1 Gastrointestinal physiology
This chapter is directed primarily at the normal function of the gastrointestinal tract (GIT) of the prototype 500 kg adult horse. Readers interested in the GIT of foals are advised to consult the various available texts on equine neonatology. The authors have focused on information that is specifically available for the horse and data applicable to other species is only presented to make a point of comparison or contrast. This chapter therefore differs in some aspects from previous reviews on this topic which inferred that certain mechanisms have been shown to be present in horses when, in fact, they are not. This chapter considers the different anatomical regions of the GIT in sequence (i.e. mouth, esophagus, stomach, small intestine, and large intestine). It must be remembered from the outset that the horse is a classic “hind gut fermenter” with a particularly large cecum and complex large colon structure in which digestion of plant fiber occurs (Fig. 1.1).
Enteric Nervous System
In mammals, all GIT function is constantly monitored and modified by the highly complex enteric nervous system (ENS), commonly referred to as the “brain of the gut”. It functions both on an independent local level and in conjunction with modulatory input from the central nervous system (CNS) via the vagus nerve (parasympathetic) and the sympathetic ganglia. Essentially, the ENS itself is made up of intrinsic afferent neurons, ascending and descending interneurons and motor neurons. At least twenty different subtypes of these motor neurons have been identified (Furness 2006, 2008, Goyal & Hirano 1996).
Concerning GI motility, virtually all of the parasympathetic (vagal) input into the gastro intestinal tract (GIT) is processed through the ENS, with acetylcholine (ACh) playing a predominant role in intra-neuronal communication (Berthoud 2006, Furness 2006, Goyal & Hirano 1996). Parasympathetic input is stimulatory whereas sympathetic (adrenergic) input, with respect to controlling motility, is inhibitory. The majority of the sympathetic input is directly from the sympathetic ganglia, and mediated at the neuromuscular junction by norepinephrine (NE). There is a smaller component of the sympathetic input to the ENS that down-regulates ENS-mediated ACh stimulation via intrinsic -adrenergic pathways (Scheibner et al 2002).
Synaptic transmission, paracrine signaling and hormonal signaling are forms of chemical information transfer within the ENS. Motor neurons that stimulate muscle contraction may express, in addition to ACh, either substance P (SP) or the peptide motilin at the neuromuscular junction. Those neurons from the ENS that inhibit contraction can express a variety of neurotransmitters including nitric oxide (NO), vasoactive intestinal peptide (VIP) or adenosine triphosphate (ATP). These three neurotransmitters, along with SP, comprise the non-adrenergic, non-cholinergic (NANC) component of the ENS (Burnstock 2009, Goyal & Hirano 1996, Kunz & Furness 1999). Calcitonin gene-regulated peptide (CGRP) and numerous subtypes (e.g. 5-HT1, 5-HT2, 5-HT3, etc.) of serotonin (5-HT) act within the ENS to either up-regulate or down-regulate the activity (Berthoud 2006, Goyal & Hirano 1996, Kunz & Furness 1999). Various receptors within the GIT mucosa that constantly monitor the wall tension and the physicochemical characteristics of the digesta evoke either stimulatory or inhibitory responses via the ENS intrinsic afferent neurons; again, CGRP and 5-HT are important mediators in this process (Braun et al 2007, Cooke 1986, Holzer et al 2001, Lundgren 2004 Schemann & Mazzuoli 2010) (Fig. 1.2).
In horses, all of the mentioned neurotransmitters have been found, with varying degrees of expression depending upon the segment of the GIT (Ceccarelli et al 1995, Chiochetti et al 2009, Domenghini et al 2004, Fink et al 2006, Freytag et al 2008, Kotz & Van Aswegan 1990, Malone et al 1999, 2000, Merritt et al 1998, Pearson 1994; Rakestraw et al 1996, Rutkowski et al 1998, Sasaki & Yoshihara 1998, Sasaki et al 2000, Sellers et al 1984b, Solinger & Sonea 2008). There may be some equine species specificity with respect to predominance of 5-HT subtype, which has important implications concerning the development of drugs to control GIT function in the horse, but this still needs further clarification (Delesalle et al 2008, Lippold et al 2004, Nieto et al 2000, Prause et al 2009).
In all species, secretory epithelia, endocrine cells and vasculature within the GIT are also under ENS control. Neurotransmitters involved, in addition to those mentioned above, include numerous well-known peptides such as cholecystokinin (CCK), somatostatin (SST), gastrin-releasing peptide (GRP), neuropeptide Y (NPY) and various opioids (Berthoud 2006, Cooke 1986, Furness 2006, Wood & Galligan 2004). Finally, orexin A, a neurotransmitter found to be involved in appetite control in rats, has also recently been found within the ENS of the horse (Dall’Aglio et al 2009).
Mouth
The horse has three main salivary glands, parotid, mandibular (submandibular), and sublingual, named according to their anatomical location. The parotid is the largest and most peripheral, the dorsal end being just in front of the ear and the ventral end being just behind the caudoventral margin of the ramus of the mandible. The mandibular gland lies underneath the ventral portion of the parotid gland and extends from the atlantal fossa to the hyoid bone. The sublingual gland, which is the smallest, is located just under the oral mucous membrane between the body of the tongue and mandible (Sisson & Grossman 1959).
Secretion
Equine saliva is >99% water (Alexander, 1966). In general it contains relatively more calcium and chloride, and less bicarbonate and sodium, than that of ruminants and is thus more similar in composition to that found in carnivora and omnivora (Alexander & Hickson 1970, Stick et al 1981). Resting electrolyte concentrations in parotid saliva, which is hypotonic to plasma, are listed in Table 1-1. Concentrations of Na+, Cl− and HCO3− increase in a linear relationship to the rate of secretion. Adult horses may secrete up to 35–40 liters/day with a pH of 8.6–9.1, the majority originating from the parotid (Meyer et al 1985, Moeller et al 2008, Stick et al 1981). As in other species, the rate of secretion in the horse is stimulated by food intake and mastication. The greater the dry matter within the food, the greater the amount of saliva secreted due in part to the physical composition of the meal and in part to the time needed for adequate mastication, with the latter being the major determinant (Meyer et al 1985, 1986). In one study using esophagofistulated horses, the DM content of swallowed material varied between 11–15% during intake of roughage and increased to 21–34% after intake of concentrates (Meyer et al 1986) (Table 1-2).
Finally, in contrast to carnivora and omnivora, equine saliva contains virtually no digestive enzymes: as an example, the average concentration of amylase was reported to be 0.44 U/ml in horses vs. the average data 77 U/ml in humans and 98 U/ml in swine (Varloud 2006). Therefore, its most important digestive functions appear to be the lubrication of swallowed ingesta and the buffering of gastric contents which would promote intra-gastric bacterial fermentative activity (see Stomach).
Host source enzymatic digestion
The mean value of α-amylase activity in equine saliva was reported to be very low as stated earlier. This concentration is considered to be insufficient, when linked to the extent of any mastication, to digest starch (Varloud 2006). There are no specific data in horses regarding the activity levels and properties of salivary lysozyme. Lysozyme is an antibacterial enzyme found in human saliva with activity against gram-positive bacteria.
Digestive ecosystem and digestion/fermentation
Saliva contains less than 10 cfu total bacteria/ml (Varloud 2006). The contribution of these bacteria to digestive processes is typically ignored. Lindner and his collaborators measured salivary lactate concentrations in exercising horses in an attempt to find a means to monitor lactate production during exercise without having to take blood samples. After continuous exercise, the average concentration sampled was 2.21 ± 1.97 mmol/l saliva at the upper site of sampling, close to the opening of the parotid duct (Lindner et al 1999). Considering the potential production 40 l of saliva per day, this could represent up to 160 mmol of lactate flowing into the stomach and helping to acidify the contents.
Motility
Anyone who has watched a horse eat is left with the impression that it chews its food quite thoroughly, which reduces the particle size of the ingesta and presumably provides a higher digestion from both enzymatic and microbial enzymes (Ellis & Hill 2005). In fact, however, horses may actually chew their food less thoroughly than do ruminants, based upon minutes of chewing per kg of DM of food ingested (Dulphy et al 1997). Bonin et al (2007) describe three phases of the equine chewing cycle: opening, closing, and power stroke. During the opening phase there is a downward hinge movement combined with a rolling motion around the rostrocaudal axis that separates upper and lower dental arcades on the chewing side. At the same time a yaw motion around the dorsoventral axis swivels the mandible away from the chewing side. During the closing phase a small amount of roll brings the upper and lower arcades into apposition on the chewing side, while the yaw swivels the mandible across the midline. For the power stroke, the lower dental arcade slides across the upper arcade in a lateral to medial direction. In their study where horses were fed either hay or pellets in random order, Bonin et al. found that the chewing cycle duration was significantly longer, and thus chewing frequency reduced, during intake of hay vs. pellets (Bonin et al 2007). Meyer et al report that after a typical mastication of a roughage meal, the particle size of about one-half of the contents of the swallowed bolus is <1.6 mm (Meyer et al 1985). It is presumed by many that teeth in poor condition or any other problem that may interfere with normal mastication can cause digestive disturbances and/or chronic weight loss in horses, but the validity of this association has been questioned (Ralston et al 2001, Ellis and Hill 2005, Carmalt and Allen 2006). However, Meyer et al. (1985) have shown that chewing releases a notable portion of the soluble components within ingested roughage, a function that could perhaps be reduced when there is malocclusion (Meyer et al 1993). After mastication food is moved into the pharynx where the pattern-elicited swallowing action occurs.
Key Points
• Equine saliva is ~99% water and is relatively high in Ca and Cl content compared to other species. It contains only a trace of amylase activity thus it serves primarily as a lubricant for ingested food.
• Horses secrete large amounts of saliva. The secretion increases in response to DM food intake and chewing duration. In general, forage intake, which requires more chewing, causes a larger volume of salivary secretion than does concentrate intake.
• Three phases of the equine chewing cycle have been described: opening phase, closing phase and power stroke. Chewing not only breaks ingested fiber down into smaller particles for optimal digestion, within the large intestine, but it also releases soluble nutrient components within the fiber which can be digested pre-cecally.
Esophagus
The esophagus is 1.2–1.5 meters long in the adult 500 kg horse. It is anatomically divided into cervical, thoracic and abdominal parts (Nickel et al 1979). It has a sphincter at either end, the upper (UES) marking the division between it and the pharynx, and the lower (LES) between it and the stomach. The musculature of the proximal two thirds of the equine esophagus is striated, while the distal third is smooth. It is, as in all mammalian species, lined with a modified stratified squamous epithelium. This mucosa has no significant secretory activity (Slocombe et al 1982).
Motility
When a swallow is induced by the presence of food in the caudal pharynx, both sensory and motor components of which are via the vagus nerve (Meyer 2009), the upper UES relaxes to allow the bolus of food to enter the esophageal lumen. The bolus is passed through the esophagus by classical peristaltic (propagating ring of contraction) motor activity. Contractions through the striated muscle region are of shorter duration and propagate more rapidly than those through the smooth muscle region (Clark et al 1987, Ruckebusch et al 1981, Stick et al 1983). In humans, the peristalsis within the striated muscle is centrally controlled solely by vagal afferent nerves whereas the smooth muscle is innervated by intramural inhibitory (nitric oxide releasing) and excitatory (ACh releasing) neurons that receive inputs from separate sets of preganglionic neurons located in the dorsal motor nucleus of the vagus (Goyal and Chadbury 2008). Once the bolus reaches the distal end of the esophagus, the LES relaxes, allowing the contents to enter the stomach. The rate at which swallowed material traverses the total length varies, according to physical content, but typically takes between 4 and 10 seconds (Greet 1982), with liquid passing more rapidly (Fig. 1.3).
Stomach
The volume of the equine stomach is about 8% of the total GIT; thus, in the adult 500 kg horse it is 8–15 liters (Nickel et al 1979). The proximal one-half of the stomach is lined with a modified stratified squamous epithelium essentially similar to that of the esophagus and contains no secretory glands. The esophagus opens into this part. Squamous and glandular portions are well demarcated by the margo plicatus. The glandular mucosa is divided into cardiac, fundic and pyloric portions. The cardiac region comprises only a thin strip right adjacent to the margo plicatus; little is known about its secretory products. The fundic mucosa contains parietal cells which secrete acid, zymogen cells which secrete pepsin and lipase, and enterochromaffin-like (ECL) cells that secrete histamine in response to gastrin and vagal stimulation. The pyloric mucosal region is the site of G-cells which synthesize gastrin. D-cells, which synthesize somatostatin, are found in both fundic and pyloric mucosas (Hersey & Sachs 1995, Modlin & Tang 1996, Schubert and Peura 2008, Vuyyuru L et al 1995). The pyloric region, also known as the gastric antrum, is connected to the small intestine, with the point of delineation being the pyloric sphincter of the stomach.
Secretion
The major secretory product of the stomach in the horse, as in all species, is hydrochloric acid (HCl) (Andrews et al 1992, Baker & Gerring 1993, Campbell-Thompson & Merritt 1987, 1990, Murray & Schusser 1993, Sangiah et al 1988). As indicated in the anatomical review, HCl is secreted by the parietal cells which are located within the gastric glands of the fundic mucosa. Like any other bodily function, control of secretion involves a complex interaction between stimulatory and inhibitory signals. From the most elemental standpoint, the primary neural component for stimulation is vagally released acetylcholine, and the primary hormonal component is the peptide, gastrin, that is produced by G-cells located within pyloric mucosa. But this simple concept has now become much more complex in light of continuing discoveries. Current evidence suggests that the major stimulatory effect of both acetylcholine and gastrin on the parietal cells is indirect, by inducing ECL cells to release histamine that acts via the H2 receptors, rather than by directly stimulating the muscarinic and gastrin receptors (Modlin & Tang 1996). Acid secretion is modulated by various feedback loops, mainly involving somatostatin, that are, in themselves, responsive to numerous factors, including intra-gastric pH and ingesta composition (Kidd et al 1996, Modlin & Tang 1996, Schubert and Peura 2008, Vuyyuru et al 1995) (Fig. 1.4).
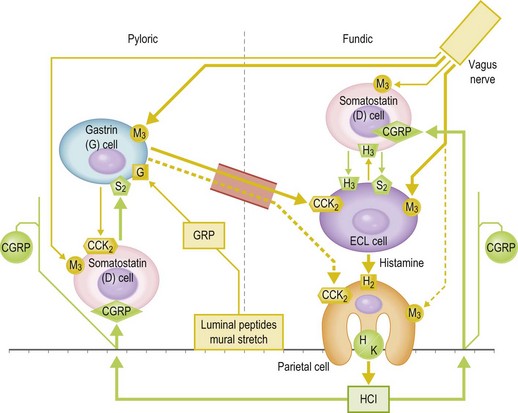
Figure 1.4 Diagrammatic representation of the major components of the control of gastric acid secretion.
In the equine, Olowo-okorun was the first to demonstrate the presence of gastrin in pyloric mucosa in 1975 (Olowo-okorun 1975). In 1990, Young and Smythe confirmed this and suggested that the posttranslational G-cell processing of progastrin in horses may differ from that of species previously studied (Young & Smythe 1990). This suggestion was confirmed by Johnsen et al (1998) who found that, in contrast to other species, equine antral gastrins are virtually non-sulfated due to low tyrosyl sulfotransferase activity within the G-cells. This may explain why plasma gastrin in the horse is proportionally high in the “large” (G-34) vs the “small” (G-17) form, but the implications of this with respect to endogenous gastrin activity remain to be elucidated.
Horses, like pigs, monkeys, rats and humans, and in contrast to carnivora, continue to secrete HCl acid at a variable rate even when the stomach is empty (Andrews et al 1992, Campbell-Thompson & Merritt 1987, 1990, Merritt 1999, Merritt et al 2003, Murray & Schusser 1993, Orsini et al 1991, Sangiah et al 1988). This is commonly referred to as “basal” secretion and while it is reasonable to assume that a truly empty stomach is not a normal physiological condition in the horse, it is of comparative interest. Under this contrived condition, healthy horses have been found to secrete ~200 µeq/kg/hr of HCl (Campbell-Thompson & Merritt 1990) which is mixed with varying amounts of pancreatic and duodenal fluid that has refluxed into the stomach from the upper small intestine (Kitchen et al 2000). Whether or not such reflux occurs during fed conditions remains to be determined. Also, as in all mammalian species, acid secretion in the horse is stimulated by acetylcholine (Campbell-Thompson 1994), histamine (Kitchen et al 1998) and gastrin (Campbell-Thompson & Merritt 1990), and inhibited by somatostatin (Sojka et al 1992). However, the relative responses to these agents show some species-specific characteristics. For example, in vitro studies of isolated equine parietal cells conducted by Campbell-Thompson indicated that equine parietal cells are much more sensitive to histamine than to gastrin, whilst just the opposite is the case for canine parietal cells (Campbell-Thompson 1994). Two studies have specifically looked at the effects of food intake on plasma gastrin concentration in adult horses. Brown et al recorded a significant increase to ~2.5 times the fasting value within 15 minutes of ingestion of a pelleted meal of unstated amount. This increase was present until at least 75 minutes after feeding (Brown et al 1987). Sandin et al found there was an immediate and large increase in response to a large meal, whereas a small meal evoked a later and smaller response. They suggested that the degree of gastric distention may play a role in this discrepancy. Furthermore, grain meals evoked a slower and more prolonged response than hay meals (Sandin et al 1998). The obvious implication from these studies is that size and composition of a meal are important determinants of the amount of acid secreted, although the specific amount is very difficult to measure when food is present within the stomach.
In most monogastric animals, including the neonatal foal, the gastric contents are quite acidic throughout because of the uniform semiliquid to liquid consistency of the ingesta. In contrast, the contents of an adult equid on a regular hay/grain diet, where the roughage is available on a free-choice basis, vary in their pH depending upon where they are situated within the stomach. It is the roughage component of the diet that determines this since, as in the rumen, the lower density/larger particle size components tend to remain at the top of the mat of ingesta where they are minimally exposed to acid produced in the lower glandular region and maximally exposed to swallowed saliva which has a pH of ~7.5 (Stick et al 1981).
Thus, during the time when roughage intake is the highest in horses allowed free choice intake (Dulphy et al 1997, Houpt 1998), the mean pH of the contents just inside the lower esophageal sphincter vacillates between 5–7 over time, whereas the higher density, more liquid contents found in the bottom part of the stomach are consistently acidic at pH 2.0–3.0 (Husted et al 2008, Lorenzo-Figueras & Merritt 2002, Merritt 2003). When roughage intake is decreased either because it is withheld or because horses eat less during the early morning hours even if it is available, the mean pH in the upper part of the stomach drops markedly to 4.0 or less (Husted et al 2008, 2009) (Fig. 1.5). Furthermore, the ingesta within the stomach of horses fed pellets in the morning meal and no hay has been shown to coalesce immediately into a bowl shape remaining a couple of hours within the glandular region (Varloud et al 2007) where the surrounding liquid would be quite acidic.
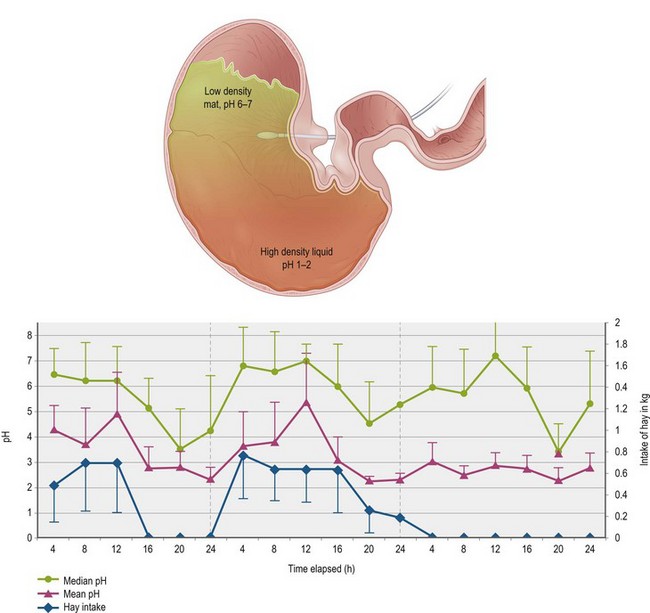
Figure 1.5 The top image represents the pH gradient of contents within the stomach of an adult horse fed forage ad libitum. The higher density, most recently swallowed food at the top maintains a median pH between 6 and 7 due to the fact that it has continuing exposure to saliva and, being in region of the non-glandular mucosa, has not been exposed to acid. The lower density contents and liquid within the lower part of the stomach, near where the acid is secreted, maintain a pH between 1–2.The graph at the bottom, published by Husted et al (2009), depicts pH changes within the non-glandular region near the opening of the lower esophageal sphincter (LES), as measured every 8 seconds via an in situ electrode shown in red. Three days of recording of median and mean pH and hay intake, broken into 4 hour blocks, are shown. During the first day, the horses, which were stalled, were allowed to eat hay ad libitum for the first 12 hours and it was taken away for the next 12 hours. During the second day, hay was available for the full 24 hours, and during the third day, it was taken away for the full 24 hours. Note that the pH of the region around the electrode trends much lower when hay is not available, thus exposing the non-glandular mucosa to a pH that could be detrimental to its integrity.
This variance in the pH has an important implication with respect to the intensity of intra-gastric fermentation since the organisms involved within the stomach are more numerous and active at the higher pH values (Meyer et al 1980, Varloud et al 2007). Furthermore, when the pH drops below 4.0 within the nonglandular region, that mucosa is challenged and may ulcerate since it does not contain the elaborate mechanisms to protect it against HCl that are found within the glandular mucosa (see discussion of gastric mucosal protection below) (Andrews et al 2006, Widenhouse et al 2002). There is evidence that feeding alfalfa hay may provide some protection against this acid challenge of the nonglandular mucosa, perhaps through buffering effects of its high Ca and protein content (Lybbert et al 2007, Nadeau et al 2000).
The two digestive enzymes of note secreted by the equine stomach are pepsin and lipase, which is in concordance with most other mammalian species. Pepsin is proteolytic in an acid medium. The primary secretion by the zymogen (chief) cells found within fundic and pyloric mucosas is pepsinogen, which is converted to pepsin when the pH of the medium is <4.0. More than one biochemical form of equine pepsinogen exists, but the functional significance of this, with respect to intragastric proteolysis, is currently unknown (Gonchar et al 1984, Khittoo et al 1991, Sayegh et al 1999). Pharmacological suppression of gastric acid secretion with an agent such as omeprazole would be expected to result in depressed pepsin activity but this seems to have negligible effect on GIT function, at least in humans (McCarthy 2010). Gastric lipase is also produced by zymogen cells, primarily within the fundic mucosa. Horses produce a notable amount of gastric lipase which has a pH optimum ranging between pH 4.0 and pH 6.0 and is resistant to exposure at pH conditions as low as 1.5 (Moreau et al 1988), but as with pepsin, nothing is known about its role in the processing of ingesta in this species.
Finally, all regions of glandular gastric mucosa secrete mucus and bicarbonate, which stay close to the respective mucosal surfaces and are a very important component in the protection of these surfaces from destruction by gastric acid (Flemström & Isenberg 2001, Holzer 1998). Thus, under normal conditions, the pH of the medium just adjacent to the glandular mucosal surface is biologically neutral. As is well known, one of the most important inducers of mechanisms directed at protecting the glandular mucosa is prostaglandin-E2 (PGE2) that is up-regulated by cyclo-oxygenase-1 (COX-1) and 2 (COX-2) of the arachidonic acid cycle (Peskar 2001a, b). Both COX-1 and COX-2 are found throughout the equine GIT submucosa, in varying degrees according to region (Morissey et al 2008, Morton et al 2008a, b). Probably one of the most important systems by which PGE2 and other mediators involved in promoting mucosal protection are affected is the so-called “gastric neural emergency system” (Fig. 1.6) (Holzer 1998). This is a component of the ENS within the stomach, which is essentially a reflex arc, the afferent arm of which is constantly sampling the pH at the mucosal surface. A sufficient drop in pH results in the neural reflex up-regulation of PGE2 expression, along with that of other mediators of protection, such as calcitonin gene-related peptide (CGRP) and constitutive nitric oxide synthase (cNOS) which increase mucosal blood flow to enhance mucus and bicarbonate secretion (Holzer 1998, Peskar 2001a) (Fig. 1.6). Another mucosal protective effect of PGE2 is the direct inhibition of acid secretion by the parietal cell (Peskar 2001b, Peskar & Maricic, 1998). In a study done by Cargile et al, daily feeding to ponies of 20 ml/kg of corn oil, which is made up of ~40% of the arachidonic acid precursor linoleic acid, significantly increased PGE2 and reduced gastric acid output, both before and during IV pentagastrin infusion. This indicates that this mechanism is present within the equine stomach (Cargile et al 2004). Additional evidence of the importance of PGE2 to the protection of the equine glandular mucosa is the glandular ulcerogenic effects of nonsteroidal anti-inflammatory drugs (NSAIDs) which have strong antiprostaglandin activity (MacAllister et al 1993, Mackay et al 1983, Meschter et al 1990). There is no evidence, however, that any of the mechanisms discussed above are operative in the non-glandular (squamous) gastric mucosa (Andrews et al 2006, Widenhouse et al 2002). Bullimore et al described the presence of a thin layer of mucus on top of the gastric squamous mucosa but they proposed that its source was the glandular mucosa since they found no mucus-producing cells within the squamous mucosa (Bullimore et al 2001). However, in in vitro experiments Peretich et al. (2009) have described an increased expression of mRNA for sodium-potassium adenosinetriphosphatase (NAKA) within nonglandular mucosa that has been exposed to HCl and VFA. NAKA plays a critical role in maintaining intracellular Na-K homeostasis and could thus play an important role in protection of the non-glandular mucosa. Interestingly, this response was relatively greater in older than in younger horses. This interesting finding requires further study.
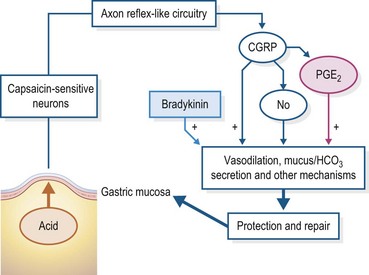
Figure 1.6 A schematic of the “neural emergency system” in the stomach as proposed by Holzer (1998) whereby receptors reside within the gastric glandular mucosa that are sensitive to the presence of gastric acid. Their stimulation induces a neural reflex arc within the gastric component of the ENS that up-regulates mechanisms that protect the mucosa against destruction by the acid.
For instance, CGRP, as depicted in Fig. 1.3, causes somatostatin release which, in turn, down-regulates release of both gastrin and histamine. Nitric oxide (NO) causes submucosal vasodilation which, in turn, makes more HCO3’ available to buffer the acid at the luminal surfaces of the mucosal cells, and PGE2 invokes numerous protective actions such as increased mucus secretion and suppression of acid secretion. This schema is based upon studies done in small laboratory animals but the mediators shown have been found in the equine stomach.
Digestion
Host source enzymatic digestion
In mammals, the conversion of pepsinogen to pepsin occurs within an acidic medium. Despite the relative importance of pepsin’s role in protein digestion in the horse is currently unknown, it probably is similar to other mammals. The endogenous and microbial proteolytic activity has been measured in the gastric content of ponies in both the fundic and pyloric regions. It was negligible in the fundic region (0.14 µg of hydrolyzed protein/mg ingesta/min). Although greater in the pyloric region, it was still ten-fold less (2.43 µg of hydrolyzed protein/mg ingesta/min) than that of the small intestine content (Kern et al 1974).
We do not know the role of gastric lipase in the horse but, presumably, as in humans, it contributes to the partial digestion of feed triacylglycerols (TAG) to form diacylglycerols and free fatty acids (FFA). TAG are mainly composed of long-chain fatty acids (LCFA, number of carbons ≥ 16), plus phospholipids and sterols. The level of lipolytic activity was reported to be the highest in the fundic mucosae compared to the nonglandular, cardiac and pyloric mucosas (87 units per g of fresh tissue versus 8, 4, and 5 respectively). However, this value remained much lower than pancreatic lipase activity: the ratio of total lipase pancreatic/preduodenal activities was 61 (Moreau et al, 1988).
Digestive ecosystem: digestion/fermentation
Intragastric fermentative activity in the horse has been recognized since the early 1940, and in the early 1960s, lactobacilli and streptococci were detected in gastric contents (Alexander & Davies, 1963). However, no protozoa have been found in the stomach (Kern et al 1974). Recent work using culture-dependent and -independent techniques has revealed a diverse bacterial community in the gastric contents and confirmed that the acidic conditions do not prevent a large number of bacteria being present (Al Jassim 2005, Varloud et al 2006). The gastric biotope, in fact, offers a favorable environment for bacterial growth: dietary components that are barely digested prior to introduction into the stomach and are retained, in the most part, for 85–300 min postprandially; water content that varies from 98.9% in fasted animals (Nadeau et al 2000) to 69.3% after a meal (Wolter & Chaabouni, 1979). Temperature is widely recognized as being crucial for managing bacterial cultivation, but very few data are available about this characteristic in the gastric ecosystem. Varloud and collaborators reported temperatures that never exceeded 28°C with an average temperature of 24°C (Varloud et al 2007). No data are available regarding the aerobic–anaerobic status of the gastric environment but it is probable that the redox potential is compatible with the growth of facultative anaerobic bacteria that can tolerate small amounts of O2. As for the intragastric pH, its values fluctuate largely depending on feed ingestion and region within the stomach, as previously described.
In fasting horses, the pH of gastric contents can periodically increase and even exceed neutrality due to duodenal reflux (Campbell-Thompson & Merritt 1990) which is favorable to bacterial colonization. This probably explains why Varloud et al found a large variation of the total anaerobe concentration that from 102 could reach 106 cfu/ml within the fundic glandular region (Varloud et al 2007). Similarly, concentration of total anaerobes were reported to be surprisingly high on the mucosa of non-glandular proventricular (104–106 cfu/ml) and glandular cardiac (105–107 cfu/ml) regions in the empty stomach (Varloud 2006). Despite the large densities of microbial communities, the end-product concentrations were low, confirming that bacterial fermentative activity is weak in the empty stomach (Varloud et al 2007).
In fed horses, saliva is secreted and contributes to increased pH mean values, which can reach up to 7.0 in the proximal stomach (Healy et al 1995, Husted et al 2008, 2009, Lorenzo-Figueras & Merritt, 2002). In one feeding study, from the first postprandial hour the concentrations of bacterial communities increased greatly whereas the inter-individual variability decreased (e.g. pH fluctuations were less pronounced and more homogeneous; Varloud et al 2007). After the first postprandial hour, the gastric microfloral concentration continued increasing with total anaerobe concentration from 9.5 × 106 to 2 × 108 cfu/ml in average at 60 and 210 min post ingestion of the meal respectively (Varloud et al 2007). Even higher values were reported in previous studies (de Fombelle et al 2003, Kern et al 1974). The total anaerobe concentration in the gastric content is equivalent to, or even higher than, that measured in the post-ileal compartments, such as the cecum or the colon (de Fombelle et al 2003). In some recent studies, lactobacilli concentration averaged 1.3 × 107 cfu/ml 210 min after ingestion of the meal (Varloud et al 2007) and from 1.3 × 107–2.5 × 108 cfu/ml 30 min later (de Fombelle et al 2003). As for streptococci, their concentration averaged 1.5 × 106 cfu/ml 210 min after ingestion of the meal (Varloud et al 2007) and varied from 2 × 107–3.2 × 108 cfu/ml thirty min later (de Fombelle et al 2003). The concentration of lactate-utilizing bacteria also increased and averaged 2.5 × 104 cfu/ml 210 min after the distribution of the meal (Varloud et al 2007) and varied from 6.5 × 106–4 × 107 cfu/ml 30 min later (de Fombelle et al 2003). Despite the decrease in intragastric pH when the meal contained more starch, the 240 min postprandial concentrations of the different bacterial communities were similar or even higher (de Fombelle et al 2003).
Merritt has suggested that microbes that convert ingested non-structure carbohydrates to VFA colonize, in particular, the coarser fibrous ingesta, which collects towards the top of the stratified mat of gastric contents. Here the pH of the contents is a more suitable environment for the microbes as the mat has not been fully penetrated by gastric acid (Merritt 2003). The marked increases in bacterial count observed postprandially cannot be explained purely by an influx from the small intestine, and the bacterial concentrations in the feed are too low to cause the effect. As mentioned above, total anaerobe concentration is very high on the mucosa of non-glandular proventricular (105–107 cfu/ml) and glandular cardiac regions (105–107 cfu/ml) in the empty stomach, which suggests that these bacteria are responsible for the inoculation of the ingested bolus. Also, three species of lactobacilli (L. crispatus, L. salivarius and L. reuteri) have been identified on the gastric mucosa (Yuki et al 2000) and within the gastric content (Al Jassim et al 2005, Fujisawa et al 1993), sustaining the hypothesis of an inoculation of the gastric contents by the desquamation of mucosal cells previously colonized by bacteria (Yuki et al 2000).
In the horse stomach, cellulolytic bacterial concentrations have been reported to be negligible (de Fombelle et al 2003, Kern et al 1974). Therefore, the contribution of gastric microflora to plant cell wall degradation is probably insignificant. On the contrary, there is a strong impact of the gastric microflora on starch digestion, due to the high proportion of amylolytic bacteria. Starch is degraded into lactate intragastrically whereas it would provide glucose in the small gut. These two nutrients do not contribute equally to the horse energetic provision. The impact of this starch disappearance in the stomach has not been quantified from a global nutritional point of view.
Varloud et al found that, along with the bacterial densities, the intra-gastric lactate concentration increased linearly from the first postprandial hour and reached 8.0 mmol/L 210 min after the meal distribution (Varloud et al 2007) (Fig. 1.7). Lactate is the major end-product (Argenzio et al 1974b, de Fombelle et al 2003, Kern et al 1974, Varloud et al 2007, Wolter & Chaabouni 1979) and, depending on the studies, L-lactate (Nadeau et al 2000, Varloud et al 2007, Wolter & Chaabouni 1979) or D-lactate (de Fombelle et al 2003, Al Jassim 2006, Varloud et al 2006) represents the major isomer.
In the Varloud et al study, VFA concentration also increased linearly from the first postprandial hour to ~8.0 mmol/l 180 min after meal ingestion (Varloud et al 2007). Argenzio et al reported a maximal VFA concentration 4 h after the meal ingestion (Argenzio et al 1974b). However, others have indicated that the maximal intragastric VFA concentration is reached 1 h postprandially (Nadeau et al 2000). VFA concentrations within the gastric contents have varied from 4.4–51.3 mmol/l (Alexander and Davies 1963, Argenzio et al 1974b, de Fombelle et al 2003, Elsden et al 1946, Kern et al 1974, Morris et al 2002, Varloud et al 2007). Overall, acetate represents the highest molar proportion (70–80%). The second highest is propionate which represents less than 10% of the total VFA.
Varloud et al found that the ammonia concentration increased from 2 h postprandially and reached 3.51 mmol/l 3 h after meal ingestion (Varloud et al 2007) being probably the result of the protein degradation as this contributes to the production of the ionized form of NH3 (Jouany et al 1995). In horses fed a hay-based diet, ammonia concentrations are low postprandially (0.17–0.23 mmol/l) (Kern et al 1974), in contrast to horses fed a starchy concentrate where ammonia can reach concentration levels tenfold higher (Wolter & Chaabouni 1979). As for D-glucose, an end-product of starch hydrolysis, in the Varloud et al study its concentration increased from 14.4 mmol/l to 41.2 mmol/l 3 h after the meal ingestion (Varloud et al 2007), indicating a strong starch-degrading activity of the microbial population.
Motility
There are numerous ways that gastrointestinal motility has been studied and defined. With respect to the horse, these have included: (1) recording myoelectrical activity via electrodes implanted into muscle preparations in vitro, and either surgically implanted onto the serosa or by a trans-abdominal technique in vivo; (2) recording mechanical activity of muscle bath preparations in vitro, or via strain gauges implanted onto the serosa or intra-luminal pressure sensors in vivo; (3) measuring actual transit time by use of various markers; and, (4) undertaking ultrasonographic imaging. The best picture to date of normal GIT motility in the intact, conscious equid has been provided by myoelectrical and mechanical recordings done in numerous laboratories (Hudson & Merritt 2008).
Myoelectrical recordings are perhaps the most complex in terms of the information they can provide. There are essentially two different events that can be seen in GI smooth muscle: (1) the slow wave (SW), a regularly occurring subthreshold oscillation of the resting membrane potential with which there is no associated contraction; and, (2) the action potential (AP), which indicates muscle depolarization and, therefore, contraction. An AP can only occur associated with a SW (Bass et al 1961, Christensen 1971). Therefore, the inherent SW frequency within any given region determines the maximal contraction frequency of the muscle fibers within that region and during periods of time when there are only slow waves present, there is no motility.
The contractile events of the stomach are governed primarily by local myoelectrical activity and neural control through the vagus (Furness 2008). Myoelectrically, the slow wave frequency which, as mentioned above, determines the maximum frequency that the stomach can contract, is around 3/min in the horse and pig, whereas in dogs and ruminants it is closer to 6/min (Kelly et al 1969, Malbert & Ruckebusch 1989, Merritt et al 1989, Minami & McCallum 1984, Ruckebusch & Bueno, 1976). An interesting feature of equine and ruminant gastroduodenal motility is that just prior to the commencement of phase I of the migrating motility complex (MMC) in the proximal duodenum, the gastric antrum stops moving for a few minutes (Merritt et al 1989). During this time gastric emptying ceases which may allow backflow of duodenal contents into the stomach (see section on small intestinal motility for explanation of the MMC).
From the limited number of studies that have been done to date, it appears that equine gastric motility that results in emptying, per se, is not fundamentally different from that of other species. That is, the coarser contents are moved out primarily by peristaltic contractions that start at mid-fundic level and move through the pyloric region with increasing rate and strength (“antral systole”) to force the contents into the upper duodenum. The finer, more liquid contents collect within the pyloric region and are forced into the upper duodenum by a combination of increased proximal gastric tone and antral systole (Camilleri et al 1985, Hinder and Kelly 1977, Kwiatek et al 2009, Tack 2000, Treacy et al 1990). Basically, the time it takes for a meal to leave the stomach is determined by the latency period between time of ingestion and the initiation of emptying and the rate of emptying itself. In general, ingested liquids empty significantly more rapidly than solids. The data available indicate a half time (T1/2) for emptying of a small liquid meal in the horse is ±30 minutes, and for a small solid meal ±90 minutes (Lohmann et al 2000, Ringger et al 1996, Sutton et al 2003). Clearly, however, these values are dependent upon experimental procedure and the size and composition of the diets used for the studies. For instance, Metayer et al found that increasing the amount of starch in a solid concentrate meal significantly increased the T1/2 value and that despite a greater rate of emptying when a large meal was fed the T1/2 value was increased compared to a smaller meal of the same concentrate composition (Metayer et al 2004) (Fig. 1.8).
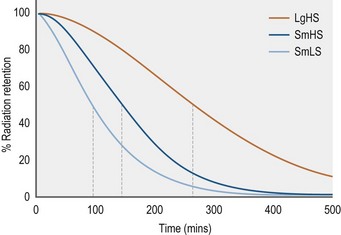
Figure 1.8 Gastric emptying, as measured by scintigraphy, of various meals labeled with Tcm99.
Adapted from Metayer et al 2004.
Conversely, the normal gastric wall increases in compliance in response to meal ingestion. This relaxation response has been divided into two phases – an initial “receptive relaxation” phase occurring as the meal is being eaten followed by a more prolonged “adaptive relaxation” phase (Kwiatek et al 2009, van den Berghe et al 2009). Some have referred to the whole process as “accommodation”. What this means with respect to a maximal volume limitation in the normal equine stomach has not yet been determined. It has been proposed that the first phase is induced by mechanosensors within the pharynx and/or esophagus, whereas the second phase is controlled by sensors within the duodenum that are triggered by food entering it from the stomach (Schwizer et al 2002). Such a biphasic response has been documented in horses ingesting a hay meal of either 0.5 or 1 g/kg body weight. The duration of the receptive relaxation phase was directly related to the time it took to eat the meal, and was greatest during ingestion of the larger meal. Furthermore, the only significant degree of second phase adaptive relaxation above baseline was after the large hay meal (Lorenzo-Figueras et al 2002) suggesting that it surpassed a threshold whereby duodenal interaction was induced which could putatively down-regulate the delivery of gastric contents to the duodenum. Based upon studies done in rats, carnivores and humans, “duodenal interaction” includes the release of CCK which, in turn, increases gastric wall compliance and decreases gastric emptying rate. In these species, there is a direct relationship between the amount of CCK released and the amount of fat in the diet. Accordingly, a follow-up to this study was done by Lorenzo-Figueras et al where a commercial sweet feed meal, instead of hay, was given, supplemented isocalorically with either corn oil or glucose (Fig. 1.9). Interestingly, the glucose-supplemented meal induced a significantly more prolonged receptive relaxation phase than the fat supplemented meal, which is just the opposite of what was expected based upon results in other species as described above. But there was no significant difference in emptying time between the meals as determined by the 13C-octanoic acid breath test technique (Lorenzo-Figueras et al 2005). Wyse et al found, however, that the addition of soybean oil to an oats and bran mixture significantly decreased gastric emptying rate in ponies, also determined by the 13C-octanoic acid breath test, although they did not measure receptive relaxation (Wyse et al 2001). In conclusion, this an area of research that needs a much more standardized approach in the horse.
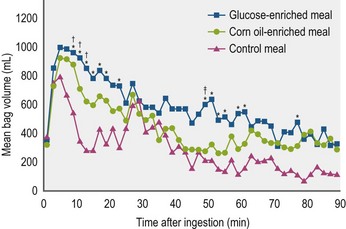
An increase in volume indicates relaxation. At time 0, the horses were fed either a control meal of 0.5 kg/kg body weight of a commercial 10% protein sweet feed mixture, or a control meal with 1.95 kcal/kg body weight of either glucose or corn oil mixed in. Each post-prandial volume represents a value from which the baseline value for that experiment has been subtracted. Bag volume was significantly higher than baseline during the entire length of the experiment after ingestion of each enriched meal, whereas it remained significantly higher than baseline for only 40 minutes after ingestion of the control meal. *Significant (P < 0.5) difference between glucose-enriched meal and control meal within the same period. †Significant difference between corn oil-enriched meal and control meal within the same period. ‡Significant difference between glucose and corn oil-enriched meals during the same period (Lorenzo-Figueras et al 2005).
Absorption
In vitro the absorptive capacity of the gastric epithelium was studied and the data showed that the VFA produced within the equine stomach are not absorbed from the stomach into the bloodstream (Argenzio et al 1974b). This suggested that the normal nonglandular (squamous) mucosa is particularly resistant to VFA penetration. More recently, Nadeau et al have found, in in vitro studies also, that VFA can penetrate into the non-glandular submucosa if the mucosa is exposed to a pH of <4.0 (Nadeau et al 2003). As indicated above, this can be detrimental to the integrity of the mucosal barrier but whether it allows significant movement of VFA from lumen to blood in vivo remains to be determined.
Key Points
• The proximal one-half of the equine stomach is lined with a non-glandular, squamous, mucosa that is similar to esophageal mucosa. The distal one-half is lined with glandular mucosa comprised of the classical cardiac, fundic and pyloric portions.
• As in other species, the fundic mucosa is the site of HCl secretion and the pyloric mucosa is the production site of gastrin, a major HCl secretagogue. HCl secretion is stimulated by food intake but continues at a low level even if the stomach is empty.
• In the horse allowed roughage intake ad libitum, there is a distinct pH gradient within the contents, with it being notably higher in those in the proximal vs the distal stomach. Salivary secretion contributes to the higher pH in the proximal region.
• There is a diverse and active gastric bacterial population that, dependent upon prevailing pH, converts some of the non structural carbohydrate-based feeds into lactic acid essentially and volatile fatty acids to a lesser extent. How much, if any, of these end-products is absorbed across the gastric mucosa is not well understood.
• Size and composition of diet appear to be important determinants of degree of fermentation receptive relaxation and emptying rate of the equine stomach. Limited data available indicate that, as in other species, liquids empty more rapidly than solids, but retention times for concentrates and forage may differ.
Small intestine
As in other mammalian species, the small intestine of the horse is divided into three major regions: duodenum, jejunum, and ileum. Relative to its body size, the horse has a rather short small intestine – around 25 meters in length in the 500 kg adult, with the majority being the jejunum with the duodenum and ileum being about 1 and 0.7 meters long respectively. The bile duct and the primary pancreatic duct open into the duodenal diverticulum which is located ~15 cm aboral to the pyloric sphincter. Because of this location, it is common for bile and pancreatic juice to reflux back into the stomach, especially if it is empty, since the sphincter remains open during the intervals between antral contractions (Kitchen et al 2000). A secondary pancreatic duct opens into the duodenum very close to the duodenal diverticulum (Nickel et al 1979).
The small intestine is lined throughout by a mucosa composed of finger-like villi, each of which is surrounded by a group of crypts; the surface epithelial cells arise from the crypts and migrate up the villi as they mature (Fig. 1.10). During maturation numerous small projections, called microvilli, develop on the luminal (apical) surface of certain cells and it is within these microvilli, especially those in the cells lining the top one-third of each villus, that the intestinal digestive enzymes are found. Those same cells incorporate the mechanisms involved in absorption of the digested nutrients, thus their designation as absorptive cells. Other cells that can be found within the surface epithelium are mucus (goblet) cells, enteroendocrine cells that interact with the ENS, Paneth cells and undifferentiated columnar cells. Within the center of each villus there is a circulatory network, the venous arm of which carries absorbed water soluble micronutrients into the portal vein system, and a lymphatic vessel into which the absorbed long-chain fats move to eventually attain their entrance into the blood stream via the thoracic duct (see section on small intestinal absorption). Enterocytes are connected to each other by tight junctions, which restrict the transmucosal flux of large molecules, although they are permeable to water and many low-molecular weight substances such as nicotinamide, ascorbic acid and biotin (Madara and Trier 1994).
Secretion
A considerable amount of fluid and other substances are secreted into the small intestine, predominantly from the liver and pancreas in order to aid digestion. In those species in which it has been studied, fat digestion is promoted by the interaction of bile salts secreted through the biliary system and lipase from the pancreas. Bile salt excretion in the equid appears to be dependent upon an intact entero-hepatic circulation, as in other species (Engelking et al 1989). The only actual flow data available is from studies using ponies where the bile flow rate was 18.6 ± 1.7 µl/kg-min, with a bile acid excretion rate of 0.18 ± 0.02 µmol/kg-min (Gronwall et al 1975). It is presumed that this represents true original bile production since there is no gall bladder in the horse in which the bile might be stored.
With respect to the pancreas, in contrast to most other species, the nonstimulated (basal) secretion in the horse is profuse and apparently continuous. Alexander and Hickson reported a secretory rate of 10–12 l/day in a 100 kg pony (Alexander & Hickson 1970) and Kitchen et al estimated that the average size adult horse secretes 20–25 L/day (Kitchen et al 2000). When further secretion is stimulated with secretin the concentration of bicarbonate in equine pancreatic juice does not increase, with a corresponding decrease in chloride concentration, as it does in other species. Rather, both remain much closer to their resting concentrations (Alexander & Hickson 1970) (Fig. 1.11). Nonetheless, the pH of equine pancreatic juice is basic (~8.0) due to its bicarbonate content (~30 mEq/l) and it is an important source of buffering against the gastric acid entering the duodenum (Alexander & Hickson 1970, Kitchen et al 2000). There is now some evidence that gastrin may also be a major stimulant of pancreatic water and electrolyte output in horses, comparable to the effect of secretin in equid and nonequid species (Merritt et al 1996, Kitchen et al 2000).
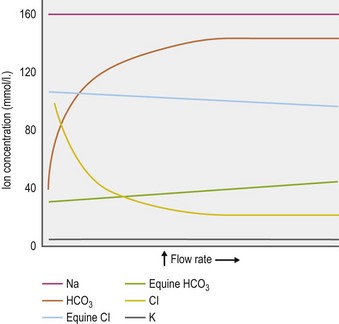
Figure 1.11 Trends in ion concentration in pancreatic juice in relation to flow rate.
Adapted from Alexander & Hickson 1970.
The concentrations of those digestive enzymes within the pancreatic juice of horses that have been investigated (i.e., amylase and trypsinogen (trypsin)), are very low in comparison to other species (Alexander & Hickson 1970, Kienzle et al 1994). Of interest is that, on a relative basis, equine pancreatic tissue contains much more lipase than any of the other digestive enzymes (Lorenzo-Figueras et al 2007) (Table 1-3). Whether this is also true for the juice itself remains to be determined. It is presumed, but not yet shown, that CCK, which is produced by specialized cells in the small intestinal mucosa, plays a major role in stimulating enzyme secretion from the equine pancreas. In other species CCK release from these cells is stimulated by a specific CCK releasing factor (CCK-RF) secreted into the intestinal lumen by another cell type in the mucosa and CCK-RF secretion itself is stimulated by the presence of trypsin in the lumen (Liddle 1995). CCK, along with other GI peptides, is present within the equine small intestinal mucosa (Kitamura et al 1984), but Lorenzo-Figueras and Merritt found that, in contrast to other species, it is immeasurable in equine plasma, even after meal ingestion (Lorenzo-Figueras & Merritt 2006).
Table 1-3 Comparative Pancreatic Tissue Specific Activity Expressed as Mean IU/mg Protein (Lorenzo-Figueras et al 2007)
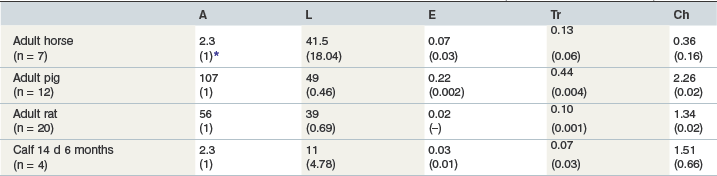
A = amylase; L = lipase; E = elastase; Tr = trypsin; Ch = chymotrypsin.
Under normal conditions, secretion of electrolytes by the small intestine itself, which occurs within the crypts, is normally out-paced by absorption so that net movement of water, which follows solute, is from lumen into bloodstream. Chloride is the major anion involved in this secretion (Hirota & McKay 2006, Venkatasubramanian et al 2010) and there is evidence that in horses its mechanism of secretion may be different from respective transport mechanisms in other species because it could not be blocked in vitro by the usual chloride channel blockers (Cehak et al 2009
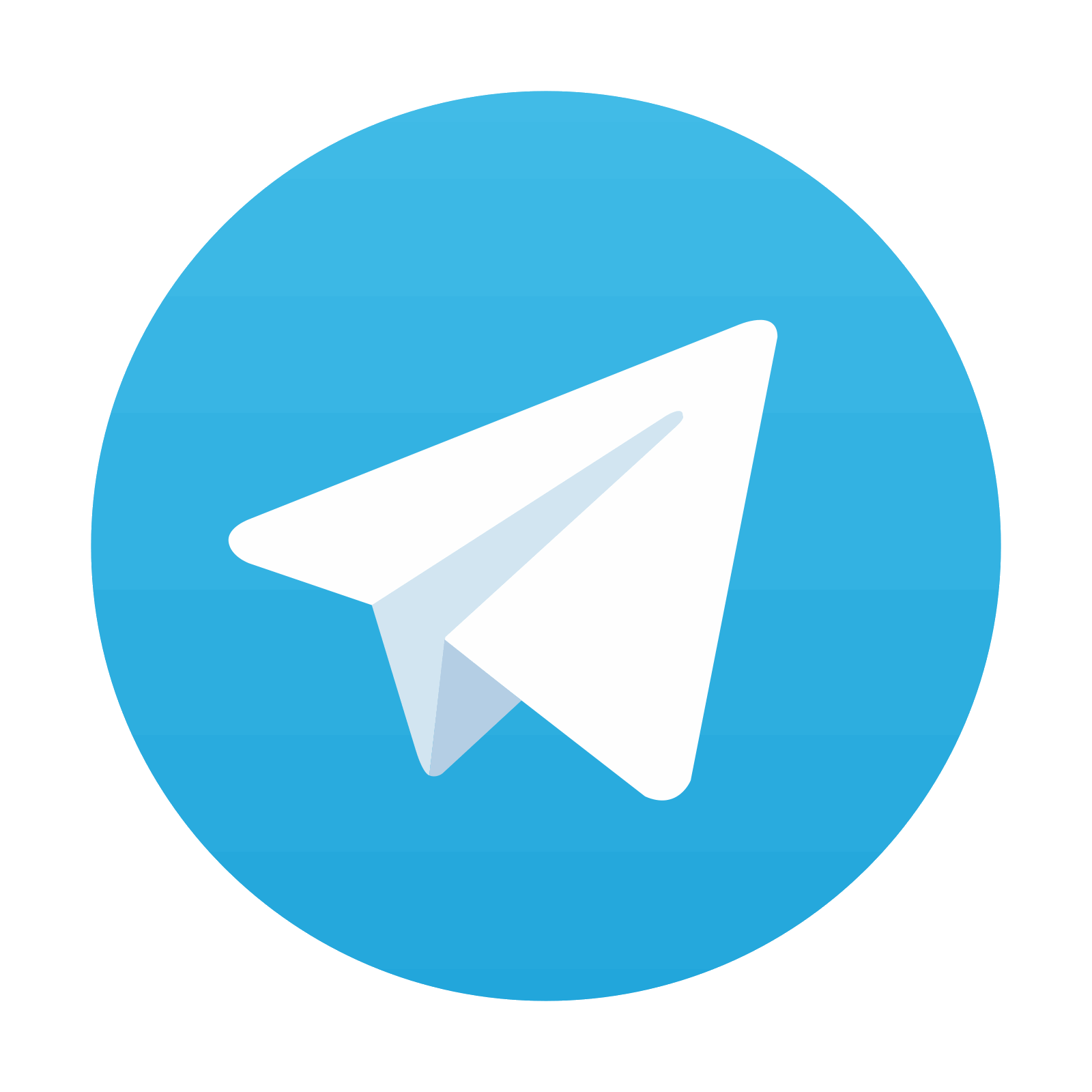
Stay updated, free articles. Join our Telegram channel
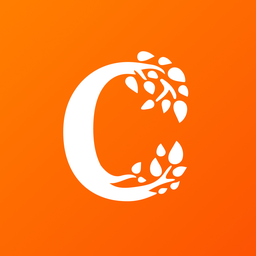
Full access? Get Clinical Tree
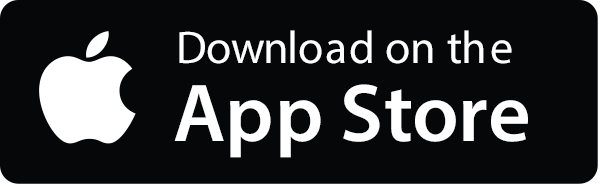
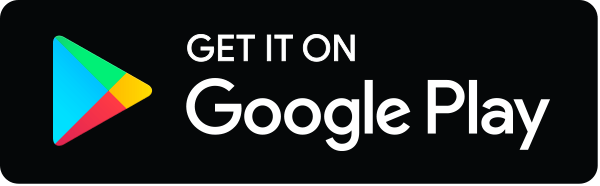