The horse is often considered one of, if not the, premier athletic mammalian species. However, depending upon the criterion used, there are other distinct (and sometimes surprising) contenders for that title. For example, as seen in Figure 31.1, compared with the Thoroughbred racehorse (40 miles/h, 65 km/h), the cheetah can achieve speeds in excess of 70 miles/h (120 km/h) and several species of antelope, as well as the blackbuck, gnu, and ostrich, are all faster than the horse (fastest human 27–28 miles/h, ~44 km/h). The fastest horses ever clocked are Quarter Horses, which reach speeds of 50–55 miles/h (~85 km/h).1 Because body length determines the distance that individual muscles shorten, it might be more appropriate to judge athletic ability in terms of speed relative to body length.2 From this perspective, the Merriam kangaroo rat is superlative, achieving 110 body lengths per second, which is three times faster than the cheetah (32 length/s) and an order of magnitude faster than the horse (~10 length/s). Within humans, the aerobic capacity or maximal oxygen uptake ( This chapter addresses the structural and functional capacities of the cardiovascular system that permit the horse to achieve such prodigious O2 flows and athletic performances. As we shall see, a large, high-capacity heart is requisite. Whereas animals such as the shrew and pronghorn antelope have evolved in accordance with the laws of nature, the horse has been subjected to several thousand years of selective breeding (Fig. 31.2)6a based upon athletic performance. As detailed below, this practice has produced a disproportionate increase in the horse’s heart size and pumping capacity (cardiac output, The purpose of this chapter is to present those features of the equine heart and cardiovascular system that facilitate this animal’s extraordinary performance. Those mechanisms underlying respiratory system failure will be discussed as they relate intimately to the cardiovascular system. Particular attention will be afforded to the plasticity of the equine cardiovascular system to exercise training and, although such changes are important, compared with the magnitude of inherited inter-animal variations, they are relatively modest. Throughout this chapter and contingent upon available data, reference will be made to several other species, including the human, dog, ox, and camel, as a basis for comparison (Fig. 31.3).7 The energetic capability of skeletal muscle is so high that it far surpasses the capacity of the respiratory and cardiovascular systems to deliver O2. In Thoroughbreds, skeletal muscle comprises over 50% of body mass8 and aerobic capacity ( • Breathing high O2 mixtures elevates arterial O2 content and • With respect to equine • Pericardectomy elevates stroke volume, cardiac output and • Restricting the exercising muscle mass in humans to 2–3 kg (knee extensors) rather than the 15–20 kg recruited during running or cycling elevates mass specific • Blood doping (reinfusion of autologous red cells) elevates Several steps in the pathway of O2 from the atmosphere to its site of utilization within muscle mitochondria may limit the achievable Therefore: In the horse during maximal exercise, SV is determined principally by heart size and HR may approach 240 beats/min in young adults, which is unusually high for such a large animal (though it does decrease with advancing age and other factors20). Circulating hemoglobin concentration ([Hb]) increases nearly twofold above rest as red blood cells are released from the large muscular spleen in response to increased sympathetic activity. Table 31.1 demonstrates that the size of the heart and spleen is relatively larger in the horse than in either the ox or man. Relative heart size in the athletic dog (i.e., greyhound24–26) approaches that found in the horse, but the horse is unusual in that the spleen is so large. Note that in the horse, the proportion of skeletal muscle is very high (close to 50%) and the lungs are relatively small (particularly with respect to heart size, see ‘Cardiovascular physiology and responses to exercise’ below). A comparison between actual heart size and Table 31.1 Relative comparison of the mass of organs key to the loading and transport of O2 as a % of body mass Data from Webb and Weaver23 and Haun.31 Note ‘Dog’ excludes greyhounds. where CaO2 and CvO2 denote arterial and mixed venous O2 contents. Figure 31.7 demonstrates that the effective muscle diffusing capacity (estimated by the slope of the line projecting from the origin) determines the level to which CvO2 will fall at maximal exercise (i.e. extraction) and also the This chapter deals primarily with horses in their athletic prime and considers their maximal capacities irrespective of age per se. In this respect, horses increase their cardiovascular (Fig. 31.9) and muscle oxidative enzyme (Table 31.2) capacities substantially during their first 3 years. Thus, 3- to 4-year-old horses have larger hearts,27,29 and a lower HR27 (larger stroke volume) at a given running speed, as well as a reduced blood lactate response to submaximal running speeds (Fig. 31.10).27 The mechanistic bases for these last two alterations, as they relate to increased heart size and vascular adaptations which improve muscle O2 delivery and exchange, are encompassed within the training section of this chapter (‘Exercise training’). Table 31.2 Relative citrate synthase (CS, carbohydrate metabolism), 3-hydroxy-acyl CoA dehydrogenase (HAD, fat metabolism) and lactate dehydrogenase (LDH, lactate→pyruvate interconversion) activities in the middle gluteal muscle of Thoroughbreds and Standardbreds of different ages Significance over the four age groups: XX, P < 0.01; XXX, P < 0.001. (Based upon data from Snow and Valberg.28) The size of the heart is a key determinant of maximum stroke volume, cardiac output, and hence aerobic capacity and exercise performance (Figs 31-11–31.14). This relationship has been documented in humans by examination of the electrocardiogram (ECG), ultrasound, radiographs, and post-mortem examination of heart size. For example, Paavo Nurmi, multiple Olympic champion distance runner, reportedly had a heart mass nearly three times larger than predicted for his body size. At post-mortem, the heart of the seven-time Boston Marathon winner, Clarence DeMar, who died of a non-myocardial cancer, was substantially larger than normal and his coronary arteries were threefold larger than found in his non-athletic counterparts.31 In horses, heart mass approximates 0.9–1% of body mass, which is greater than that for other non-athletic species (Fig. 31.14) and may exceed 1.1% of body mass in trained horses, possibly reaching ~2% in elite horses.23,26 Amongst different horse breeds, racing horses have proportionally larger hearts (Fig. 31.14) and Table 31.3 lists some famous horses and their heart masses. The heaviest horse heart actually weighed was that of Sham at 18 lb (8.2 kg), who was consistently runner-up to Secretariat. Secretariat was a Triple Crown winner and holds the track record at Belmont Park (2 min 24.4 s for 1.5 miles on turf). Tragically, Secretariat’s heart was never weighed. However, the same pathologist, Dr Thomas Swerczek, who weighed Sham’s heart estimated that Secretariat’s heart weighed 22 lb (10 kg) and he considered it to be in perfect condition.31 If that mass is correct, Figure 31.13A predicts that Secretariat may have achieved a cardiac output in excess of 500 L/min and Figure 31.13B a Table 31.3 Heart mass and heart scores of famous racehorses b, bay; ch, chestnut; g, gelding; m, mare; s, stallion. aSome pathologic enlargement (may add 2–3 lb to heart mass). (Reprinted from Haun M, 1997, The X factor – what it is and how to find it, with kind permission from Flying Island LLC.) Given the crucial importance of heart size in setting athletic potential, there has been great interest in establishing a convenient and accurate non-invasive method of estimating this variable in horses. The mean/average duration of the QRS complex (in ECG leads I, II, and III, Fig. 31.16) has been shown to correlate with heart mass at autopsy and also racing performance.32 Thus, larger hearts have a wider QRS complex and the so-called ‘heart score’ measured in milliseconds has been related to heart mass and subsequently predicted stroke volume and cardiac output, as shown in Table 31.4 and Table 31.5. It should be noted, however, that other studies have not been able to confirm the relationship between heart score and heart mass.33–37 Based upon the inheritance of heart scores in racehorses,38 there is currently great interest in identifying the gene, located on the X-chromosome, that codes for heart mass.31 Echocardiographic and cardiac ultrasound techniques have demonstrated that a significant relationship exists between left ventricular mass and Table 31.4 Relationship derived between heart mass and heart score (Reprinted from Haun M, 1997, The X factor – what it is and how to find it, with kind permission from Flying Island LLC.) Table 31.5 Relationships derived among heart score, heart mass, and stroke volume and cardiac output at maximal exercise aEstimated by Steel (unpublished data 1977). As shown above, O2 delivery depends not only upon cardiac output ( In the Thoroughbred, total blood volume approximates 10% of body mass46,47 and of this approximately 50 L of blood, 75% resides in the systemic circulation, of which 60% is in the highly distensible venous system and only 15% in the arteries. Depending upon the activity undertaken (e.g. digestion, thermal stress, exercise), the cardiovascular system must redistribute The pulmonary vasomotor tone can be influenced by a variety of neural and humoral factors. Specifically, pulmonary arteries have both sympathetic and parasympathetic innervation and respond to serotonin, epinephrine (adrenaline), norepinephrine (noradrenaline), isoproterenol, acetylcholine, angiotensin II, leukotrienes, prostacyclins, histamine, thromboxane, bradykinin, and arachidonic acid. However, as seen in Figure 31.20, the horse has relatively little pulmonary vascular smooth muscle compared with cattle and pigs.54 Thus, the horse exhibits only a weak vasoconstrictive response which is most evident in the low susceptibility of the horse to pulmonary hypoxic vasoconstriction but which causes a profound pulmonary hypertension in cattle and pigs at altitude. During exercise, the elevated pulmonary arterial pressures induce recruitment and distension of the vascular bed, which reduces vascular resistance, elevates vascular conductance, and increases pulmonary capillary volume (Fig. 31.21). Despite this elevated conductance, pulmonary vascular pressures do become extraordinarily high during maximal exercise (see ‘Cardiovascular physiology and responses to exercise’ below). The pulmonary capillaries form a dense plexus around each alveolus and, unlike their systemic counterparts, are not embedded within a supporting tissue matrix and thus are subject to collapse at positive alveolar pressures, particularly when perfusion pressure is low. In the horse, these vessels are about 6–7 µm in diameter56 (which is narrower than found in dog or rabbit lungs) and have a total wall thickness (capillary endothelium, basement membrane, alveolar epithelium) which averages only 0.9 µm (Fig. 31.22). Consequently, even at relatively low transmural pressures (positive luminal plus negative alveolar), these vessels bulge into the alveolar space and may rupture during exercise (exercise-induced pulmonary hemorrhage). Pulmonary capillary blood volume constitutes only a very small fraction of that present in the pulmonary circulation, which confers the advantage of maximizing blood-gas spatial contact but has the consequence of limiting red blood cell transit time in the capillary. Pulmonary capillary blood volume in the horse is 60–80% greater than that of a steer of the same mass.57 In healthy animals as for humans, active vasomotor control is traditionally thought to play a relatively minor role (compared with the effects of hydrostatic pressure gradients, lung volume, and alveolar pressure) in setting the distribution of blood flow within the pulmonary circulation.53 However, more recent investigations have demonstrated that there is a gravity-independent distribution of
Heart and vessels
Function during exercise and training adaptations
Introduction
) is considered an excellent (though by no means the sole) indicator of performance for running events over 1 minute in duration. Across the spectrum of terrestrial mammalian species, aerobic capacity increases over five orders of magnitude as a function of body size from approximately 0.001 L/min in the 2 g Etruscan shrew (world’s smallest mammal) to in excess of 80 L/min in the elite Thoroughbred racehorse. Whereas it is possible that a large rapidly walking elephant may have a higher total
, this remains to be demonstrated. When aerobic capacity is expressed relative to body mass, the diminutive Etruscan shrew (
~400 mL O2/kg/min)2,3 reigns supreme, as this measure of performance decreases systematically with increasing body mass. Amongst the larger animals, the pronghorn antelope (
~300 mL O2/kg/min)4 and the horse (
> 200 mL O2/kg/min)2,5 are outstanding and each can consume more O2 in toto and per unit body mass than any other mammal of their respective sizes.
) compared to lung capacity; the consequence of this is a structural and functional failure of the respiratory system during maximal exercise.
Role of the heart and cardiovascular system in setting aerobic capacity
) (at least of Standardbreds) increases as a function of fat-free mass.9 Moreover, under many conditions
is considered to be O2 supply-limited because the mitochondrial oxidative enzyme capacity for utilizing O2 exceeds that of the cardiorespiratory systems to deliver O2. In support of this notion, there is strong evidence that increasing muscle O2 delivery during intense exercise will elevate
. Specifically:
in horses10 and humans.11
, there is recent evidence that horses run to maximal speeds on an incline (6o) exhibit higher cardiac output (
) (Fig. 31.4) and
values than on the flat. This increased
and
results from an elevated stroke volume12 and may relate to greater hindlimb muscle recruitment, greater swings in intrapleural pressures (higher tidal volume) aiding heart function, and/or increased hydrostatic pressures improving muscle blood flow.
) and also elevates
. Reprinted from McDonough P, Kindig CA, Hildreth T, et al. Effect of body incline on cardiac performance. Equine Vet J Suppl 2002; 34:506-509, with permission of the Equine Veterinary Journal.
in dogs13 and pigs.14
substantially.15,16
in humans.17
.18,19 These include O2 diffusion across the blood-gas barrier in the lungs, conductive transport of O2 in the blood
, and O2 concentration (CaO2), and diffusion of O2 from the skeletal muscle capillary into the myocyte. The coordinated function of respiratory, cardiovascular, and muscular systems provides for rapid changes in O2 flux from lungs to mitochondria (Fig. 31.5) and in most species, including the horse, human, and dog, the strongest determinant of
is the capacity of the cardiovascular system to transport O2 (i.e.
, Fig. 31.6). However, during maximal exercise in the horse other steps in the O2 pathway assume greater importance for limiting
than in other species, in large part because of the disproportionality between the cardiovascular and respiratory systems. For example, O2 loading in the lung is impaired and arterial hypoxemia becomes manifested (see ‘Cardiovascular physiology and responses to exercise’ below). In addition, the ability to offload O2 in the muscle capillary is constrained by the finite O2-diffusing capacity of skeletal muscle which is determined by the capillary bed and blood flow within those capillaries. Figure 31.7 integrates the conductive and diffusive elements of O2 transport to demonstrate how the horse achieves its very high
.
, mitochondrial O2 delivery/utilization;
, oxygen uptake;
, CO2 output, SV, stroke volume; HR, heart rate; VT, tidal volume; f, breathing frequency; CO2, carbon dioxide; Creat-PO4, creatine phosphate; Pyr, pyruvate; Lac, lactate;
, mitochondrial carbon dioxide production. Reprinted from Wasserman K, Hansen JE, Sue DY, et al. Principles of exercise testing and interpretation, 2nd edn. Philadelphia: Lea & Febiger; 1987, with permission from John Wiley and Sons, © 1988 Wiley Periodicals, Inc.
) and maximal oxygen uptake (
) after bedrest and exercise training in five humans. Reprinted from Saltin B, Blomqvist G, Mitchell JH, et al. Response to exercise after bed rest and after training. Circulation 1968; 38 (suppl VII):1-78, with permission of Wolters Kluwer Health.
) by conductive (
) and diffusive movement of O2 by the cardiovascular and muscle microcirculatory systems (‘Wagner’ diagram18). The curved line denotes mass balance according to the Fick principle and the straight line from the origin represents Fick’s law of diffusion. DO2 is effective diffusing capacity and K is a constant that relates venous PO2 to mean capillary PO2. PvO2, CaO2, and CvO2 are the partial pressure of venous O2 and the concentrations of O2 in arterial and venous blood, respectively.
occurs at the intersection of the two relationships. (A) is a general schematic whereas (B) presents actual values for a very fit Thoroughbred at maximal exercise. Understanding the conductive and diffusive determinants of
is essential for interpreting the structural and functional mechanisms that increase
with exercise training . See pp. 670 and 671 for additional details. Wagner PD, Hoppeler H, Saltin B. Determinants of maximal oxygen uptake. In: Crystal RG, West JB, Barnes PJ, et al, eds. The lung: scientific foundations, 2nd edn. New York: Lippincott-Raven; 1997; 2033-2041.
Conductive O2 transport (lungs to muscle)
in the athletic horse and human is shown in Figure 31.8.
HORSE
DOG
OX
MAN
Spleen
up to 1.1
0.3
0.2
0.3
Heart
0.7–2.0
0.8
0.4
0.5
Lungs
0.9–1.5
0.9
0.7
1.4
Diffusive O2 transport within muscle
. The section ‘Cardiovascular physiology and responses to exercise’ details the determinants of muscle O2 diffusing capacity.
Age
Sex
ENZYME ACTIVITIES (SCALED TO 1 AT AGE 1 FOR MARES (M, FILLY) AND STALLIONS (S, COLT))
Thoroughbreds
Standardbreds
CS
HAD
LDH
CS
HAD
LDH
1
M
1
1
1
1
1
1
1
S
1
1
1
1
1
1
2
M
1.4
1.1
1.2
1.2
1.1
1
2
S
1.3
1.2
0.8
1.4
1.1
0.9
3
M
2.1
1.6
0.9
1.9
1.1
0.7
3
S
1.8
1.7
0.9
1.8
1.43
0.68
4–6
M
2.2
1.6
0.8
1.9
1.1
0.9
4–6
S
2.1
2.1
0.8
2.3
1.5
0.8
Age
XXX
XXX
XX
XXX
NS
XXX
Sex
NS
NS
NS
NS
NS
NS
Anatomy of the cardiovascular system
Heart size
) (A) and
and cardiac output at maximum exercise (B). (A) The solid symbols are determined from the data of Evans and Rose (1988);30 the hollow symbols are determined from that relationship and the measured or estimated (Secretariat) heart masses published for each named horse.31 (B) An arterial–venous O2 difference of 22.8 mL/100 mL of blood is assumed to estimate
values for Secretariat and Sham. The echocardiographic data of Young et al.5 are consistent with this relationship. Note Secretariat’s extraordinary
(~ 540 L/min) and
(over 120 L/min, which would be 240 mL O2/kg/min at 500 kg body mass).
over 120 L/min! To place heart size in visual perspective, Figure 31.15 compares Key to the Mint’s 15.8 lb (7.2 kg) heart to that of an unexceptional stallion.
NAME OF HORSE (COLOR, SEX, YEAR BORN)
HEART MASS
HEART SCORE (ms)
lb
kg
Secretariat (ch.s. 1970)
22
10
Shama (ch.s. 1970)
18
8.2
Mill Reefa (b.s. 1968)
16.9
7.7
Key to the Mint (b.s. 1969)
15.8
7.2
157
Easy Goer (ch.s. 1986)
15
6.8
Althea (ch.m. 1981)
15
6.8
Eclipse (ch.s. 1764)
14
6.4
Phar Lap (ch.g. 1926)
14
6.4
Star Kingdom (ch.s. 1946)
14
6.4
Tulloch (b.s. 1954)
13.5
6.2
136
Killaloe (b.m. 1970)
12.9
5.9
Northern Dancer (b.s. 1961)
150
Soviet Problem (ch.m. 1990)
150
Moscow Ballet (b.s. 1982)
147
The Last Red (ch.m. 1993-twin)
140
Desert Secret (b.s. 1990)
140
Hyperion (ch.s. 1930)
133
Vo Rouge (b.g. 1983)
130
max in Thoroughbred racehorses.5 Moreover, positive relationships among left ventricular size, systolic function, and race performance are emerging.39,40 These latter findings offer some hope that non-invasive evaluation methods may prove valuable in helping to select for athletic potential.
HEART SCORE (ms)
HEART MASS
lb
kg
100
6.6
3.0
110
7.4
3.4
120
10.1
4.6
130
11.9
5.4
140
13.6
6.2
150
15.4
7.0
160
17.2
7.8
HEART SCORE (ms)
HEART MASS (kg)
STROKE VOLUME (L)a
CARDIAC OUTPUT (L/min AT MAX EXERCISE)b
100
3.0
0.5
100
110
3.8
0.75
150
120
4.6
1.0
200
130
5.4
1.25
250
Spleen
) but also upon arterial O2 content (CaO2), and the equine spleen is of paramount importance for setting the horse’s high exercising blood hemoglobin concentration ([Hb]) and thus CaO2. Splenic contraction may dump 12 L or more of red cells into the circulation, thereby doubling the number of circulating red blood cells.41–44 In keeping with the importance of
in determining racing performance, splenectomy reduces
by over 30% in the Thoroughbred.43 Splenic reserve is correlated with spleen mass and total blood volume but not body mass per se.36,41,44 Racing horses have a significantly greater splenic mass than non-racing breeds, i.e. Arabian, stock, draft32 (Fig. 31.17). There is some evidence that erythropoietin treatment increases
via elevated circulating hemoglobin concentration in Standardbred horses.45 However whether this is efficacious for the racing Thoroughbred who may already be at a hematocrit approaching 70% remains to be determined.
Systemic circulation and microcirculation
amongst the appropriate organs. Physical exercise produces the most profound physiological stress to the horse and, as shown in Figure 31.18, the percentage of
that perfuses the splanchnic region and kidneys is reduced from ~50% at rest to only 5% at maximal exercise. By contrast, exercising skeletal muscle may receive 80–90% of
compared with only 10–20% at rest. Such flexible and precise control over blood flow distribution demands a network of powerful muscular arterioles that provide the principal resistance to flow in the systemic circulation and which can dilate or constrict rapidly in response to the vasoactive effects of muscle metabolites, prostacyclins, and nitric oxide (dilation), or sympathetic stimulation, angiotensin, and endothelin (constriction). From rest to maximal exercise, skeletal muscle blood flow may increase over 60–70-fold. Although the perfusion pressure does increase substantially, elevated muscle vascular conductance (dilation) constitutes the primary mechanism by which the increased muscle blood flow (
) is achieved, and this response is detailed in the section ‘Cardiovascular physiology and responses to exercise’ below. Following a systematic series of bifurcations through several orders of progressively narrower arterioles, the vascular tree ramifies into a series of capillaries which form the principal site for blood-tissue exchange (Fig. 31.19). The capillary wall is devoid of smooth muscle and presents a barrier typically less than 1 µm thick between the capillary blood and the myocyte sarcolemma. The density, volume, and surface area of the skeletal muscle capillary bed are correlated closely with oxidative capacity and thus muscle fiber type.51,52 In equine muscle (transverse section across fibers), there may be between 400 and 800 capillaries per square millimeter with a mean diameter of 4–6 µm,49,53 and these contain over 80% of the intramuscular blood volume. As we shall see in the section ‘Cardiovascular physiology and responses to exercise’ below, the capillary volume is crucial for setting red blood cell transit time in the capillary and facilitating O2 offloading during exercise (or loading in the pulmonary capillary).
) at rest and during maximal exercise. In the highly trained, very fit Thoroughbred, it is possible that skeletal muscle blood flow may reach as much as 90% of cardiac output. Values from Erickson et al.48
Pulmonary circulation and microcirculation
toward the dorsal aspect of the equine lung (Fig. 31.23).58 This effect may be explained by a regionally dependent arteriolar reponsiveness. For example, arterioles in the upper lung regions exhibit a pronounced vasodilation to methacholine (an endothelium-dependent vasodilator) whereas those at the bottom do not.59
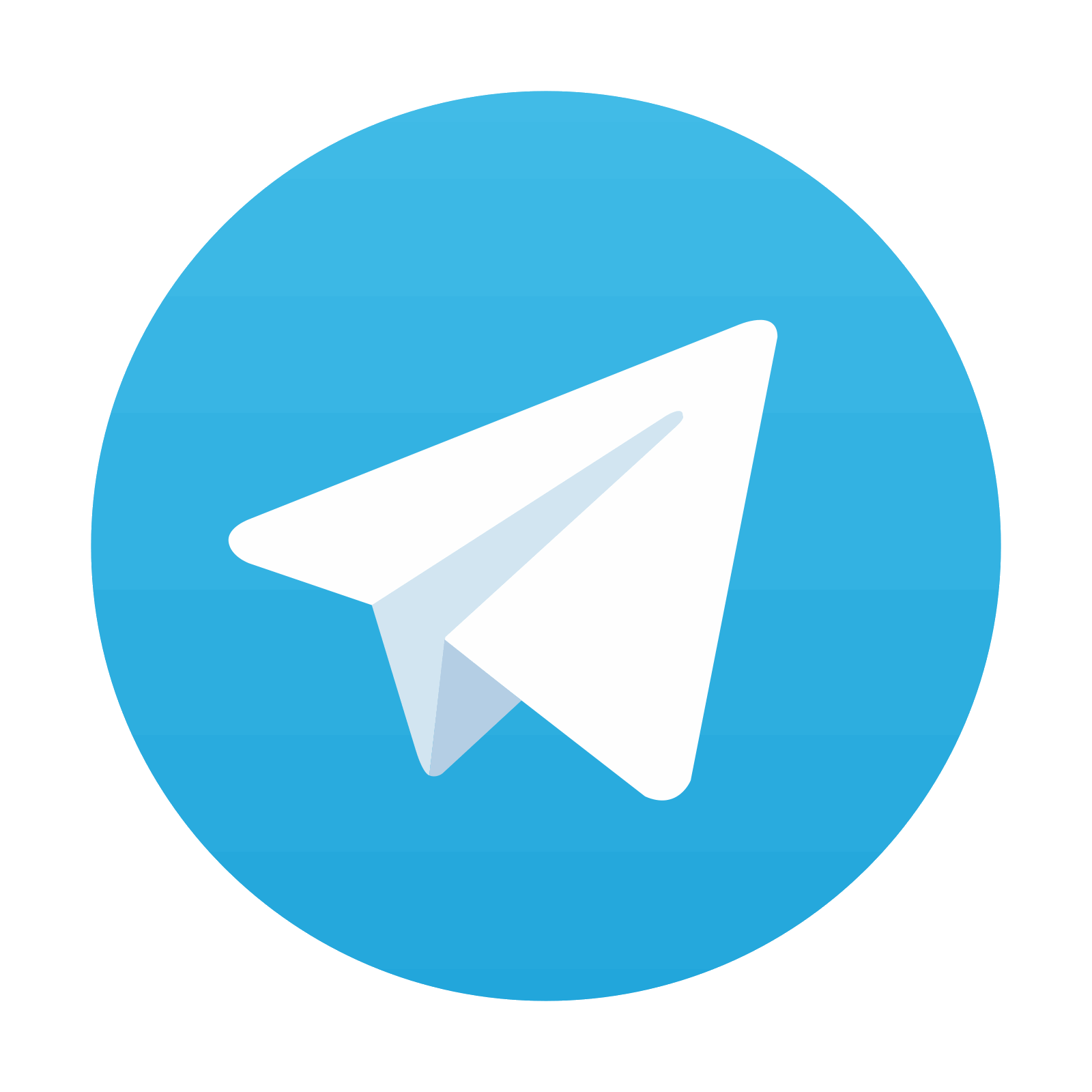
Stay updated, free articles. Join our Telegram channel
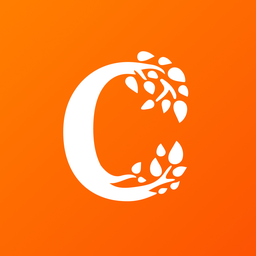
Full access? Get Clinical Tree
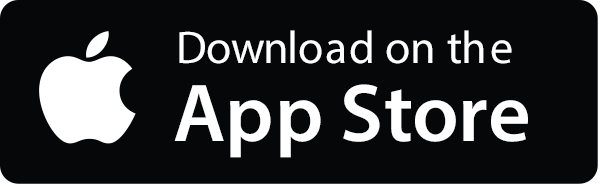
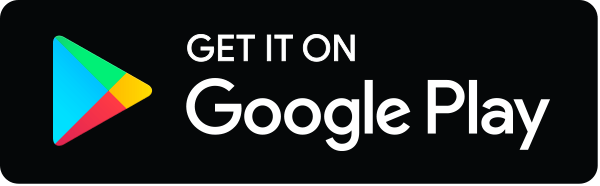