Danika L. Bannasch, Consulting Editor Carrie J. Finno Genetic testing based on deoxyribonucleic acid (DNA) involves the analysis of an animal’s DNA to determine the individual’s genotype for an inherited disorder, trait, or anonymous marker. Genetic testing can be used for positive or negative selection in a population, depending on whether it is being used to identify a disease (negative) or a trait (positive). Genetic testing can also be used for permanent individual identification and parentage determination. Many breed registries require parentage verification to ensure the accuracy of their pedigrees. Using genetic testing results for selection requires an understanding of the mode of inheritance of the disease or trait. Most often, a genetic test will be performed for a recessive disorder to determine if an animal is a carrier. Carriers are asymptomatic but have the potential to produce diseased progeny. Because they have no outward manifestation of disease, a genetic test is extremely valuable for managing their breeding appropriately. Carrier animals can be bred to noncarriers if needed to retain valuable characteristics while not producing diseased offspring. In the case of positive selection for a trait of interest, carrier animals may have higher breeding values because they can produce a trait if bred to other carriers or to animals with the trait. Genetic tests may also be used for dominant disorders if the disease/trait has a late age of onset or if it is inherited in a co-dominant manner. DNA testing for traits that are controlled by more than one locus (polygenic) may also be used for selection for economically important traits. In these cases, one particular genotype may confer a slight advantage over another and therefore, in a large population, can have a significant effect on production. Box 52-1 defines key genetic terms; see also Chapter 51. Researchers use genetic markers distributed along all the chromosomes as tools to identify regions associated with diseases or traits. The markers are composed of small nucleotide repeats and are called microsatellite markers or short tandem repeats (STRs). These markers have a feature that makes them extremely useful to geneticists; the markers have been chosen to be “polymorphic” (show differences) between individuals. In other words, individual animals will have different lengths of the nucleotide repeats for each of these markers. The high level of polymorphism of this type of marker makes them useful for “mapping” (identifying the chromosomal location of diseases and traits). The microsatellite markers are assayed by polymerase chain reaction (PCR) amplification using fluorescent-labeled primers. Primers are short (20 base pairs), single-strand lengths of DNA that are complementary to a specific region of the genome. PCR is the amplification of a section of DNA contained between two primers designed to complement the unique sequence flanking the STR. The PCR products are then resolved by electrophoresis based on their length. Figure 52-1 shows a single microsatellite marker in three different animals. This marker is polymorphic and would be a useful marker for individual identification or parentage. Because the markers show differences between individuals, a collection of these markers can be used as a form of identification of an animal. High statistical significance can be obtained with as few as 10 markers, depending on the species and breed. The DNA type of an animal will not change over its lifetime and can therefore be used as a form of permanent identification. Many purebred registries require parentage verification for registration purposes. To accomplish parentage verification, a DNA sample must be available from both parents as well as the offspring. DNA samples are taken in the form of hair, blood, or buccal swabs (depending on the species and registry) and submitted at the time registration is requested. Each animal inherits one copy of each marker from its sire and one copy from its dam, so the markers can also be used to verify parentage. The most useful marker has a high polymorphism rate because that type of marker will be most likely to show differences not only between the sire and the dam, but also between the two copies (alleles) of the marker. A set of polymorphic markers (10 to 20) is used to verify parentage to ensure a high probability that the parentage is correct. Table 52-1 shows the allele sizes for a set of markers in a parentage case. For marker A the offspring inherited a 122 and a 126 allele. The 122 came from its dam, so the 126 came from the sire. Because sire 1 does not have a 126 allele, it has been excluded. In this example, sire 1 is excluded as the sire of the offspring, and sire 2 is verified on the basis of the results for all three markers. Early attempts to construct whole-genome maps of large animal species were based on two technologies that had been used to construct the first human genome maps: somatic cell genetics and in situ hybridization. This technology involved using labeled complementary DNA or RNA strands (i.e., probes) to localize a specific DNA or RNA sequence in somatic cells. Initial mapped markers were generally genes, or gene products, highly conserved across mammalian genomes. These markers, defined as syntenic (genes on the same chromosome but not necessarily linked) and cytogenic locations, were determined through sequences hybridizing specific DNA probes. Therefore, the first “genome maps” were defined as synteny groups: genes on the same chromosome defined by gene products that segregated in hybrid somatic cell lines. Synteny groups were developed for cattle, horses, and sheep. The next stage of mapping required the use of highly polymorphic markers, including microsatellites, within families of animals to create linkage maps. Collaborative efforts between researchers interested in large animal genomics were required to create these linkage maps for cattle, horses, sheep, and goats (Table 52-2). Radiation hybrid (RH) maps, which used x-ray breakage of chromosomes to determine the distances between DNA markers, followed. These RH maps allowed for the incorporation of genetic (i.e., linkage) and physical maps. The first RH maps involved only specific chromosomes, but later generations included whole genome RH and comparative maps. The isolation of bacterial artificial chromosome (BAC) clones for a large assortment of loci in the various large animal species was instrumental in facilitating chromosomal locations for these loci. In the horse, a complete physical BAC contig map of the entire genome was developed. TABLE 52-2 Map Status and Genomic Resource for Large Animal Species * September 2007 2.0 draft assembly Equus caballus, available from http://www.ncbi.nlm.nih.gov/genome/145. † October 2011 4.6.1 draft assembly Bos tauru, available from http://www.ncbi.nlm.nih.gov/genome/82. ‡ February 2010 1.0 draft assembly Ovis aries, available from http://www.ncbi.nlm.nih.gov/genome/83. § December 2012 1.0 draft assembly Capra hircus, available from http://www.ncbi.nlm.nih.gov/genome/10731. ‖ Illumina, Inc., San Diego, Calif. ¶ Agilent Technologies, Inc., Wilmington, Del. # Affymetrix, Inc., Santa Clara, Calif. Whole genome sequencing and genome assembly of large animal species was completed in the early 2000s (horse, September 2007; cow, October 2011; sheep, August 2012; goat, December 2012). In the horse, a Thoroughbred mare (Twilight) was selected for sequencing because of her low heterozygosity rate (1/1380 base pairs). In 2010, a Quarter Horse mare underwent whole genome sequencing and was aligned to Twilight.1 In cattle, a single partially inbred Hereford cow was selected to contribute 6x whole-genome shotgun (WGS) reads and another 1.5x came from individual animals of the Holstein, Angus, Jersey, Limousin, Brahman, and Norwegian Red breeds for detection of single nucleotide polymorphisms (SNPs). Following sequencing of each species, over 20,000 protein-coding genes were annotated on the sequences by virtue of previously sequenced cDNAs as well as by prediction software that compare known genome sequences in other species with newly sequenced genomes. These annotated genome databases are publicly available (see Table 52-2). With the advent of whole genome sequencing and assemblies, there has been a rapid expansion in the number of SNPs discovered in the genomes of large animal species. SNPs are now considered the next generation of markers to conduct breed diversity and to map disease-causing traits. The availability of such a tool as an SNP-chip facilitates rapid mapping of diseases to specific chromosomal regions and analysis of candidate genes. SNP arrays are currently available on many large animal species (see Table 52-2) and are currently being used in mapping studies of various complex disorders. Haplotype structures are being identified across species with targeted resequencing and, most recently, whole genome next-generation sequencing, of animals from different breeds. In addition to genomic mapping, functional genomic tools have become more readily available and affordable to researchers. Expression arrays have been designed in the horse, cow, and sheep (see Table 52-2). Most recently, sequencing of the transcriptome, through technologies such as RNA sequencing (RNA-seq), has allowed researchers to evaluate gene expression differences among tissues and between animals of different disease states. Initial genetic mutations in large animal species were discovered through the use of comparative genomics. Genes involved in a specific disease were targeted because of equivalent diseases in other species, namely humans. In the horse (Table 52-3), the genetic mutations for many diseases that have genetic tests currently available, including hyperkalemic periodic paralysis (HYPP)2 and severe combined immunodeficiency (SCID),3 were uncovered by evaluating candidate genes that had been associated with similar diseases in humans. With the sequencing and annotation of whole genome maps, other diseases were discovered through whole genome linkage mapping (hereditary equine regional dermal asthenia [HERDA]4), genome-wide association studies with microsatellites (type I polysaccharide storage myopathy [PSSM]5) and genome-wide association studies using SNP array technology (Lavender foal syndrome6). TABLE 52-3 Genetic Tests for Horses A similar theme is evident in cattle. Initial genetic mutations were discovered based on sequencing of candidate genes known to cause similar disease in humans (bovine leukocyte adhesion deficiency [BLAD]7). Later studies used microsatellite markers and performed linkage analysis (complex vertebral malformation8) and, most recently, the use of SNP-based genome-wide association studies has identified recessive defects (congenital muscular dystony types 1 and 29). At the time of publication, there are 90 genetic tests available in cattle (Table 52-4). It is worth noting that the majority of genetic tests currently available are for diseases/traits that are inherited as autosomal recessive traits. With the current technologies available through SNP-association mapping and next-generation sequencing, we should expect to further our understanding of polygenic traits and diseases. TABLE 52-4 Genetic Tests for Cattle
Genetic Tests for Large Animals
Individual Identification and Parentage Testing
Genome Maps
Horse
Cattle
Sheep
Goat
Chromosome (2n)
64
60
54
60
Linkage map
Yes
Yes
Yes
Yes
Cytogenic map
Yes
Yes
Yes
Yes
RH map
Yes
Yes
Yes
No
Whole genome sequencing
6x*
7x†
3x‡
12X§
SNP-chip available
Yes: 76,000 SNPs‖
Yes: 778,000 SNPs‖
Yes: over 50,000 SNPs‖
Yes: over 50,000 SNPs
Expression array available
Yes¶
Yes#
Yes¶
No
Identifying Genetic Mutations
Disease/Trait
Gene
Mode of Inheritance
Reference(s)
Androgen insensitivity syndrome (AIS)
AR
X-linked recessive
18
Cerebellar abiotrophy (CA)
TOE1/MUTYH
Autosomal recessive
19
Hereditary regional dermal asthenia (HERDA)
PPIB
Autosomal recessive
4
Junctional epidermolysis bullosa (JEB)
LAMA3
Autosomal recessive
20
Junctional epidermolysis bullosa (JEB)
LAMC2
Autosomal recessive
21
Foal immunodeficiency syndrome (Immunodeficiency of Fell ponies)
SLC5A3
Autosomal recessive
22
Gaitedness
DMRT3
Multifactorial
23
Glycogen storage disease IV (Glycogen branching enzyme deficiency)
GBE1
Autosomal recessive
24
Lavender foal syndrome (LFS)
MYO5A
Autosomal recessive
6
Malignant hyperthermia (MH)
RYR1
Autosomal dominant
25
Megacolon (Ileocolonic aganglionosis or lethal white foal syndrome)
EDNRB3
Autosomal semi-dominant
10–12
Multiple ocular defects
PMEL17
Autosomal semi-dominant
26
Myotonia
CLCN1
Autosomal recessive
27
Night blindness, congenital stationary
TRPM1
Autosomal recessive
28
Ovotesticular disorder of sexual development
SRY
Y-linked
29
Periodic paralysis II (hyperkalemic periodic paralysis; HYPP)
SCN4A
Autosomal semi-dominant
2
Polysaccharide storage myopathy type I (PSSM I)
GYS1
Autosomal dominant
5
Severe combined immunodeficiency (SCID)
DNAPKcs
Autosomal recessive
3
Thrombasthenia (Glanzmann thrombasthenia)
ITGA2B
Autosomal recessive
30, 31
Disease/Trait
Gene
Mode of Inheritance
Reference(s)
Disease
Abortion/Stillbirth
MIMT1
Maternally imprinted
32
Abortion
APAF1
Autosomal recessive lethal
33
GART
Autosomal recessive lethal
34
CWC15
Autosomal recessive lethal
35
SHBG
Autosomal recessive lethal
34
SLC37A2
Autosomal recessive lethal
34
Acrodermatitis enteropathica (bovine hereditary zinc deficiency or lethal trait A46)
SLC39A4
Autosomal recessive
36
Anhidrotic ectodermal dysplasia
EDA
X-linked recessive
37
Arachnomelia (spider limbs)
MOCS1
Autosomal recessive
38
SUOX
Autosomal recessive
39
Axonopathy (Demetz syndrome)
MFN2
Autosomal recessive
40
Beta-lactoglobulin, aberrant low expression
PAEP
Autosomal
41
Brachyspina
FANCI
Autosomal recessive
42
Cardiomyopathy and woolly haircoat syndrome
PPP1R13L
Autosomal recessive
43
Cardiomyopathy, dilated
OPA3
Autosomal recessive
44
Chediak-Higashi syndrome
LYST
Autosomal recessive
45
Chondrodysplasia
EVC2
Autosomal
46
Citrullinemia
ASS1
Recessive
47
Complex vertebral malformation
SLC35A3
Autosomal recessive
8
Congenital muscular dystonia 1
ATP2A1
Autosomal recessive
9
Congenital muscular dystonia 2
SCL6A5
Autosomal recessive
9
Deficiency of uridine monophosphate synthase (DUMPS)
UMPS
Autosomal recessive
48
Dominant white with bilateral deafness
MITF
Autosomal dominant
49
Dwarfism, Angus
PRKG2
Autosomal recessive
50
Dwarfism, Dexter
ACAN
Autosomal recessive lethal
51
Dwarfism, growth-hormone deficiency
GH1
Autosomal recessive
52
Ehlers-Danlos syndrome, Holstein variant
EPYC
Autosomal recessive
53
Ehlers-Danlos syndrome, type VII (Dermatosparaxis)
ADAMTS2
Autosomal
54
Epidermolysis bullosa
KRT5
Autosomal dominant
55
Epidermolysis bullosa, dystrophic
COL7A1
Autosomal recessive
56
Factor XI deficiency
F11
Autosomal recessive
57
Forelimb-girdle muscle anomaly
GFRA1
Autosomal recessive
58
Glycogen storage disease II (Pompe disease)
GAA
Recessive
59
Glycogen storage disease V
PYGM
Autosomal recessive
60
Goiter, familial
TG
Autosomal
61
Hemophilia A
F8
X-linked
62
Hypotrichosis
HEPHL1
Autosomal recessive
63
Ichthyosis congenita
ABCA12
Autosomal recessive
9
Lethal multi-organ developmental dysplasia
KDM2B
Autosomal recessive
64
Leukocyte adhesion deficiency, type I
ITGB2
Autosomal recessive
7
Mannosidosis
Alpha
MAN2B1
Autosomal recessive
65
Beta
MANBA
Autosomal recessive
66
Maple syrup urine disease
BCKDHA
Autosomal recessive
67
Marfan syndrome
FBN1
Autosomal dominant
68
Mucopolysaccharidosis IIIB
NAGLU
Autosomal recessive
69
Multiple ocular defects
WFDC1
Autosomal recessive
70
Muscular hypertrophy (double muscling)
MSTN
Autosomal recessive
13–15
Myasthenic syndrome, congenital
CHRNE
Autosomal
71
Myoclonus
GLRA1
Autosomal recessive
72
Myopathy of the diaphragmatic muscles
HSPA1A
Autosomal recessive
73
Neuronal ceroid lipofuscinosis 5
CLN5
Autosomal recessive
74
Osteopetrosis
SLC4A2
Autosomal recessive
75
Ovotesticular disorder of sexual development
SRY
Y-linked
76
Polled and multisystemic syndrome
ZEB2
Autosomal dominant
77
Protoporphyria
FECH
Autosomal
78
Pseudomyotonia, congenital
ATP2A1
Autosomal recessive
79
Renal dysplasia
CLDN16
Autosomal recessive
80
Scurs, type 2
TWIST1
Autosomal dominant
81
Spherocytosis
SLC4A1
Autosomal incompletely dominant
82
Spinal dysmyelination
SPAST
Autosomal recessive
83
Spinal muscular atrophy
KDSR
Autosomal recessive
84
Syndactyly
LRP4
Autosomal recessive
85, 86
Tail, crooked
MRC2
Autosomal recessive
87
Thrombopathia
RASGRP2
Unknown
88
Trimethylaminuria
FMO3
Autosomal recessive
89
Yellow fat
BCO2
Unknown
90
Trait
Milk yield and composition
GHR
Polygenic
91
DGAT1
Polygenic
92
Leptin
Polygenic
93, 94
Meat tenderness
Calpastatin
Polygenic
95, 96
µ-Calpain
Polygenic
97, 98 Stay updated, free articles. Join our Telegram channel
Full access? Get Clinical Tree
Genetic Tests for Large Animals
Chapter 52
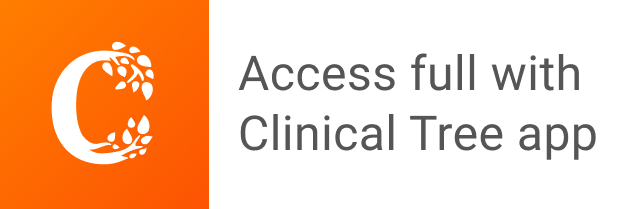