1971; Stabenau et al., 1959; Warren and Nathan, 1958). These principles are important in determining the amount of ammonia absorbed from the gastrointestinal tract or the amount that can pass from blood into the brain and to other tissues (Dimski, 1994).
equilibrium in the direction of the toxic freebase.
is related directly to the pH gradient between blood and urine, and total urinary excretion of
is high in acid urine and proportionately low in alkaline urine.
(Windmuller and Spaeth, 1974).
C. Hepatic Photosensitivity
D. Ascites
IV. LABORATORY ASSESSMENT OF HEPATIC FUNCTION
A. Hepatic Enzymes
1. Serum Alanine and Aspartate Aminotransferases
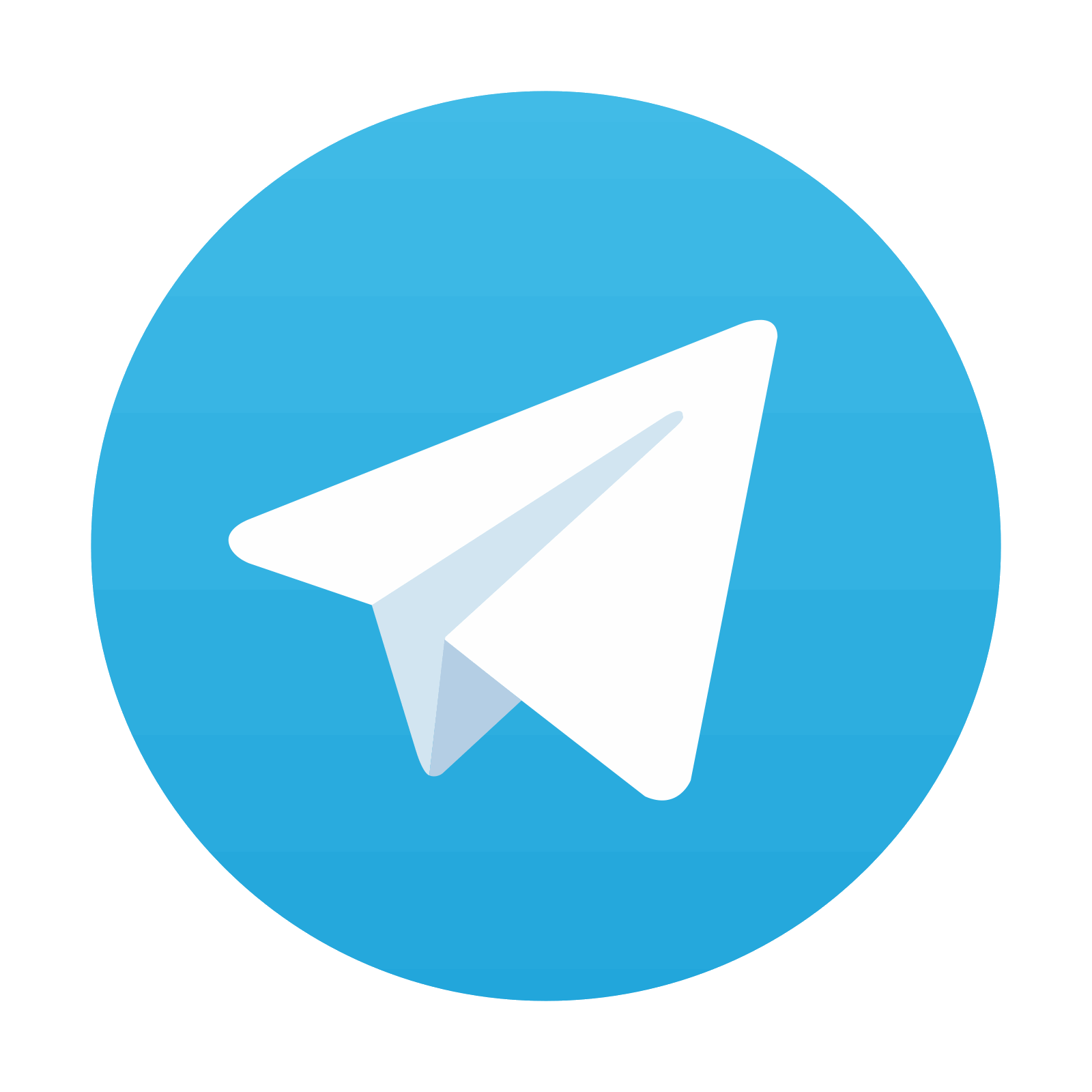
Stay updated, free articles. Join our Telegram channel
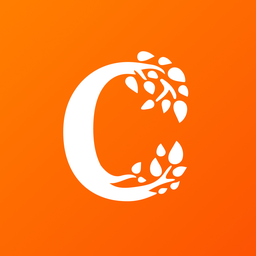
Full access? Get Clinical Tree
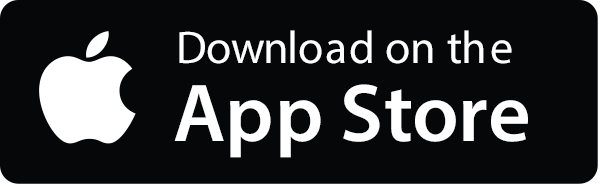
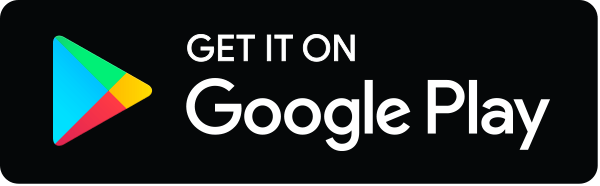