Chapter 14 Gastrointestinal Function A. Composition of Gasteric Secretions B. Control of Gastric Secretion D. Enterohepatic Circulation of Bile Acids V EXOCRINE PANCREATIC SECRETIONS A. Composition of Pancreatic juice B. Control of Pancreatic Secretions VI. OTHER GASTROINESTINAL HORMONES B. Carbohydrate Digestion and Absorption VIII. DISTURBANCES OF GASTROINTESTINAL FUNCTION C. Ischemia-Reperfusion Injury M. Clostridial-Associated Diseases in Horses and Cows IX. DISTURBANCES OF RUMEN FUNCTION A. Acute Rumen Indigestion (Rumen Overload, Lactic Acidosis) B. Acute Rumen Tympany (Bloat) The digestive system is composed of the gastrointestinal (GI) tract or the alimentary canal, salivary glands, the liver, and the exocrine pancreas. The principal functions of the gastrointestinal tract are to digest and absorb ingested nutrients and to excrete waste products of digestion. Most nutrients are ingested in a form that is either too complex for absorption or insoluble and therefore indigestible or incapable of being digested. Within the GI tract, much of these substances are solubilized and further degraded enzymatically to simple molecules, sufficiently small in size and in a form that permits absorption across the mucosal epithelium. This chapter describes the normal biochemical processes of intestinal secretion, digestion, and absorption. Once these issues have been put in perspective, the chapter explores the pathogenesis of the important gastrointestinal diseases of domestic animals and the biochemical basis for their diagnosis and treatment. Saliva is produced by three major pairs of salivary glands and by small glands distributed throughout the buccal mucosa and submucosa. Two types of secretory cells are found in the acinar portions of the salivary glands: (1) the mucous cells, which contain droplets of mucus, and (2) the serous cells, which contain multiple secretory granules. In those species that produce salivary amylase (e.g., pig and human), the secretory granules are the zymogen precursors of this enzyme. A third cell type is found lining the striated ducts. The striations along the basal borders of these cells are caused by vertical infoldings of the cell membrane, a characteristic of epithelial cells involved in rapid movement of water and electrolytes. The primary secretion of the acinar cells is modified by active transport processes of the ductal epithelium. The distribution of the different types of secretory cells in the salivary glands varies among species. The parotid glands of most animals are serous glands, which produce a secretion of low-specific gravity and osmolality containing electrolytes and proteins including certain hydrolytic enzymes. The mandibular (submaxillary) and sublingual glands are mixed salivary glands that contain both mucous and serous types of cells and produce a more viscous secretion that contains large amounts of mucus (Dukes, 1955). Mucus is an aqueous mixture of proteoglycans and glycoproteins. One of the most completely studied glycoproteins is mucin. Salivary mucins are O-glycosylated and consist of peptides with many oligosaccharides linked covalently to the hydroxyamino acid serine or threonine. The carbohydrate portion of submaxillary mucin from sheep is a disaccharide of N-acetylneuraminic acid (sialic acid) and N-acetylgalactosamine (Carlson et al., 1973). The enzymes that link protein with hexosamine have been purified from the mandibular glands of sheep (Carlson et al., 1973) and swine (Schachter et al., 1971). The physiological functions of mucin are related to its high viscosity. N-acetylneuraminic acid is the component responsible for the formation of viscous aqueous solutions and, at physiological pH, causes expansion and stiffening of the mucin molecule. The resistance of mucin to enzymatic breakdown is also due to the presence of disaccharide residues. Removal of terminal N-acetylneuraminic acid residues by action of neuraminidase significantly increases the susceptibility of peptide bonds to trypsin. The principal inorganic constituents of saliva are sodium, potassium, chloride, and bicarbonate, which, with the exception of bicarbonate, originate directly from the plasma. Rates of salivary flow vary depending on stimulation, and there are wide variations in electrolyte concentration. Saliva is formed by a process that initially requires uptake of sodium and other electrolytes from the interstitium of the terminal structural unit of the salivary gland, the acinus or end piece. Water flows passively. This primary or precursor fluid has a sodium concentration similar to plasma, and the potassium concentration is similar to or slightly higher than plasma. As the primary fluid passes from the acinus along the duct system, the concentration of sodium, potassium, and other electrolytes changes. In most species, there is net sodium absorption and potassium secretion. Wide variations in electrolyte composition may occur depending on the flow rate (Young and Schneyer, 1981), the salivary gland of origin, and the species (Table 14-1). The saliva of rodents contains the α-amylase, ptyalin, but this enzyme activity is absent in the saliva of dogs, cats, horses, cattle, and sheep (Dukes, 1955; Young and Schneyer, 1981). Salivary amylase splits the α1,4-glucosidic bonds of various polysaccharides. Salivary amylase is similar in major respects to pancreatic α-amylase, which is described in Section V.B. Salivary amylase initiates digestion of starch and glycogen in the mouths of those species that secrete the enzyme. The optimal pH for amylase activity is approximately 7, so that this activity ceases when the enzyme mixes with acidic gastric contents. Lingual lipase is secreted by Von Ebner’s gland of the tongue and is important in the digestive processes of the human newborn, rats, and preruminant calves (Cook et al.,1994; Plucinski et al., 1979). Saliva continuously bathes the oral cavity, which protects the surface epithelium. Ingested food is moistened and lubricated by saliva, thereby facilitating mastication and swallowing. Saliva also protects teeth from decay by washing food particles from the surfaces of the teeth and using its buffering capacity to neutralize the organic acids produced by bacteria normally present in the mouth. Saliva is necessary for vocalization, and, in some species that groom themselves, saliva promotes cooling as it evaporates. Additionally, it may be a source of pheromones. Salivary glands contain large numbers of growth factors, vasoactive serine proteases, and regulatory peptides (Cook et al., 1994). There is reason to believe that these glandular constituents affect a wide range of biological functions not necessarily limited to the alimentary system. Ruminants produce much greater quantities of saliva than do simple-stomached animals, and their saliva has a higher pH and bicarbonate ion concentration. In ruminants, saliva serves several unique functions (Phillipson, 1977). It is required for maintenance of the fluid composition of the contents of the rumen. The great buffering capacity of ruminant saliva is necessary to neutralize the large amounts of organic acids that are end products of rumen fermentation. Rumen bacteria for protein synthesis can utilize the urea in saliva. Protein synthesized in the rumen is then used to meet dietary protein requirements. In this way, urea nitrogen can be “recycled” through the amino acid pool of the body, and in ruminants it need not be considered an end stage in protein catabolism. The ability to reutilize urea has also been demonstrated in the horse, and this may be of particular benefit during periods of protein deficiency (Houpt and Houpt, 1971). The stomach is divided into two main regions on the basis of secretory function. The oxyntic gland area corresponds approximately to the body of the stomach in most species of domestic animals and also to the fundus in the dog and cat. The oxyntic glands contain (1) oxyntic or parietal cells that produce hydrochloric (HCl) acid, (2) peptic (zymogenic, chief) cells that produce pepsinogen, and (3) mucous cells. The pyloric gland area contains mucus-producing pyloric glands whose secretion is slightly alkaline. This area also contains the G cells, which produce the polypeptide hormone, gastrin. There are two components of gastric secretion. The surface epithelial cells and other mucus-producing cells continuously secrete the basal component. This component is neutral or slightly alkaline pH. The electrolyte composition is similar to that of an ultrafiltrate of plasma (Table 14-2). The basal secretion contains large amounts of mucus, which has a cytoprotective effect on the epithelium. The secretory component produced by the oxyntic gland cells in response to stimulation contains free HCl and pepsinogen, the principal enzyme of gastric digestion. The composition of gastric juice depends on the relative amounts of the basal and secretory components in the juice and, in turn, is a function of the flow rate of each. In the dog, gastric juice is produced in the resting state at a rate of approximately 5 ml/h. The composition is similar to that of the basal component, containing practically no peptic activity or HCl. When the flow of gastric juice is stimulated maximally, the dog may produce 80 ml or more per hour of a secretion containing large amounts of peptic activity and HCl. Na+, the principal cation in the basal secretion, is replaced to a large extent by H+ ion. The concentration of K+ is similar in both basal and stimulated secretions and, therefore, remains relatively constant at the various rates of flow. HCl and pepsinogen are secreted by separate mechanisms, but their production appears closely linked under physiological conditions. Stimulation of the vagus nerve or intravenous injection of gastrin increases pepsinogen and HCl levels together. Other stimuli may affect the two processes differently; for example, in the dog histamine infusion stimulates HCl production maximally but appears to inhibit pepsinogen secretion (Emas and Grossman, 1967). TABLE 14-2 Composition of Parietal and Nonparietal Secretions of Canine Gastric Mucosa a Determined in vivo using dogs with gastric fistulas (Gray and Bucher, 1941). b Determined in vitro with isolated gastric mucosa (Altamirano, 1963). c Calculated from bicarbonate concentration assuming pCO2 of 40 Torr. Pepsinogen is the zymogen, or inactive precursor, of pepsin, the principal proteolytic enzyme of gastric juice. Pepsinogen was first crystallized from the gastric mucosa of swine, and several pepsinogens have now been separated. The porcine pepsinogen has a molecular weight of approximately 43 kd and is composed of the pepsin molecule and several smaller peptides. One of these peptides has a molecular weight of 3.2 kd and is an inhibitor of peptic activity. Activation of pepsin from pepsinogen occurs by selective cleavage of this small basic peptide from the parent pepsinogen (Neurath and Walsh, 1976). Autocatalytic conversion begins below pH 6. At pH 5.4, the inhibitor peptide dissociates from the parent molecule, and at pH 3.5 to 4, the inhibitor is completely digested by pepsin. Pepsin has a very acidic isoelectric point and is stable in acidic solution below pH 6, but it is irreversibly denatured at pH 7 or above. In contrast, pepsinogen is stable in neutral or slightly alkaline solution. The optimal pH for peptic activity is generally between 1.6 and 2.5, but the effect of pH may vary with the substrate. Pepsin is capable of hydrolyzing peptide bonds of most proteins, mucin being one important exception. Pepsin splits bonds involving phenylalanine, tyrosine, and leucine most readily but can hydrolyze almost all other peptide bonds. In canines, gastric lipase is secreted in response to pentagastrin, histamine, prostaglandin E2, and secretin (Simpson, 2005). It parallels the secretion of gastric mucosa and plays a role in fat digestion. Unlike pepsin, it is not dependent on an acid pH, remains active in the small intestine, and constitutes up to 30% of the total lipase secreted over a 3-hour period. Gastric lipase as well as pepsin are not essential in fat digestion, but resulting fatty acids and peptides help coordinate gastric emptying and pancreatic secretion. Rennin is another proteolytic enzyme produced by the gastric mucosa and has characteristics that are similar to those of pepsin. It has been separated from pepsin in preparations from the stomachs of newborn calves. Rennin splits a mucopeptide from casein to form paracasein, which then reacts with calcium ion to form an insoluble coagulum. The coagulated milk protein probably delays gastric emptying and increases the efficiency of protein digestion in young calves. The oxyntic cells produce HCl. When the normal mucosa is stimulated, both Cl– and H+ are secreted together, but current evidence suggests that H+ and Cl– are secreted by separate, closely coupled mechanisms. Unstimulated oxyntic cells continuously secrete small amounts of Cl– in the absence of H+ secretion, and this mechanism is responsible for the negative charge of the resting mucosal surface of the stomach relative to the serosa. For every H+ secreted, an electron is removed that ultimately is accepted by oxygen to form OH–, which is neutralized within the cell by H+ from H2CO3. The HCO3– then enters the venous blood by means of a Cl–/HCO3– exchange (“alkaline tide”), and during HCl secretion, the pH of gastric venous blood frequently is greater than that of arterial blood (Davenport, 1966). The membrane-bound enzyme responsible for transport of H+ by the oxyntic cell is a K+-stimulated ATPase (Sachs et al., 1976; Wallmark et al., 1980) that serves as an H+/K+ exchange pump. At the time of oxyntic cell stimulation, the secretory membrane is altered to provide augmented K+ and Cl– conductances (Wolosin, 1985). KCl leaves the apical cell membrane passively, and net production of HCl results from the electroneutral exchange of K+ for H+ (Fig. 14-1). A variety of stimuli can initiate gastric secretion. The sight or smell of food or the presence of food within the mouth causes gastric secretion by a reflex mechanism involving the vagus nerve. The presence of certain foods within the stomach or distension of the stomach alone can also initiate both intrinsic and vagal nerve reflexes, which cause secretion of gastric fluid. In addition to neural reflexes, these stimuli also cause the release of the gastrin from the pyloric gland area, which enters the bloodstream, stimulating gastric secretion. The release of gastrin from G cells is inhibited by excess H+, and this negative feedback mechanism is important in the control of HCl production. Gastrin has been isolated in pure form from the antral mucosa of swine (Gregory et al., 1964). When administered intravenously, the purified hormone causes the secretion of HCl and pepsin and stimulates gastrointestinal motility and pancreatic secretion. Two separate peptides have been obtained from porcine gastric mucosa and have been designated gastrin I and gastrin II. Gastrin is a heptadecapeptide amide, with a pyroglutamyl N-terminal residue and with the amide of phenylalanine as the C-terminal residue (Fig. 14-2). In the center of the molecule is a sequence of five glutamyl residues, which give the molecule its acidic properties. Gastrin II differs from gastrin I only in the presence of a sulfate ester group linked to the single tyrosyl residue. The C-terminal tetrapeptide amide, Trp-Met-Asp-Phe-NH2, is identical in all species so far studied (Gregory, 1967). The tetrapeptide has all of the activities of the natural hormone. It is not as potent as the parent molecule, but lengthening of the peptide chain can increase activity. FIGURE 14-1 Movement of ions across the mucosal (apical) and serosal (basal) cell membranes of the parietal during HCl secretion. FIGURE 14-2 Amino acid sequence of porcine gastrin I (Gregory, 1966). Gastrin II differs from gastrin I by the presence of a sulfate ester group on the single tyrosyl residue. Gastrin-releasing peptide—as well as luminal peptides, digested protein, and acetylcholine—stimulates gastrin secretion from G cells and affects histamine release from enterochromaffin-like cells (Simpson, 2005). Gastrin is the only hormone known to simulate HCl (Walsh and Grossman, 1975). Histamine secreted locally within the mucosa has a major effect on the function of oxyntic cells (Soll and Grossman, 1978). For many years, histamine has been recognized as a potent stimulant of HCl production, but this effect was not inhibited by traditional antihistaminic drugs (H1 antagonists), and until the demonstration by Black et al. (1972) of H2 receptors in the stomach (the atrium and uterus), the physiological role of histamine in HCl secretion was controversial. Specific H2 antagonists (cimetidine) now have been shown to inhibit the secretory response not only to histamine but to other secretory stimuli as well (Grossman and Konturek, 1974). The complex of oxyntic cell receptors involved in the control of oxyntic cell function is shown in Figure 14-3. When the H2 receptor of the oxyntic cell is occupied by histamine, basal lateral adenylate cyclase is activated, resulting in increased cellular cyclic AMP (cAMP) and in a sustained secretory response. The secretogogue action of cAMP is mediated by the activity of cAMP-dependent protein kinases (Chew, 1985). Cholinergic stimulation of the oxyntic cell involves type I muscarinic receptors and a calcium activation pathway. Calmodulin inhibitors such as trifluoroperazine inhibit H+ secretion (Raphael et al., 1984). The Ca-calmodulin system may influence the rate of cAMP synthesis, and a more distal site of action has been suggested by the identification of a Ca-dependent protein kinase activity in a membrane fraction prepared from oxyntic cells that was rich in H+, K+-ATPase (Schlatz et al., 1981). A specific receptor for gastrin has been demonstrated on oxyntic cells and a specific gastrin antagonist, proglutamide, inhibits H+ production. Gastrin appears to act synergistically with histamine and acetylcholine (AcCh) to regulate H+ production but the actual mechanism of action of gastrin is unknown. FIGURE 14-3 Pathways of secretagogue action on the parietal cell. Stimulation by gastrin and acetylcholine is mediated by entry of Ca2+ onto the cell. Histamine activates adenylate cyclase with production of cAMP, the action of which is mediated by protein kinase. Prostaglandins, in addition to inhibiting HCl secretion, also act on a mucosal cell population that is distinct from oxyntic cells, which secrete cytoprotective substances (mucin, glycosaminoglycans). The ulcerogenic effects of inhibitors of prostaglandin synthesis (indomethacin, aspirin) apparently are the result of inhibition of the protective effect of endogenous prostaglandins. Knowledge of the molecular aspects of receptor function of HCl secretion by oxyntic cells now provides the opportunity for specific pharmacological intervention for the control and treatment of ulcerative diseases of the upper gastrointestinal tract that appear to be the result of HCl-induced mucosal injury (Aclund et al., 1983; Becht and Byars, 1986; Campbell-Thompson and Merritt, 1987). Potential therapeutic target sites are listed in Table 14-3. Famotidine is commonly administered to dogs and cats. Injectable ranitidine is administered to foals and horses during critical stages of gastrointestinal ulceration before switching to oral administration of omeprazole. The hepatocytes continuously secrete bile into the bile canaliculi; it is transported through a system of ducts to the gallbladder, where it is modified, concentrated, and stored. During digestion, bile is discharged into the lumen of the duodenum, where it aids in emulsification, hydrolysis, and solubilization of dietary lipids. The digestive functions of bile are accomplished almost exclusively by the detergent action of its major components, the bile salts and phospholipids. The carboxyl group of the bile acids is completely ionized at the pH of bile and is neutralized by Na+ resulting in the formation of bile salts. These bile salts are effective detergents. They are amphipathic molecules that have both hydrophobic and hydrophilic regions. In low concentrations, bile salts form molecular or ideal solutions, but when their concentration increases above a certain critical level, they form polymolecular aggregates known as micelles. The concentration at which these molecules aggregate is called the critical micellar concentration (CMC). Bile salt micelles are spherical and consist of a central nonpolar core and an external polar region. Fatty acids, monoglycerides, and other lipids are solubilized when they enter the central core of the micelle and are covered by the outside polar coat. Solubilization occurs only when the CMC is reached. For the bile salt-monoglyceride-fatty acid-water system present during normal fat digestion, the CMC is approximately 2 mM, which normally is exceeded both in bile and in the contents of the upper small intestine (Hofmann, 1963, 1967). Phospholipids, principally lecithin, are also major components of bile. In the lumen of the small intestine, pancreatic phospholipase catalyzes the hydrolysis of lecithin, forming free fatty acid and lysolecithin. The latter compound also is a potent detergent, which acts with the bile salts to disperse and solubilize lipids in the aqueous micellar phase of the intestinal contents. The primary bile acids (BA) are C-24 carboxylic acids synthesized by the liver from cholesterol. BA synthesis is the major end-stage pathway for cholesterol metabolism (Danielsson, 1963). Cholic acid (3α,7α,12α-trihydroxy-5β-cholanoic acid) (CA) and chenodeoxycholic acid (3α-,7α-dihydroxy-5β-cholanoic acid) (CDCA) are the primary BA synthesized by most species of domestic animals. In swine, CDCA is hydroxylated at the 6α position by the liver to yield hyocholic acid (HCA), which is a major primary BA in this species. BA are secreted as amino acid conjugates of either glycine or taurine. Taurine conjugates predominate in the dog, cat, and rat. In the rabbit, the conjugating enzyme system appears to be almost completely specific for glycine (Bremer, 1956). Both taurine and glycine conjugates are present in ruminants. In the newborn lamb, 90% of the bile acids are conjugated with taurine. As the lamb matures, glycine conjugates increase to reach one-third of the total BA in mature sheep (Peric-Golia and Socic, 1968). Under normal conditions, only conjugated BA are present in the bile and in the contents of the proximal small intestine. In the large intestine, the conjugated BA are hydrolyzed rapidly by bacterial enzymes so that in the contents of the large intestine and in the feces, free or unconjugated BA predominate. Several genera of intestinal bacteria, including clostridium, enterococcus, bacteroides, and lactobacillus, are capable of splitting the amide bonds of conjugated BA. Intestinal bacteria also modify the basic structure of the BA. One such reaction is the removal of the α-hydroxyl group at the 7 position of CA or CDCA. These bacterial reactions yield the secondary BA, deoxycholic acid (DCA), and lithocholic acid (LCA) (Gustafsson et al., 1957). LCA is relatively insoluble and is not reabsorbed to any great extent (Gustafsson and Norman, 1962). DCA is reabsorbed from the large intestine in significant quantities and is either rehydroxylated by the liver to CA and secreted (Lindstedt and Samuelsson, 1959) or secreted as the conjugated DCA. The extent to which bacteria transform the primary BA depends on the nature of the diet, the composition of the intestinal microflora, and the influences of these and other factors on intestinal motility (Gustafsson, 1969; Gustafsson et al., 1966; Gustafsson and Norman, 1969). The enterohepatic circulation begins as conjugated BA enter the duodenum and mix with the intestinal contents, forming emulsions and micellar solutions. The BA are not absorbed in significant amounts from the lumen of the proximal small intestine. Absorption occurs primarily in the ileum (Lack and Weiner, 1961, 1966; Weiner and Lack, 1962) where an active transport process has been demonstrated (Dietschy et al., 1966). The absorbed conjugated BA pass unaltered into the portal circulation (Playoust and Isselbacher, 1964) and return to the liver, where the cycle begins again. This arrangement provides for optimal concentrations of BA in the proximal small intestine where fat digestion occurs and then for efficient absorption after these functions have been accomplished. Absorption of unconjugated BA from the large intestine accounts for 3% to 15% of the total enterohepatic circulation (Weiner and Lack, 1968). In dogs, the total BA pool was estimated to be 1.1 to 1.2 g. The half-life of the bile acids in the pool ranged between 1.3 and 2.3 days, and the rate of hepatic synthesis was 0.3 to 0.7 g/day. Because the daily requirement for bile acids greatly exceeds the normal synthetic rate, the repeated reutilization of the BA is facilitated by the enterohepatic circulation. Under steady-state conditions, the total BA pool passes through the enterohepatic circulation approximately 10 times each day. The size of the BA pool depends on the diet, the rate of hepatic synthesis, and the efficiency of the enterohepatic circulation. Surgical removal of the ileum in dogs interrupts the enterohepatic circulation, thereby increasing the BA turnover and reducing the size of the BA pool (Playoust et al., 1965). In diseases of the ileum, there may be defective BA reabsorption and a bile salt deficiency. If the deficiency is severe, the utilization of dietary fat may be impaired, resulting in steatorrhea and impaired absorption of the fat-soluble vitamins. The exocrine pancreas is an acinus gland with a general structure that is similar to the salivary glands. The cytoplasm of the secretory cells contains numerous zymogen granules, which vary in size and number depending on the activity of the gland. These granules contain the precursors of the hydrolytic enzymes responsible for digestion of the major components of the diet. Cells of the terminal ducts appear to secrete the HCO3– responsible for neutralizing the HCl, which enters the duodenum from the stomach. The cation content of pancreatic secretion is similar to that of plasma, Na+ being the predominant cation and the concentrations of K+ and Ca2+ being much lower. A unique characteristic of pancreatic fluid is its high HCO3– concentration and alkaline pH. In the dog, the pH ranges from 7.4 to 8.3, depending on HCO3– content. The volume of pancreatic secretion is directly related to its HCO3– content, and the pH increases and Cl– concentration decreases as the rate of flow increases. The Na+ and K+ concentrations and osmolality appear to be independent of the secretory rate (Fig. 14-4). FIGURE 14-4 Influence of secretory rate on the electrolyte composition of canine pancreatic juice. From Bro-Rasmussen et al. (1956). The amylase produced by the pancreas catalyzes the specific hydrolysis of α-1,4-glucosidic bonds, which are present in starch and glycogen (α-1,4-glycan-4-glycan hydrolase). Pancreatic amylase appears to be essentially identical to the amylase of saliva. It is a calcium-containing metalloenzyme. Removal of calcium by dialysis inactivates the enzyme and markedly reduces the stability of the apoenzyme. Pancreatic amylase has an optimal pH for activity of 6.7 to 7.2 and is activated by Cl–. After synthesis of pancreatic α-amylase in the ribosomes, the enzyme is transferred from the endoplasmic reticulum to cytoplasmic zymogen granules for storage. It is secreted in active form upon stimulation of the acinar cells. Newborn calves and pigs secrete amylase at a significantly lower rate than mature animals. The rate of synthesis is also influenced by diet. Animals fed a high-carbohydrate diet synthesize amylase at several times the rate of animals on a high-protein diet. Unbranched α-1,4-glucosidic chains, such as those found in starch, are hydrolyzed in two steps. The first is rapid and results in formation of the maltose and maltotriose. The second step is slower and involves hydrolysis of maltotriose into glucose and maltose. Polysaccharides such as amylopectin and glycogen contain branched chains with both α-1,4- and α-1,6-glucosidic linkages. When α-amylase attacks these compounds, the principal products are maltose (α-1,4-glycosidic bond), isomaltose (α-1,6-glucosidic bond), and small amounts of glucose. Final hydrolysis of the maltose and isomaltose occurs at the surface of the mucosal cell, where the enzymes maltase and isomaltase are integral parts of the microvillous membrane. The proteolytic enzymes of the pancreas are responsible for the major portion of protein hydrolysis, which occurs within the lumen of the gastrointestinal tract. The pancreas secretes two types of peptidases. Trypsin, chymotrypsin, and elastase are endopeptidases that attack peptide bonds along the polypeptide chain to produce smaller peptides. The exopeptidases attack either the carboxy-terminal or amino-terminal peptide bonds, releasing single amino acids. The principal exopeptidases secreted by the pancreas are carboxypeptidases A and B. The endopeptidases and exopeptidases act in complementary fashion (Table 14-4), ultimately producing free amino acids or very small peptides. The free amino acids are absorbed directly, and the small peptides are further hydrolyzed by the aminopeptidases of the intestinal mucosa. The pancreatic peptidases are secreted as the inactive proenzymes (zymogens), trypsinogen, chymotrypsinogen, and the procarboxypeptidases A and B. Trypsinogen is converted to active trypsin in two ways. At alkaline pH, trypsinogen can be converted autocatalytically to trypsin. The activated enzyme is then capable of converting more zymogen to active enzyme. Trypsinogen also can be activated by the enzyme enterokinase, which is produced by duodenal mucosa. The latter reaction is highly specific in that enterokinase will activate trypsinogen but not chymotrypsinogen. Chymotrypsinogen, proelastase, and the procarboxypeptidases A and B are converted to active enzymes by the action of trypsin. The amino acid sequences and other structural characteristics of bovine trypsinogen and chymotrypsinogen have been determined (Brown and Hartley, 1966; Hartley et al., 1965; Hartley and Kauffman, 1966). The polypeptide chain of trypsinogen contains 229 amino acid residues. Activation of trypsinogen occurs with hydrolysis of a single peptide bond located in the 6 position between lysine and isoleucine. As the C-terminal hexapeptide is released, enzyme activity appears along with a helical structure of the parent molecule. Chymotrypsinogen A is composed of 245 amino acid residues and has numerous structural similarities to trypsinogen. Activation of the chymotrypsinogen also occurs with cleavage of a single peptide bond. The pancreas produces several lipolytic enzymes with different substrate specificities. The most important of these from a nutritional viewpoint is the lipase responsible for hydrolysis of dietary triglyceride. This enzyme has the unique property of requiring an oil-water interface for activity so that only emulsions can be effectively attacked. The principal products of lipolysis are glycerol, monoglycerides, and fatty acids. The monoglycerides and fatty acids accumulate at the oil-water interface and can inhibit lipase activity. Transfer of these products from the interface to the aqueous phase is favored by HCO3– secreted by the pancreas and by the bile salts. Two other carboxylic ester hydrolases have been characterized in pancreatic secretion. Both enzymes have an absolute requirement for bile salts, in contrast to glycerol ester hydrolase, which is actually inhibited by bile salts at pH 8. One of the enzymes requiring bile salts is a sterol ester hydrolase responsible for hydrolysis of cholesterol esters, and the other enzyme hydrolyzes various water-soluble esters. The pancreas also secretes phospholipase A, which in the presence of bile converts lecithin to lysolecithin, an effective detergent that contributes to the emulsification of dietary fat. Pancreatic secretion is controlled and coordinated by both neural and endocrine mechanisms. When ingesta or HCl enters the duodenum, the hormone secretin, which is produced by the duodenal mucosa, is released into the circulation. Secretin increases the volume, pH, and HCO3– concentration of the pancreatic secretion. Secretin is a polypeptide hormone containing 27 amino acid residues, and all 27 amino acids are required to maintain the helical structure of the molecule and its activity (Bodanszky et al., 1969). The C-terminal amide of secretin is a property shared with other polypeptide hormones such as gastrin and vasopressin, which act on the flow of water in biological systems (Mutt and Jorpes, 1967). In addition to its effects on the pancreas, secretin also increases the rate of bile formation. The secretin-stimulated pancreatic juice has a large volume, high HCO3– concentration but a low enzyme activity. Stimulation of the vagus nerve causes a significant rise in pancreatic enzyme concentration. This type of response also is produced by cholecystokinin (pancreozymin), another polypeptide hormone produced by the duodenal mucosa, which also causes contraction of the gallbladder. The C-terminal pentapeptide of cholecystokinin-pancreozymin is exactly the same as that of gastrin. This fascinating relationship suggests that gastrin and cholecystokinin-pancreozymin participate in some integrated yet poorly understood system of digestive control. Several molecular forms of cholecystokinin (CCK) exist (Ward and Washabau, 2005). CCK-33, CCK-39, and CCK-59 are the predominant forms that account for most of the gastrointestinal hormone responses. Endocrine cells in the duodenum and jejunum secrete CCK in response to intraduodenal fatty acids, amino acids, and H+ ion. CCK-8 is particularly important in cats. Intraluminal distension will activate CCK-8 containing neurons, resulting in acetylcholine release from the myenteric plexus and subsequent peristaltic reflexes in the ileum and colon. CCK-8 neurons in the brain are involved in mediating the satiety response following eating. A large number of polypeptides have been isolated from the gastrointestinal mucosa and have been classified as gut hormones (Table 14-5). Some of these substances have not yet met all the rigid physiological requirements of true hormones. Some may have paracrine rather than endocrine activities—that is, their actions are on cells and tissues in the immediate vicinity of the cells of origin rather than being released into the vascular system. Motilin is a polypeptide containing 22 amino acids that was originally isolated from porcine duodenal mucosa (Brown et al., 1971). The amino acid composition and sequence have been described (Brown et al., 1972, 1973). Immunoreactive motilin has been found in the enterochromaffin cells of the duodenum and jejunum of several species (Polak et al., 1975), and, by means of radioimmunoassay, motilin has been identified in the plasma of dogs (Dryburgh and Brown, 1975). Motilin has been shown to stimulate pepsin output and motor activity of the stomach (Brown et al., 1971) and to induce lower esophageal sphincter contractions (Jennewein et al., 1975). Studies by Itoh et al. (1978) suggest that motilin plays an important role in initiating interdigestive gastrointestinal contractions, which are referred to as the interdigestive motility complex or the migrating motility complex (MMC). The cyclic release of motilin from the intestinal mucosa coordinates gastric, pancreatic, and biliary secretions with phase III of the MMC (Ward and Washabau, 2005). Erythromycin has been shown to induce an MMC similar to motilin and, along with other macrolide-like antibiotics, might be useful in selected cases with motility disorders. Somatostatin, which is named for its activity of inhibitory release of growth hormone from the pituitary gland, has been purified from ovine and bovine hypothalamus. The hypothalamic hormone is composed of 14 amino acids. Somatostatin also has been demonstrated in the stomach, pancreas, and intestinal mucosa in concentrations higher than in the brain (Pearse et al., 1977). Somatostatin from porcine intestine has been isolated and sequenced, and it contains 28 amino acids and apparently is a prohormone (Pradayrol et al., 1980). Somatostatin is a potent inhibitor of insulin and glucagon release. It also inhibits gastrin release and gastric acid secretion (Barros D’Sa et al., 1975; Bloom et al., 1974), apparently acting independently on parietal cells and on G cells. These and a variety of other physiological effects suggest that somatostatin has important gastrointestinal regulatory functions. Enteroglucagon is the hyperglycemic, glycogenolytic factor isolated from the intestinal mucosa. It occurs in two forms, one a 3.5 kd form and another somewhat larger (Valverde et al., 1970). Enteroglucagon differs from pancreatic glucagon biochemically, immunologically, and in its mode of release. The physiological function of enteroglucagon is not known, but its release from the mucosa following a meal and the associated increase in circulating blood levels have suggested a regulatory role on bowel function (Pearse et al., 1977). Enteroglucagon also differs significantly from the glucagon produced by the A cells of the gastric mucosa of the dog (Sasaki et al., 1975). Canine gastric glucagon is biologically and immunochemically identical to pancreatic glucagon. Gastric glucagon appears to be unique to the dog, similar activity not being observed in the stomach of the pig or the abomasum of cattle and sheep. The microvillous membrane of the intestinal mucosa, because of its lipid composition, acts as a barrier to water and water-soluble substances. Water and polar solutes penetrate the mucosa in one of three ways. They may pass through aqueous pores or channels that connect the luminal surface of the cell with the apical cytoplasm, they may attach to membrane carriers that facilitate passage through the lipid phase of the mucosal cell membrane, or they may pass paracellularly through tight junctions (shunt pathway). Transport of water and water-soluble compounds is influenced by the permeability characteristics of the limiting membrane and by the nature of the driving forces that provide energy for transport. Passive movement occurs either by simple diffusion or as a result of concentration gradients (activity), pH, osmotic pressure, or electrical potential that may exist across the membrane. The movement of an ion in the direction of an electrochemical gradient is considered passive in nature. Active transport is said to occur when a substance moves in a direction opposite that of an established electrochemical gradient. Most water-soluble compounds, such as monosaccharides and amino acids, cannot diffuse across the intestinal mucosal membrane at rates that are adequate to meet nutritional requirements. The transport of these nutrients requires membrane carriers, which are integral parts of the membrane and their binding is highly specific. Carrier-mediated transport systems can be saturated and competitively inhibited by related compounds. Three types of carrier transport mechanisms are recognized (Curran and Schultz, 1968). (1) Active transport, as stated previously, involves movement of electrolytes against an electrochemical gradient. In the case of nonelectrolytes such as glucose, active transport is defined as movement against a concentration gradient. Active transport requires metabolic energy and is inhibited by various metabolic blocking agents or by low temperature. (2) Facilitated diffusion occurs when the passive movement of a substance is more rapid than can be accounted for by simple diffusion. Facilitated diffusion systems may increase the rate of movement across the membrane by two or three orders of magnitude. The responsible carrier mechanism is similar to that involved in active transport in that it displays saturation kinetics, may be inhibited competitively, and is temperature dependent. However, transport does not occur against concentration or electrochemical gradients, and direct expenditure of energy is not required. (3) Exchange diffusion is a transfer mechanism similar to facilitated diffusion and was postulated originally to explain the rapid transfer of radioactive Na+ across epithelial cell membranes in vitro. The mechanism involves the exchange of one ion for another of like charge (e.g., Na+ and H+ or Cl– and HCO3–), not giving rise to net transport but contributing in a major way to unidirectional flux rate. In the intestine, net water absorption is the result of bulk flow through pores. Diffusion in the usual sense plays no important role in water movement. When bulk flow of water occurs, it is possible for solutes to move across the membrane in the direction of flow by a phenomenon called solvent drag. The effect of solvent drag on the transport of a given solute depends on the rate of volume flow and on the reflection coefficient, an expression of the relationship between the radius of membrane pores and the radius of the solute molecule being transported. By means of solvent drag, it is possible for a solute such as urea to be transported by the intestine against a concentration gradient (Hakim and Lifson, 1964). Na+ and Cl– are the major ions in the fluid that are transported by the intestine during absorption or secretion, and under most conditions, transport of these two ions is coupled. The transport of water and electrolytes by the intestinal mucosa is a dynamic process, with rapid unidirectional fluxes of both occurring continuously. Net absorption occurs when the flow from lumen to plasma exceeds that from plasma to lumen. Active transport of Na+ can occur along the entire length of the intestine, but the rate and net absorption is greatest in the ileum and colon. Na+ transport is by an energy-requiring “sodium pump” mechanism that is intimately associated with the Na+-K+-ATPase located within the basolateral cell membrane of the absorptive epithelial cell. Three mechanisms exist for the entry of Na+ at the brush border: (1) electrodiffusion down a concentration gradient, (2) cotransport of electrolytes that either enter (Cl–) or exit (H+) the cell as Na+ enters, and (3) Na+ entry coupled with organic nonelectrolytes (glucose, amino acids). Current evidence suggests that in the absence of the absorption of nonelectrolytes, electroneutral uptake accounts for most NaCl absorption. At the brush border, Na+ enters down a concentration gradient but exits at the basolateral cell surface against a substantial gradient. Maintenance of the transmembrane Na+ gradient by the Na pump requires continual metabolism and generation of ATP. The Na+-K+-ATPase can be inhibited by cardiac glycosides such as ouabain, which are effective inhibitors of Na+ transport. The Na+ gradient ultimately serves as an energy source for transport of other solutes (Schultz and Curran, 1970). In the jejunum, net absorption of sodium occurs slowly unless nonelectrolytes, such as glucose or amino acids, are absorbed simultaneously. In the ileum, Na+ absorption is independent of glucose absorption. Net water absorption in the jejunum is almost entirely dependent on the absorption of glucose and other nonelectrolytes, whereas absorption from the ileum is unaffected by glucose. The differential effect of glucose on absorption from the jejunum and ileum is the result of fundamental differences in electrolyte transport mechanisms in these two regions of the intestine. As Na+ is transported across the mucosa, an equivalent amount of anion must be transported to maintain electrical neutrality. A major fraction of Cl– absorption can be accounted for by passive cotransport with Na+. Under certain circumstances, Cl– enters the cell in exchange for HCO 3–. Dietary K+ is absorbed almost entirely in the proximal small intestine. Absorption across the intestinal mucosa occurs down a concentration gradient (high luminal concentration to a low concentration in plasma). The intestinal fluid reaching the ileum from the jejunum has a K+ concentration and a Na+/K+ ratio that are similar to plasma. In the ileum and colon, the rate of Na+ absorption is much greater than that of K+ so that, under normal conditions, the Na+/K+ ratio in the feces is much lower than that of plasma, approaching a ratio of 1. The absorption of water has been one of the most extensively studied aspects of intestinal transport. Water movement is the result of bulk flow through membranous pores, and simple diffusion plays only a minor role. The question of whether water is actively or passively transported has been the subject of considerable controversy, and the controversy itself points to the fundamental difficulties that arise in trying to establish a definition of active transport. Hypertonic saline solutions can be absorbed from canine intestine in vivo and from canine and rat intestine in vitro. These observations indicate that water absorption can occur against an activity gradient and that the process is dependent on metabolic energy. This suggests that an active transport process is involved, but Curran (1965) presented an alternate interpretation, which is now generally accepted. This view is that water transport occurs secondarily to active solute transport and is the result of local gradients established within the mucosal membrane. Water transport is then coupled to the energy-dependent processes responsible for solute transport but is one step removed from it. In the dog and probably other carnivores, the ileum is the main site of net Na+ and water absorption. In the dog, the colon accounts for no more than perhaps 20% of the total. In herbivorous animals that have a well-developed large intestine, there may actually be a net secretion of water within the small intestine during digestion. For example, in the guinea pig (Powell et al., 1968) and horse (Argenzio, 1975), all net absorption of water takes place in the cecum and colon. Vasoactive intestinal polypeptide (VIP) and acetylcholine have an important role in fluid and electrolyte balance (Hall and German, 2005). As mediators of secretion, they increase intracellular calcium and cyclic adenosine monophosphate (cAMP), inhibit neutral sodium and chloride absorption, and facilitate transcellular chloride efflux. Some bacterial infections result in diarrhea because of an increase in cAMP; functional tumors of VIP-producing cells can also produce diarrhea. Noradrenaline, somatostatin, and opioids, which are the important regulators of absorption, lower intracellular cAMP and calcium concentrations and stimulate neutral NaCl absorption. For these reasons, they can have antidiarrheal effects. a. Starch and Glycogen Carbohydrate is present in the diet primarily in the form of polysaccharides. The most common polysaccharides are starch, glycogen, and cellulose. Starch and glycogen are composed of long chains of glucose molecules linked together by repeating α-1,4-glucosidic bonds. Branch points of the chains are linked by α-1,6-glucosidic bonds. In those species that secrete salivary amylase, digestion of starch and glycogen begins in the mouth when this enzyme mixes with food. The action of salivary amylase is interrupted in the stomach, however, because of the low pH of the gastric secretion. Starch digestion begins again in the proximal small intestine with the highly specific action of pancreatic amylase on α-1,4-glucosidic bonds. This enzyme catalyzes a series of stepwise hydrolytic reactions, resulting in formation of the principal end products of starch digestion, the disaccharides maltose and isomaltose, and small amounts of glucose. Glucose is absorbed directly by the intestinal mucosa and transported to the portal vein. Enzymes of the intestinal cell brush border hydrolyze the disaccharides further. b. Cellulose Cellulose, like starch, is a polysaccharide of glucose but differs from starch in that the glucose molecules are linked by β-1,4-glucosidic bonds. All species can utilize starch, but only animals that have extensive bacterial fermentation within the gastrointestinal tract utilize cellulose indirectly as a significant source of energy. Ruminant species digest cellulose most efficiently, but other animals in which the large intestine is well developed (e.g., the horse) also utilize cellulose as an important energy source. In ruminants, hydrolysis of cellulose is accomplished by cellulitic bacteria, which are part of the complex rumen microflora. The primary end products of cellulose fermentation are short-chain fatty acids: acetic, propionic, and butyric acids. These are absorbed directly from the rumen and serve as the major source of energy for ruminants. Propionic acid is the major precursor for carbohydrate synthesis in mature ruminants. Maltose and isomaltose are the disaccharides (glucose-glucose) produced as end products of starch digestion. The diet also may contain lactose (galactose-glucose) and sucrose (fructose-glucose). There is general agreement that disaccharide digestion is completed at the surface of the cell by disaccharidases (Gray, 1975), which are components of the brush border (Table 14-6). The disaccharidases have been solubilized from the brush border and partially purified. Sucrase and isomaltase have been purified together as a two-enzyme complex (Gray et al., 1979; Kolinska and Semenza, 1967), and this enzyme complex accounts for the total hydrolysis of the products of amylase digestion (Gray et al., 1979; Rodriguez et al., 1984). The mutual mucosa contains two enzymes with lactase activity. One of these is a nonspecific β-galactosidase that hydrolyzes synthetic β-galactosides effectively but hydrolyzes lactose at a slow rate. This enzyme has an optimal pH of 3 and is associated with the lysosomal fraction of the cell. The other lactase hydrolyzes lactose readily, is associated with the brush border fraction of the cell, and is the enzyme of primary importance in the digestive process (Alpers, 1969). Maltase, isomaltase, and sucrase are almost completely absent from the intestine in newborn pigs (Dahlqvist, 1961) and calves. The activity of these disaccharidases increases after birth and reaches adult levels during the first months of life. Lactase activity is highest at birth and decreases gradually during the neonatal period. The relatively high lactase activity may be an advantage to the newborn in utilizing the large quantities of lactose present in their diets. Bywater and Penhale (1969) demonstrated lactase deficiency following acute enteric infections and suggested that lactose utilization may be decreased in such cases. a. Specificity of Monosaccharide Transport Regardless of whether monosaccharides originate in the lumen of the intestine or are formed at the surface of the mucosal cell, transport across the mucosa involves processes that have a high degree of chemical specificity. Glucose and galactose are absorbed from the intestine more rapidly than other monosaccharides. Fructose is absorbed at approximately half the rate of glucose, and mannose is absorbed at less than one-tenth the rate of glucose (Kohn et al., 1965). Glucose and galactose can be absorbed against a concentration gradient. The monosaccharides that are transported most efficiently against gradients have common structural characteristics: (1) the presence of a pyranose ring, (2) a carbon atom attached to C-5, and (3) a hydroxyl group at C-2 with the same stereoconfiguration as D-glucose, but these features are not absolute requirements. Both D-xylose, which has no substituted carbon atom at C-5, and D-mannose, which lacks the appropriate hydroxyl configuration at C-2, can be transported against concentration gradients under specific experimental conditions (Alvarado, 1966b). Glucose transport is competitively inhibited by galactose (Fisher and Parsons, 1953) and by a variety of substituted hexoses that compete with glucose for carrier binding sites. The glucoside phlorizin is a potent inhibitor (Alvarado and Crane, 1962; Parsons et al., 1958). Phlorizin also competes for binding sites but has a much higher affinity for these sites than does glucose. The absorptive surface of the mucosal cell is the microvillous membrane, or brush border. It is through this part of the plasma membrane that glucose must pass during the initial phase of mucosal transport. Techniques have been developed for isolating highly purified preparations of microvillous membranes from mucosal homogenates (Forstner et al., 1968). Faust et al. (1967) studied the binding of various sugars to these isolated membrane fractions. They found that D-glucose was bound by the membrane preferentially to L-glucose or to D-mannose and that glucose binding was completely inhibited by 0.1 mM phlorizin. The specificity of their observations suggested that binding represented an initial step in glucose transport, namely, attachment to a membrane carrier. b. Sodium Requirement The absorption of glucose and other monosaccharides is influenced significantly by Na+ (Kimmich, 1973; Schultz and Curran, 1970). When Na+ is present in the solution bathing the intestinal mucosa, glucose is absorbed rapidly, but when Na+ is removed and replaced by equimolar amounts of other cations, glucose absorption virtually stops (Bihler and Crane, 1962; Bihler et al., 1962; Csaky, 1961; Riklis and Quastel, 1958). Glucose absorption is inhibited by ouabain, digitalis, and other cardiac glycosides that are also inhibitors of Na+-K+-ATPase activity and Na+ transport (Csaky and Hara, 1965; Schultz and Zalusky, 1964). These observations demonstrate the close relationship between the transport of glucose and Na+. FIGURE 14-5 Model of a Na+-activated glucose carrier of the intestinal brush border. From Wright and Peerce, 1985). c. Characteristics of the Na+-Glucose Transporter (Carrier) The concentrative step in the active transport of glucose occurs at the brush border membrane, and energy for this process is derived from an electrochemical Na+ gradient (Schultz, 1977; Schultz and Curran, 1970). Under conditions of net influx, Na+ and glucose enter in a ratio of 1:1 (Goldner et al., 1969; Hopfer and Groseclose, 1980). Cotransport of glucose and Na+ involves a membrane transporter or carrier that is believed to be a 75-kd polypeptide (Wright and Peerce, 1985). Na+ activates glucose transport primarily by increasing the affinity of the carrier for glucose. A model showing two hypothetical forms of the glucose carrier is presented in Figure 14-5. A galent channel or pore mechanism has been proposed in which the glucose binding site is located within the membrane. The translocation of glucose in this model is believed to be the result of a Na+-induced conformational change in the transporter (Semenza et al., 1984). The initial step in protein digestion is the enzymatic hydrolysis of peptide bonds by proteases with formation of smaller peptides and amino acids. The endopeptidases hydrolyze peptide bonds within the protein molecule and also hydrolyze certain model peptides. Exopeptidases hydrolyze either the carboxy-terminal (carboxypeptidase) or the amino-terminal (aminopeptidase) amino acids of peptides and certain proteins. Thus, a mixture of exopeptidases and endopeptidases cleaves long chain polypeptides from the ends as well as within the length of the chain resulting in sequentially shorter and shorter polypeptide chains and amino acids. Dietary proteins first come in contact with proteolytic enzymes in the stomach. The best known of the gastric proteases is the family of pepsins (Samloff, 1971), which hydrolyze most proteins with the exception of keratins, protamines, and mucins. Pepsins are relatively nonselective and hydrolyze peptide bonds involving many amino acids, the most readily hydrolyzed of which involve leucine, phenylalanine, tyrosine, and glutamic acid. The extent of proteolysis in the stomach depends on the nature of the dietary protein and the duration of time the protein remains in the stomach. The food bolus mixed with saliva has a neutral or slightly alkaline pH as it enters the stomach, and a period of time is required for it to mix with gastric secretions and become acidified. Proteolytic digestion begins when the pH of the gastric contents approaches 4 and occurs optimally in two pH ranges, 1.6 to 2.4 and 3.3 to 4 (Taylor, 1959a, 1959b). Because of the relative lack of specificity of the pepsins, some peptide bonds of almost all dietary proteins are split during passage through the stomach. The gastric phase of protein digestion may have a minor and possibly dispensable role in overall protein assimilation (Freeman and Kim, 1978), but the reservoir function of the stomach contributes to the gradual release of nutrients, ensuring more efficient utilization in the small intestine. Partially digested peptides pass from the stomach to the duodenum, where the acidic contents are neutralized by sodium bicarbonate present in bile and pancreatic juice. Peptic activity persists in the duodenum only during the period required to raise the pH above 4. The major peptidases that are active within the lumen of the small intestine are the pancreatic enzymes trypsin, chymotrypsin, elastase, and carboxypeptidases A and B. The action of these enzymes is integrated so that the endopeptidases produce peptides with C-terminal amino acids, which then become substrates for the exopeptidases. Trypsin produces peptides with basic C-terminal amino acids that are particularly suited for the action of carboxypeptidase B. Chymotrypsin produces peptides with aromatic amino acids in the C-terminal position, and elastase produces peptides with C-terminal amino acids that are nonpolar. Carboxypeptidase A hydrolyzes both types of C terminal peptide bonds (Table 14-4). The final steps in peptide digestion are associated with mucosal epithelial cells. Almost all of the aminopeptidase activity is associated with the mucosa, and very little activity is present in luminal contents. Mucosal aminopeptidase activity is located both in the cytosol and in the brush border membrane fractions of the epithelial cell (Heizer and Laster, 1969; Kim et al., 1972). These physically separate enzymes have remarkably different substrate specificities (Kim et al., 1974). The brush border enzyme has more than 50% of the activity for tripeptides yet less than 10% of the total activity for dipeptides relative to the cytosolic enzyme(s) (Kim et al., 1972; Peters, 1970). Almost all activity for tetrapeptides is present in the brush border (Freeman and Kim, 1978). Proline-containing peptides are hydrolyzed almost exclusively by cytosolic peptidases, whereas leucine aminopeptidase activity is located primarily in the brush border. The brush border peptidases appear to have digestive functions similar to the disaccharidases and oligosaccharidases of the brush border. Endopeptidase activity of the intestinal mucosa is associated primarily within the lysosomal fraction of the cell. Despite the long interest in the subject of this section, the relative amounts of the various protein digestion products (i.e., peptides versus amino acids) that are actually absorbed by intestinal mucosal cells during normal digestion remain problematic. It is a difficult process to investigate because the products of proteolysis are absorbed rapidly after they are formed and, therefore, studies of luminal contents give only an estimate of the overall rate of protein digestion. Equally important, dietary protein is continually mixed with endogenous protein in the form of digestive secretions and extruded mucosal cells. Most endogenous proteins are hydrolyzed and the amino acids absorbed in a manner similar to that of dietary protein, and the two processes occur simultaneously. Endogenous protein accounts for a significant part of the amino acids of the intestinal contents. Even when dietary protein is labeled with a radioactive tracer, there is such rapid utilization that the tracer soon reenters the lumen in the form of endogenous protein secretion. In adult mammals, protein is not absorbed from the intestine in quantities of nutritional significance without previous hydrolysis. Most neonatal animals absorb significant amounts of immunoglobulin and other colostral proteins, but this capacity is lost soon after birth. The intestinal mucosa, however, is not totally impermeable to large polypeptide molecules. The absorption of insulin (MW 5700; Danforth and Moore, 1959; Laskowski et al., 1958), ribonuclease (MW 13,700; Alpers and Isselbacher, 1967), ferritin, and horseradish peroxidase (Warshaw et al., 1971) has been demonstrated. During the digestion of protein, the amino acid content of portal blood increases rapidly, but attempts to demonstrate parallel increases in peptides in the portal blood have not been uniformly successful. This has been regarded as evidence that only amino acids can be absorbed by the intestinal mucosa and that the absorption of peptides does not occur. Although it seems clear that most dietary protein is absorbed by the mucosal epithelium in the form of free amino acids, peptides also may be taken up by the mucosal cell in quantitatively significant amounts. Peptides so absorbed may be hydrolyzed either at the cell surface or intracellularly, and individual amino acids finally enter the portal circulation via the basolateral cell membrane. Small peptides, under certain circumstances, may cross the intestinal epithelium intact and enter the portal circulation. Webb (1986) suggested that intact peptide absorption accounted for more than half of luminal amino acid nitrogen in the calf. The amount of peptide nitrogen entering the portal circulation in other species characteristically has been lower and variable depending on the source of protein and on the digestibility of the peptide being investigated (Gardner, 1984). Amino acids, like glucose and certain other monosaccharides, are absorbed and transferred to the portal circulation by active transport processes. The same type of saturation kinetics observed in studies of monosaccharide absorption are observed with amino acids, which suggests the presence of carrier transport mechanisms. Certain monosaccharides inhibit amino acid transport (Newey and Smyth, 1964; Saunders and Isselbacher, 1965), and whereas inhibition generally has been of the noncompetitive type, competitive inhibition between galactose and cycloleucine has been demonstrated (Alvarado, 1966a), which suggests that a common carrier may be involved. Most amino acids are transported against concentration and electrochemical gradients, and the overall transport process requires metabolic energy. The chemical specificity of these transport mechanisms is shown by the fact that the natural l-forms of various amino acids are absorbed more rapidly than the corresponding d-forms and that only the l-amino acids appear to be actively transported. For most transport systems, Na+ is necessary for absorption of amino acids as it is for a variety of other nonelectrolyte substances (Gray and Cooper, 1971; Schultz and Curran, 1970). Separate transport systems exist for different groups of amino acids. Each member of a group inhibits the transport of other members competitively, suggesting that they share the same carrier. There is demonstrable overlap between groups, indicating that the overall transport process is complex (Christensen, 1984, 1985; Stevens et al., 1984). The following is a summary of the designations and substrates of the recognized amino acid transport systems of the intestinal brush border (Stevens et al., 1984): 1. The neutral brush border (NBB) pathway is responsible for monoaminomonocarboxylic (neutral) amino acids and histidine. Na+ is required, and these amino acids show mutual competition for transport. 2. The monoaminodicarboxylic acids (aspartic and glutamic acid) pathway (XGA) requires Na+. Aspartic and glutamic acids are not transported against concentration gradients. Following uptake, they are transaminated by the intestinal mucosa and under physiological conditions enter the portal vein as alanine. 3. Imino acids (IMINO), proline, hydroxyproline, methylaminoisobutyric acid, and N-substituted glycine derivatives sarcosine (N-methyl glycine) and betaine (N-dimethylglycine). This pathway also has a Na+ requirement. 4. Dibasic amino acids (Y+), including lysine, arginine, ornithine, and the neutral amino acid cystine. 5. Phenylalanine and methionine share the PHE amino acid transport system. The ν-glutamyl cycle has been proposed as a possible transport system for amino acids (Meister and Tate, 1976). ν-Glutamyltransferase (GGT) is a membrane-bound enzyme that is present in a number of mammalian tissues and catalyzes the initial step in glutathione degradation. The 0-glutamyl moiety of glutathione is transferred to amino acid (or peptide) receptors with the production of cysteinylglycine: The highest GGT activity is present in tissues that are known to transport amino acids actively (e.g., the jejunal villus, the proximal convoluted tubule of the kidney and liver). Meister and his colleagues (1976) have suggested that GGT may function in translocation by interaction with extracellular amino acids and with intracellular glutathione. The hypothetical mechanism involves the noncovalent binding of extracellular amino acids to the plasma membrane, whereas intracellular glutathione interacts with GGT to yield a ν-glutamyl-enzyme complex. When the ν-glutamyl moiety is transferred to the membrane-bound amino acid, a ν-glutamyl-amino acid complex is formed, which, when released from the membrane binding site, moves into the cell. The ν-glutamyl-amino acid complex is split by the action of ν-glutamyl cyclotransferase, an enzyme appropriately located in the cytosol. Glutathione is regenerated by means of the ν-glutamyl cycle, which is a good substrate for GGT (Thompson and Meister, 1975). The ν-glutamyl cycle does not require sodium, and the cycle would not explain the previously demonstrated sodium dependence for amino acid transport. The cycle is not considered to be the only amino acid transport system, and its quantitative significance in individual tissues is unknown. At birth, most domestic species, including the calf, foal, lamb, pig, kitten, and pup, absorb significant quantities of colostral protein from the small intestine. Immune globulin (Ig) either is absent in the serum of domestic species at birth or the level is low. Within a few hours after ingestion of colostrum, the serum Ig levels rise. This represents the principal mechanism by which the young of most domestic animal species acquire maternal immunity. Under normal environmental conditions, ingestion of colostrum is an absolute requirement for health during the neonatal period. The rabbit is the exception in that maternal Ig is received primarily in utero by transplacental transfer. Protein enters the neonatal absorptive cell by pinocytosis and passes through the cell to the lymphatics. The process is not selective because many proteins other than Ig can be absorbed (Payne and Marsh, 1962). The ability to absorb intact protein is lost by domestic species soon after birth. In the piglet, “closure” occurs within 1 to 2 days (Leary and Lecce, 1978; Westrom et al., 1984) beginning in the duodenum and occurring last in the ileum. In rodents, protein absorption normally continues for approximately 3 weeks. The mechanism of intestinal “closure” was studied, and researchers found that complete starvation of pigs lengthened the period of protein absorption to 4 to 5 days, whereas early feeding shortened the period (Lecce, 1965; Lecce and Morgan, 1962; Leece et al., 1964). Feeding different fractions of colostrum including lactose and galactose resulted in loss of protein absorptive capacity. The route of feeding may not be the critical factor, however. Calves that are prevented from eating but that receive nutrients parenterally lose the ability to absorb protein at the same time as control calves (Deutsch and Smith, 1957). In the neonatal calf, Ig deficiency resulting from a failure of colostral Ig absorption plays a role in the pathogenesis of Gram-negative septicemia (Gay, 1965; Smith, 1962). Most calves deprived of colostrum develop septicemia early in life and may develop acute diarrhea before death (Smith, 1962; Tennant et al., 1975; Wood, 1955). Hypogammaglobulinemia is almost always demonstrable in calves dying of Gram-negative septicemia and is the result either of insufficient Ig intake or of insufficient intestinal absorption. The Ig fraction is the essential factor in colostrum that protects against systemic infections (Penhale et al., 1971). Serum immunoglobulin values of neonatal calves vary, and a 10% incidence of hypogammaglobulinemia may occur in clinically normal calves (Braun et al., 1973; House and Baker, 1968; Smith et al., 1967; Tennant et al., 1969; Thornton et al., 1972). Most such individuals probably have insufficient colostrum intake. Even when calves were given the opportunity to ingest colostrum, however, a surprising number were hypogammaglobulinemic. Some of the reasons for varying gammaglobulinemia values are recognized, but the relative importance of each is not known. The concentration of lactoglobulin, the volume consumed (Selman et al., 1971), the time elapsed from birth to ingestion of colostrum (Selman et al., 1971), and the method of ingestion (natural suckling versus bucket feeding) may have an important influence on the serum IgG (McBeath et al., 1971; Smith et al., 1967). Calves that suckle their dams usually attain serum IgG concentrations that are higher than those attained by calves given colostrum from a bucket. The frequency of hypogammaglobulinemia may be influenced by season (Gay et al., 1965b; McEwan et al., 1970a), although this relationship is not consistent (Smith et al., 1967; Thornton et al., 1972). Familial factors also may influence development of hypogammaglobulinemia (Tennant et al., 1969). Regardless of cause, the mortality of hypogammaglobulinemic calves is higher than that of calves with normal serum IgG levels (Boyd, 1972; Gay, 1965; House and Baker, 1968; McEwan et al., 1970a; Naylor et al., 1977; Thornton et al., 1972). In addition to having more septicemic infections (Gay, 1965; McEwan et al., 1970a; Roberts et al., 1954; Smith, 1962; Wood, 1955), hypogammaglobulinemic calves have a greater prevalence of acute diarrheal disease (Boyd, 1972; Gay et al., 1965a; Naylor et al., 1977; Penhale et al., 1970), which indicates that the local protective effects of Ig in the intestine are important (Fisher et al., 1975; Logan and Penhale, 1971). The prevalence of hypogammaglobulinemia and the high mortality associated with it has led to the development of several rapid tests for identification of hypogammaglobulinemic calves (Aschaffenburg, 1949; Fisher and McEwan, 1967b; McBeath et al., 1971; Patterson, 1967; Stone and Gitter, 1969). The zinc sulfate turbidity test (Kunkel, 1947) was the first to be used to determine the serum immunoglobulin concentrations of neonatal calves (McEwan et al., 1970b). A close correlation has been established between test results and the amount of serum IgG and IgM (Fisher and McEwan, 1967b, 1967b; McEwan et al., 1970b). The sodium sulfite turbidity test is similar to the zinc sulfate test and also has been used to identify hypogammaglobulinemic calves (Pfeiffer and McGuire, 1977; Stone and Gitter, 1969). Failure of turbidity to develop when serum is added to a saturated solution of sodium sulfite indicates immunoglobulin deficiency. A semiquantitative assessment of the Ig concentration is made by grading the degree of turbidity (Stone and Gitter, 1969). The refractometer is used as a rapid test for Ig deficiency (Boyd, 1972; McBeath et al., 1971). There is a close relationship between the concentration of IgG and total serum protein (TSP) in neonatal calves (Tennant et al., 1969), and the wide variations in TSP were due to variations in IgG. Direct linear correlation between the refractive index (RI) and the Ig concentration has also been observed (McBeath et al., 1971). The regression line for this relationship was independently confirmed (Tennant et al., 1978). The Y intercepts in these studies were identical (4 g/dl). The refractometer has a value as a rapid field instrument for the assessment of Ig status, but in cases of hemoconcentration it has limitations (Boyd, 1972). The glutaraldehyde coagulation test was used originally in cattle to detect hypergammaglobulinemia in samples of whole blood (Sandholm, 1974). Glutaraldehyde has also been used in a semiquantitative test to evaluate IgG in canine (Sandholm and Kivisto, 1975) and human serum (Sandholm, 1976). This procedure has been modified to detect hypogammaglobulinemic calves. Calves with a negative test result (serum IgG #0.4 g/dl) had markedly higher mortality than calves with positive results (Table 14-7) (Tennant et al., 1979), which is similar to results obtained by using the zinc sulfate turbidity test (Gay et al., 1965a; McEwan et al., 1970a) or other estimates of circulating IgG. Many tests can be initiated quickly using the glutaraldehyde coagulation test, and results can be evaluated rapidly without instrumentation. TABLE 14-7 Relationship between Results of the Glutaraldehyde Coagulation Test, Serum (-Globulin) Concentration, and Death Rate a. Luminal Phase The fat in the diet is primarily in the form of triglycerides or long-chain fatty acids. In the dog, gastric lipase plays a credible role in fat digestion, leading to the formation of fatty acids, which help coordinate gastric emptying and pancreatic secretions. In other species, the initial step in utilization of triglycerides occurs in the lumen of the proximal small intestine, where hydrolysis is catalyzed by pancreatic lipase. The pancreas secretes lipase in active form. The enzyme requires an oil-water interface for activity, so only emulsions of fat can be hydrolyzed. Enzyme activity is directly related to the surface area of the emulsion, so the smaller the emulsion particle, the greater the total surface area of a given quantity of triglyceride and the greater the rate of hydrolysis (Benzonana and Desnuelle, 1965). Bile salts are not an absolute requirement but favor hydrolysis by their detergent action, which causes formation of emulsions with small particle sizes and by stimulating lipase activity within the physiological pH range of the duodenum. A colipase is present in the pancreatic secretion, which facilitates the interaction of lipase with its triglyceride substrate and protects lipase from inactivation (Borgstrom and Erlanson, 1971). Pancreatic lipase splits the ester bonds of triglycerides preferentially at the 1 and 3 positions so that the major end products of hydrolysis are 2-monoglycerides and free fatty acids. Both compounds are relatively insoluble in water but are brought rapidly into micellar solution by the detergent action of bile salts. The mixed micelles so formed have a diameter of approximately 2 nm and are believed to be the form in which the products of fat digestion are actually taken up by the mucosal cell (Hofmann and Small, 1967). The intraluminal events that occur in fat absorption are schematically summarized in Figure 14-6. FIGURE 14-6 Intraluminal events during fat absorption. From Isselbacher (1967). b. Mucosal Phase The initial step in intestinal transport of fat is the uptake of fatty acids and monoglycerides by the mucosal cell from micellar solution. The precise mechanism is yet unclear, but present evidence suggests that the lipid contents of the micelle are somehow discharged at the cell surface and enter the mucosal cell in molecular rather than micellar form (Isselbacher, 1967). The net effect is the absorption of the end products of lipolysis and the exclusion of bile salts, which are absorbed farther down the intestine, primarily in the ileum. Uptake of fatty acids appears to be a passive process having no requirement for metabolic energy. FIGURE 14-7 Biochemical reactions involved in intestinal transport of long chain fatty acids and monoglycerides. From Isselbacher (1966). Within the mucosal cell, the fatty acids are transported by a soluble binding protein to the endoplasmic reticulum, where the fatty acids and monoglycerides are rapidly reesterified to triglyceride (Ockner and Isselbacher, 1974; Ockner and Manning, 1974). The two biochemical pathways for triglyceride biosynthesis in the intestine are summarized in Figure 14-7. Direct acylation of monoglyceride occurs in the intestine and is the major pathway for lipogenesis in the intestine during normal fat absorption. The initial step in this series of reactions involves activation of fatty acids by acyl-CoA synthetase, a reaction that requires Mg2+, ATP, and CoA and that has a marked specificity for long-chain fatty acids. This specificity explains the observation by Bloom et al
I. INTRODUCTION
II. SALIVARY SECRETIONS
A. Mechanisms of Secretion
B. Composition of Saliva
1. Mucus
2. Electrolytes
3. Amylase
4. Lipase
C. Functions of Saliva
III. GASTRIC SECRETIONS
A. Composition of Gastric Secretions
1. Basal Versus Stimulated Secretion
2. Pepsin
3. Gastric Lipase
4. Rennin
5. HCL
B. Control of Gastric Secretion
1. General
2. Gastrin
3. Histamine
4. Prostaglandins
IV. BILIARY SECRETIONS
A. Composition of Bile
B. Properties of Bile
C. Synthesis of Bile Acids
D. Enterohepatic Circulation of Bile Acids
V. EXOCRINE PANCREATIC SECRETIONS
A. Composition of Pancreatic Juice
1. Electrolyte Composition
2. α-Amylase
3. Proteolytic Enzymes
4. Lipase
B. Control of Pancreatic Secretions
1. Hormonal Control
VI. OTHER GASTROINTESTINAL HORMONES
A. Motilin
B. Somatostatin
C. Enteroglucagon
VII. DIGESTION AND ABSORPTION
A. Water and Electrolytes
1. Mechanisms of Mucosal Transport
2. Sodium and Chloride Absorption
3. Potassium Absorption
4. Water Absorption
B. Carbohydrate Digestion and Absorption
1. Polysaccharide Digestion
2. Disaccharide Digestion
3. Monosaccharide Transport
C. Proteins
1. Enzymatic Hydrolysis
2. Absorption of Proteolytic Products
3. Transport of Amino Acids
4. Neonatal Absorption of Immunoglobulin
D. Lipids
1. Absorption of Fats
Stay updated, free articles. Join our Telegram channel
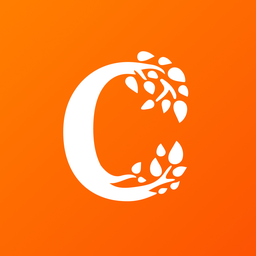
Full access? Get Clinical Tree
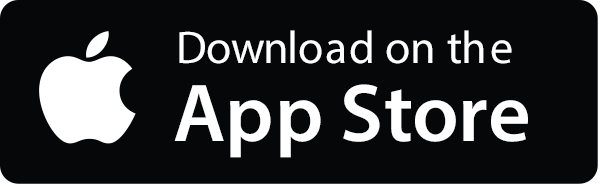
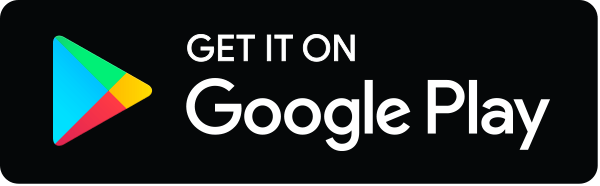