Fig. 1.
Small animal MRI scanners available from various companies. (a) shows a refurbished 200 MHz 40 cm bore Oxford NMR system with passive shielding inside a Faraday cage. The scanner is lower in cost than actively shielded and new systems, but can be used for a variety of applications in rodents and small primates. (b) shows a 200 MHz 40 cm bore system from Magnex Scientific of similar dimensions. This system is actively shielded and contains a shielded front and back panel door to reduce MR field fluctuations. Both systems in (a) and (b) are filled with cryogens on a weekly basis. (c)shows an actively shielded 300 MHz Bruker USR 20 cm bore system with cryogen recapture system and coldhead for zero helium boil-off. This system also has a bed (not shown) that is used to move the RF coil along with the animal to adjust positioning inside the system.
Table 1
Listing of equipment and analysis tools for small animal imaging studies. The purpose of the listing is to serve as a beginner’s reference for researchers interested in small animal MRI methods
Essential item(s) | Vendor list and world wide web information |
---|---|
−Radiofrequency coils for animal MR experiments −Beds with manual or automated adjustment used to position RF systems inside the scanner −Mouse and rat cradles and head restraint devices, and options for adding on physiological monitoring and stimulation | Doty NMR (http://www.dotynmr.com/) |
M2M Imaging (http://www.m2mimaging.com/) | |
Rapid MR International (http://www.rapidmri.com/) | |
InsightMRIa (http://www.inslsystems.net/) | |
Bruker BioSpin (http://www.bruker-biospin.com/) | |
Agilent Technologies (http://www.agilent.com/) | |
−Physiological monitoring and stimulation equipment and accessories | BIOPAC Systems (http://www.biopac.com/) |
SA Instruments (http://www.i4sa.com/) | |
−Gradient Coils | Resonance Research Inc (RRI) (http://www.rricorp.com/) |
Bruker BioSpin | |
Agilent Technologies | |
−Preclinical MRI Scanners | Bruker BioSpin |
Agilent Technologies | |
−Analysis software | FSL—Free Surfer Limited (http://www.fmrib.ox.ac.uk/fsl/index.html) |
SPM—Statistical Parametric Mapping (http://www.fil.ion.ucl.ac.uk/spm/) | |
AFNI—Analysis of Functional NeuroImages | |
MIVA—Medical Image Visualization and Analysis |
The use of 4.7 T and 7 T horizontal bore systems for rodent applications have been optimal for our experimental applications both because of the high SNR and good T2/T2* contrast for functional studies. There are higher field scanners now used frequently for fMRI studies in rats and mice, such as 9.4 T and 11.7 T systems. Scanners with high-quality spatial encoding gradients, automated or manual shimming (for correcting small field variations around the brain), and preinstalled pulse sequence routines that run on user-friendly console software are of choice for many applications-driven laboratories. Most animal imaging centers will use MRI methods in rodents of various sizes and small and large primates.
Among the important considerations upon purchasing a MRI for animal research are the clear bore size and different gradient sets with different inner bore diameters, which would allow applying the methods to small and larger animals. Typical clear bore sizes are 30–60 cm with internal gradient sizes of 12–20 cm. Another factor to consider is bore length. Many 9.4 T and 11.7 T scanners have long bores that may present different challenges when planning to present stimuli. The length of the bore in the longitudinal z-direction may be quite large, reaching 160 cm to isocenter (center of the magnetic field of the scanner where the sample is placed for fMRI studies). This is more than arm’s length, therefore setting up RF coils, centralizing animals, and ensuring that stimulus apparatuses are in place require a bit more ingenuity. It is important to work closely alongside vendors to ensure that everything needed to overcome these minor issues is included in the purchase of the scanner, especially if these are high field systems.
At high fields, it is possible to obtain an in-plane voxel resolution for functional scans of about 390–469 μm2 with 12–20 coronal slices (1–1.2 mm slice thickness). This covers most of the rat brain from the olfactory bulb to the cerebellum using T2-weighted fast spin echo sequences with minimal anatomical distortions. For localized rat brain studies with fewer slices, focusing on the coordinated activity of a few subsets of areas, the in-plane resolution can be increased to 100–250 μm2. Many fMRI studies have been performed using gradient echo echo planar imaging (GE EPI) because of its greater sensitivity to magnetic susceptibility and the BOLD effect. Gradient echo sequences use rapidly changing MR gradients to excite protons into the transverse plane (rather than using RF pulses). Because of the same magnetic susceptibility that contributes sensitivity to the BOLD effect, the GE EPI is highly vulnerable to signal loss at air–tissue interfaces in the temporal and paranasal regions. This leads to loss of data in important areas, such as the ventral hippocampus, amygdala, and medial and orbital regions of the prefrontal cortex (15, 16). GE EPI has a high sensitivity to physiological noise and shows anatomical distortions (spatial warping) that can produce alignment and registration errors. Most modern MR console software contains built-in algorithms that correct these distortions. For instance, Varian’s VmrJ 3.1 “epip” sequence collects phase maps between repetitions that can be used to correct spatial warping in echo planar imaging (EPI). However, to correct the spatial warping, additional scan time must be added to acquire field maps that aid in unwarping image data upon reconstruction. Finally, GE EPI sequences are more sensitive to intravascular and extravascular large vein signals that are distant from the actual foci of activity (17). Spin echo EPI at high fields are more sensitive to intra- and extravascular compartments closer to the capillaries and therefore are commonly used for fMRI studies at higher field strengths (17). At high fields, T2-weighted spin echo sequences appear to suffer less from the aforementioned issues (17–20). Single shot spin echo sequences (SE EPI) and multisegmented T2-weighted fast spin echo can be used successfully with rats. The latter has been the sequence of choice for many of our experiments in awake rats. There is support in the literature for the use of fast spin echo and SE EPI sequences for BOLD imaging (17–20).
2.2 RF Coils
Studies of the rat brain using MR scanners require the use of RF coils that serve as the source of the B1 field (the 90 and 180° pulses) that excite water protons in tissue to the transverse planes. The RF coils also serve to detect longitudinal and transverse signal relaxation. There are varieties of coils that are used for neuro-applications. Figure 2 shows examples of RF systems. Table 1 lists some current vendors.
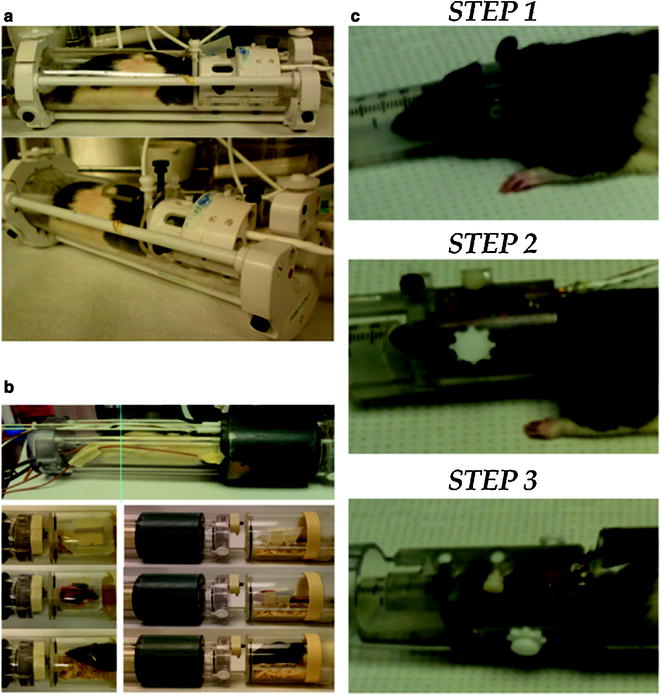
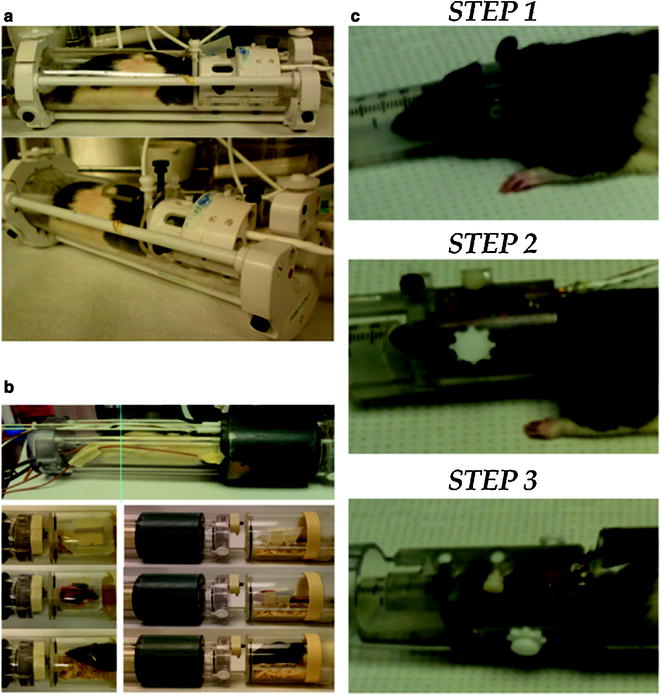
Fig. 2.
Radiofrequency electronics for imaging awake rats. (a) shows a quadrature (volume) coil system capable of transmitting and receiving tissue RF signals. (b) shows a dual coil (volume transmit and surface coil receive) system. (c) shows the steps of a rat setup procedure. Step 1 shows the placement of ear bars with lateral grooves for positioning inside the head restrainer. Step 2 shows the animal positioned inside the head restrainder. Screws are used to affix the animal in place. These are guided into the lateral grooves of the ear bars. In Step 3, the animal is in its final position before being placed into the magnet. The nose bar and lateral ear bars are in place and the animal is positioned inside a body tube.
Many laboratories design and construct their own RF coils (21–23). Some of the RF coils are as simple as single copper wire loops to more advanced actively decoupled surface receive/volume transmit dual coil systems tuned to the magnet frequency (4.7 T–7 T or 200–300 MHz range, respectively). The coil is aligned over or focused upon the area of interest, such as over the entire animal head or overlying the cortical area of interest. The loop coil configuration, however, has less spatial coverage and usually results in signal drop from dorsal to ventral areas of the brain that makes this type of configuration less favorable for developmental studies. The configuration prohibits coverage of signals from brain structures such as the hypothalamus and midbrain that are farthest in distance from the coil. This can be overcome by using a dual RF coil system built into an MR compatible restrainer of the head and body (16), or a quadrature coil system with improved B1 coverage of the brain (InsightMRI, Shrewsbury, MA) (Fig. 2; Table 1).
2.3 Accessory Equipment
In addition to the main electronics that are needed to run functional brain scanning in rats, there are other useful accessory devices (Table 1). For anesthetized preps, beds with integrated head and/or body holders are important to place animals correctly inside the bore of the magnet. Typically, these are necessary to align animals correctly within the isocenter of the MR-spectrometer prior to image acquisition. Physiological monitoring devices are also an essential part of the animal imaging setup. This includes MR compatible temperature probes, pulse oximeters, capnometers, electroencephalographic (EEG) and electromyographic (EMG) recorders, respiratory pillows and transducers, and other devices according to the needs of the investigator. The physiological measures are used to trigger the image acquisitions to remove respiratory and cardiac pulsations that appear as low-frequency oscillations (24–26). Stimulation devices may be needed when evoking sensory responses, such as for whisker and forepaw stimulations. The stimuli for sensory evoked responses can be timed to the functional image acquisitions for accurate correlations with BOLD signal responses during block design studies.
2.4 Anesthetized Preparation
Anesthetized preparations are used extensively for fMRI studies in rats. The methods used generally are not suitable for longitudinal studies in the same population of animals. In many applications, the femoral artery of the rat is catheterized to allow the sampling of arterial blood gases, and a close monitoring of arterial blood pressure and pH during scanning. Changes in the partial pressures of blood gases may be indicative of alterations of basal conditions that can alter the magnitude of the BOLD signal. This is important since hypoxia and hypercapnia modulate baseline BOLD signal in the rat, perhaps independently of basal neural activity and metabolism (27, 28). Controlling for movement is also important and some laboratories use chemical agents that can suppress muscle contractility during scanning. Marota et al. (29) used the paralyzing agent pancuronium to eliminate unwanted respiratory pulsations in the anesthetized rat. Others have used the muscle relaxant gallamine to paralyze animals during MR scanning (30). The animals in the cited studies were tracheostomized and mechanically ventilated during experiments (29). The experimenter-controlled activity facilitates removal of movement artifacts. The invasive procedure precludes long-term developmental studies in rats. Alternatively, arterial blood pressure and respiration rates can be measured noninvasively using a pulse oximetry over the tail of the rat and a respiratory pillow placed just underneath the animal’s chest (Table 1).
2.5 Awake Setup
Our laboratory has utilized methods to image awake rats as an alternative to imaging under anesthesia. Before this is done, however, animals must be acclimated to the restraint conditions and MR pulse sequence noise. The procedures for acclimation are carried out for 5 days prior to collection of imaging data. Both acclimation and actual imaging experimental setup procedures are done under similar conditions. Rats are first anesthetized under 2–4% isoflurane gas to enable placement into a head restrainer. There is evidence that isoflurane anesthetized animals regain motor function and coordination within minutes (31), and thus, volatile anesthetics such as isoflurane are useful when quick setup and awakening are desired. An important part of the restraint setup is the ear bars that allow the proper orientation of the head. A semicircular plastic headpiece containing blunted ear bars is first placed over the animal’s head and fitted into the ear canals. These are noninvasive (requiring no surgery) and are not made of abrasive or harmful material. Their placement is the same as standard stereotaxic ear bars. Other laboratories permanently affix holders to the skull of the animals to ensure that the animals will not be able to move during scanning (32–34). The animal is guided through the center of the coil/head holder unit and the incisors placed over a bite bar. A plastic latch locks down over the nose with a screw. The lateral ear bars contain outer grooves that accommodate lateral screws that are used to align the animal in the holder and fix its position in the restrainer. The body is placed into a tube that has shoulder bars and an overlying square plastic peg that prevents up-and-down movement during scanning. The entire system is placed into a chassis that fits the bore of the magnet and can be fastened inside of it. In the experience of the author, the setup time is quite short (∼10 min). The system has plenty of room to accommodate accessory equipment for stimulus delivery or physiological monitoring.
The above system allows the rodent to remain in a semicrouched position while being scanned (forelimb movement is more restricted). Most movement comes from the y direction (up and down movements). The rats seldom move in the back-and-forth (z) and side-to-side (x) if positioned correctly. The design of the newer coil system used in our laboratory (35) (Fig. 2) minimizes the y direction movement. Other groups have used positioning screws that are affixed to the skull and have obtained good results. For example, Desai and coworkers (32) carried out fMRI-optogenetic experiments in awake restrained mice. The mice had miniature plastic screws affixed to the skull. They used a short (3-day) acclimatization period and provided animals with “treats” after restraint sessions. It is possible that both approaches will yield good results, and using cranial fixtures to prevent movement may be preferable for methods that include additional invasive procedures during fMRI scanning.
2.6 Data Processing and Analysis
Motion must be minimized during MR scanning and residual motion should be corrected whenever possible. There are several preprocessing steps we have used over the years, some qualitative and others more stringent. These are done without a priori knowledge of outcomes, which would bias the procedure. Both gross movements (for example, slow shifts in head position, sustained or transient leg motion, chewing, vocalizations) and physiological motion (pulsations due to cardiac and respiratory cycles) can significantly degrade multirepetition MR scans. They can also contribute to false activation patterns that correlate motion with stimulus presentations (36). One qualitative strategy is to generate movies of multi-repetition fMR scans and visually assess excess motion. We have done this in past work using Stimulate (http://www.cmrr.umn.edu/stimulate/) (37). Motion is detected across frames, and this provides a reasonable ability to detect permanent shifts in head position, and frequent movements during scanning. Recently, Ferris and co-workers generated artificial motion in MR images and assessed the amount of movement necessary to induce false activation patterns across the rat brain (38). Using the simulations, they were able to provide a numerical threshold for movement, above which motion is in excess and cannot be corrected. Scans above the threshold are discarded from studies. Animals included in the study are processed using standard Statistical Parametric Mapping software (SPM8; http://www.fil.ion.ucl.ac.uk/spm/). The latter corrects translational motions in x–y–z planes and rotational shifts. Signal drift also occurs in MRI scans and can contribute to false positive and negative signal changes. A simple voxel-wise or image-wise correction can be used. Drift correction uses square root minimization to fit time data to a straight line (using linear regression). This can be done in each voxel independently, which is more computationally intensive, or across the entire image volume. The reasoning behind the image volume correction is that drift due to electronics, temperature change, and physiological noise is distributed across the imaging volume; therefore the correction can be applied throughout the image plane.
Following prescreening and preprocessing procedures, individual scans are further processed using Medical Image Visualization and Analysis (MIVA; see Table 1 for other options). There are other software packages available, and some are more intuitive and user-friendly than others, depending on the knowledge of programming in UNIX language required. The program we have used does not require programming skills, although there are several standardized steps involved that are specific to our applications. Other programs are more “open-ended” and thus require programming skills to adjust the software to the specific MRI study at hand. Table 1 provides a short list of MRI software that has been used in small animal MRI studies. Many are freely available packages. Not all have electronic atlases of mouse and rat brains, and therefore this is researcher supplied. Most of the programs can import data in NIFTI (.nii) data format. See websites for specific details.
Using MIVA, each subject is registered to a fully segmented electronic rat brain atlas (39, 40) (Fig. 3). The program allows alignment, segmentation and pixel-wise analysis for signal intensity changes in step-by-step modules that have been developed and incorporated into the main software (Fig. 3). Statistical t tests are performed on each subject within the original coordinate system. We determine the repetitions to be included as “baseline” where no stimulus is provided and a stimulus epoch of equal repetitions. Statistical t tests used a 95% confidence level, two-tailed distribution, and heteroscedastic variance assumptions. In order to provide a conservative estimate of significance, a false-positive detection-controlling algorithm is introduced into the analysis (41). This ensures that the false-positive detection rate is below our confidence level of 5% (42). Statistically significant pixels are assigned their percentage change values (stimulus mean minus control mean). Activated voxel numbers and percent signal changes are exported to statistical software to assess the presence of significant effects. For example, the number of voxels per region of interest (ROI) and their corresponding average percent change values can be statistically evaluated between 4 scan groups using Kruskall–Wallis analysis of variance (ANOVA p < 0.05). Independent variables will depend on the specific study design used.
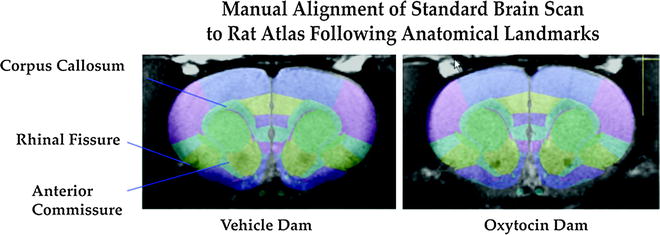
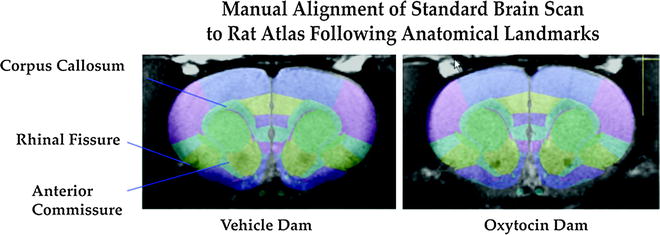
Fig. 3.
Example anatomical reference image alignment to a digital atlas of the rat brain. Landmarks are used for optimal alignment. The rat atlas is part of the software package (Medical Image Visualization and Analysis, MIVA; ccni.wpi.edu).
3 Anticipated Results and Notes
3.1 Cocaine-Induced Changes in BOLD Signal
The work in our laboratory has partly focused on studies of the effects of psychoactive substances in rats. There has been significant previous work employing in vitro methods assessing cerebral glucose metabolism following acute and repeated cocaine exposure (43–46). Since the rewarding and psychomotor properties of cocaine are attributed to changes in neuronal and synaptic activity within mesocortical and mesolimbic systems (47, 48), we used fMRI to investigate the neural actions of cocaine in awake animals (15). Prior to this experiment there were several human functional imaging experiments investigating changes in brain activation following intravenous cocaine administration (49–51); however, experiments seeking to understand the developmental events leading to an addicted state cannot be studied in humans. Animal studies carried out in anesthetized rats are hampered by the use of general anesthetics (29, 52) (see Sect. 3.4. below). We used spin EPI in conscious rats at 4.7 T following an intracerebroventricular injection of cocaine (20 μg) in artificial cerebrospinal fluid (10 μL). Within 5 min of injection, there was a significant increase in BOLD signal intensity in the substantia nigra, ventral tegmental area, nucleus accumbens, dorsal striatum, and prefrontal cortex, as compared to vehicle controls. Minimal negative BOLD signal changes were observed in response to cocaine, and there were no significant perturbations in normal cardiovascular and respiratory function. The findings demonstrated the technical feasibility of studying psychostimulant-induced brain activity using functional MRI in conscious rats.
The results using BOLD fMRI corroborate findings from previous animal studies. Metabolic mapping of radiolabelled deoxyglucose showed cocaine-induced, site-specific glucose utilization in the multiple areas of the brain (43, 53). In a follow-up study the repeated effects of cocaine were examined using the same methods. Seven days of pretreatment with cocaine significantly reduced the BOLD response to the drug. Although the lower BOLD response to cocaine appeared to be a generalized and nonspecific effect, several brain areas of acutely and repeatedly treated rats did not show differences in BOLD signal intensity (namely, the dorsal prefrontal cortex, cingulate, and somatosensory cortex). In addition, the lower BOLD response was not associated with differences in cerebrovascular reactivity between the two treatment groups, as measured by brief exposure to hypercapnia. One explanation proposed for the decreased BOLD response observed is that it might be associated with the previously observed decreases in glucose metabolism (46) and could also be related to reductions in basal and cocaine-stimulated synaptic monoamine concentrations (54–56). Alternatively, the reduced BOLD response could be due to differences in basal cerebrovascular reactivity.
To investigate changes in neural activity with repeated cocaine administration that may contribute to sex differences, we studied BOLD changes by fMRI in ovariectomized rats with and without circulating sex steroids (57). Our results indicate that cocaine-sensitized females having artificial treatments with estrogen show higher BOLD activity when reexposed to cocaine, particularly in frontal cortical areas (57). In contrast, rats without the steroid show a similar BOLD response to acute or repeated cocaine exposure. An exception was the hippocampus of OVX rats, which showed decreased neural activity compared to the response recorded on the first day of exposure. These results contrast with those obtained in males. Male rats treated with a sensitizing regime of cocaine showed less positive BOLD activation as compared to drug-naïve rats receiving cocaine. This was observed for the volume of activation and percent change in BOLD. Interestingly, these findings suggest a tolerance-like effect in male rats that would otherwise show a sensitized behavioral response. It is possible that the sex differences observed may reflect temporal sex differences. Another potential explanation for the findings using fMRI is that the basal state for either CBF or baseline neuronal firing has been modified (58, 59). PET images from detoxified male cocaine addicts show decreased CBF in basal ganglia when presented with a cocaine video compared to their response to a non drug video (60). Interestingly, females addicted to cocaine show enhanced CBF in several brain areas when exposed to cocaine, compared to males (61). These results provide evidence of gender differences in the neural response to drugs of abuse, as measured using fMRI in awake female rats.
3.2 Imaging the Maternal Neural Response to a Natural Reward: Lactational Studies in Rats
A separate series of experiments in awake lactating rats has been carried out using the methods described above. Many of these have focused on the neural processing of the natural suckling stimulus from pups as a rewarding stimulus (42, 62–64). It has been reported that suckling stimulation from pups modulates the expression of maternal behaviors in rats by promoting arched back nursing postures (65, 66) and slow-wave sleep (67, 68). The fMRI technique was used to map the cortical pattern of activity during suckling, and the stimulus was compared to artificial suction in the absence of pups and mechanical stimulation on the ventrum skin (64). During the processing of somatosensory stimuli, information coming from the landscape of peripheral sensory receptors underlying body skin surface is relayed to the cortex through the spinothalamic pathway and topographically represented in the cerebrum. In the case of the mammillae, primary afferent fibers terminate in the ipsilateral dorsal root ganglia between spinal segments C5 and L6 (69), with afferent relays along the lateral cervical nucleus, the dorsal column nuclei, and the sensory and spinal portions of the trigeminal complex (66, 70). In contrast with studies using c-fos assays that provide exquisite cellular spatial detail (71–75), the detection of real-time brain activity during the actual act of nursing is limited by the very wide temporal window (usually taken 60–120 min post-stimulus). Findings from electrophysiological recordings taken from neurons in the somatosensory cortex of the anesthetized rat indicate that the receptive field for the ventrum skin surrounding the nipple area doubles in size during the lactation period (76). It was found in the fMRI study that wide areas of the postpartum rat cerebrum exhibit an increase in the fMRI BOLD signal during suckling stimulation, suggesting that neural activity is modified over wide areas of the cerebral cortex in response to a rather specific stimulus (64). The artificial suckling stimulus caused a similar degree of cortical activation. Therefore, although auditory, olfactory, and nonsuckling tactile stimulation from pups may contribute to cortical activity, the suckling itself is fully capable of causing a widespread cortical response. There have been experiments examining the patterns of brain activity in mothers presented with infant sensory cues, but as of yet, none have investigated the effects of the lactational stimulus. Our rat studies suggest that there would be significant cortical activation not only in limbic cortical divisions, as reported previously (77), but also in areas that might correspond to long-term memory storage. These are cortical representations of the suckling stimulus that might be important for the maternal–infant bond during the early lactational period.
3.3 Comment on Interpretations and Comparisons with Existing Literature
One should be cognizant of the biological underpinnings contributing to the BOLD signal in order to interpret fMRI results. As in the original Ogawa et al. work, a greater O2 tension resulted in increased local signal intensity, which is what is measured in most fMRI studies (1). In the cocaine BOLD activation experiment and the lactation study cited above, one assumes that the BOLD signal changes are predominantly related to changes in neuronal activity and not only due to changes in blood flow or volume alone. Differences in percent changes in BOLD between the different experimental conditions are expected to represent differences in neuronal activity of comparable magnitude.
There has been other significant research in awake animals that has primarily focused on investigating the neural actions of pharmacological agents (78), investigations of the differences in the hemodynamic response function in awake versus anesthetized rats (79), cerebellar-dependent motor learning though eye-blink conditioning in the rabbit (33), functional connectivity studies of the awake rat brain (80, 81), combined examination of sensory neural processing in specific circuits using fMRI, and optogenetics in awake mice (32), awake Rhesus macaques, and marmoset monkeys (82–84). The studies support the use of awake fMRI methods in neuroscience research and have been performed using a variety of elegant and creative custom procedures that will not be discussed here. In general, all involve head restraint and many used some form of animal training prior to studies. Miller et al. (33) used the rabbit model in their studies of eye-blink conditioning. There were changes in cerebellar BOLD signal responses during progressive conditioning trials that closely matched patterns of electrical activity during learning. Desai et al. recently used optogenetic methods that pair the virally mediated expression of photorhodopsin in glutamatergic neurons of the barrel field cortex to study light-stimulated increases in neuronal activity in the somatosensory cortex of the awake mouse during fMRI (32). Light-induced increases in neuronal activity produced BOLD signal responses in the barrel field region that were comparable to activation evoked by whisker deflection. The combined use of fMRI and optongenetics is now a major approach in the investigation of the functional roles of specific neural circuits.
3.4 Potential Shortcomings and Methodological Challenges
3.4.1 Effects of Anesthetics on Basal Neuronal Firing and Hemodynamic Mechanisms
Agents typically used for anesthetizing animals can suppress certain forms of neuronal activity and modify specific patterns of neuronal activity and metabolism. In addition to these actions, anesthesia can influence global cerebrovascular reactivity. The choice of anesthetic and calibration of the depth of anesthesia are therefore important. There are differences in basal and stimulated brain glucose utilization (cerebral metabolic rate for glucose (CMRglu)) and CBF in awake versus anesthetized rats (85). Stimulation of the whisker-to-barrel cortex pathway, for example, resulted in differential CBF and CMRglu across regions when rats were anesthetized with halothane (85). The results of the latter study suggest that although the barrel cortex is active in the anesthetized state, other regions along the pathway arising from the stimulation of peripheral sensory receptors to the cortex are suppressed and may thus require a conscious state (85). Evoked potentials in the barrel field cortex have been shown to vary between anesthetic conditions (79). The amplitudes of field potential responses to graded levels of repetitive electrical stimulation to the whisker pads are reduced to a greater degree in anesthetized vs. awake rats (79). The magnitude of neuronal activity and the specificity of activity of neural networks are hampered by anesthetics. Firing of action potentials over localized regions of the awake rat visual cortex showed higher frequencies and bursting, but lower pair-wise correlations between single units than ketamine-anesthetized rats (86). This suggests that the propagation of action potential in localized networks is modified by the induction of an anesthetized state. Halothane, isoflurane, and desflurane can differentially affect gamma band oscillations in the rat cortex (87, 88). This is important because field potential power has been correlated to BOLD signal changes (10). Graded levels of isoflurane (1.8–2.2%) also suppress EEG bursts measured in the primary sensory cortical area representing the forelimb of the rat and also reduced spontaneous variations in CBF (89). These levels of isoflurane are within the range that causes suppression of bursting in sensory cortical EEG patterns (90). Research on the role of anesthetic agents in modulating neuron activity raises concerns about the use of deep levels of anesthesia for rat brain imaging experiments (91–93). Variations in the pattern and magnitude of neuronal activity will vary according to anesthesia type and concentration.
The effects of volatile anesthetics on the BOLD signal, CBF, and CBV have also been investigated. Hypercapnia-induced BOLD signal changes, which occur in the absence of neuronal activity, are of much greater magnitude in awake rats (94). This suggests that cerebrovascular reactivity is affected by anesthesia. It is also important to note that basal levels of O2 metabolism and neuronal spiking frequency in the cortex are reduced by deep levels of alpha-chloralose (58). Larger magnitude changes in BOLD may reflect lower basal firing of neurons in animals that are deeply anesthetized whereas lighter levels of anesthesia allow for smaller magnitude changes in BOLD in the face of higher basal activity (58). Basal CBF levels in 2% isoflurane anesthetized rats were observed to be greater than in the awake state (95). Isoflurane anesthesia can act as a vasodilating agent that increases blood flow. The percent change in CBF and BOLD in response to CO2, however, is lower in anesthetized rats (95). The lower magnitude response could be due to higher basal levels of blood flow (95). Thus, in isoflurane anesthetized animals there seems to be direct modulation of CBF that is independent of the effects on neuronal activity (93). Isoflurane reportedly increases CBF globally due to its vasodilating actions (89). Spontaneous CBF changes are suppressed by increasing levels of isoflurane from 1.8 to 2.2% (89). Light sedation with isoflurane (<1.8% in inspired air) might minimize the effects described above. It has also been reported that alpha-chloralose-specific parameters for forepaw-stimulated BOLD activity in the somatosensory cortex do not work under isoflurane anesthesia (93). The accumulating evidence again underscores the importance of considering the effects of anesthetics on both neuronal activity and cerebrovascular reactivity when designing fMRI studies and interpreting the data.
< div class='tao-gold-member'>
Only gold members can continue reading. Log In or Register a > to continue
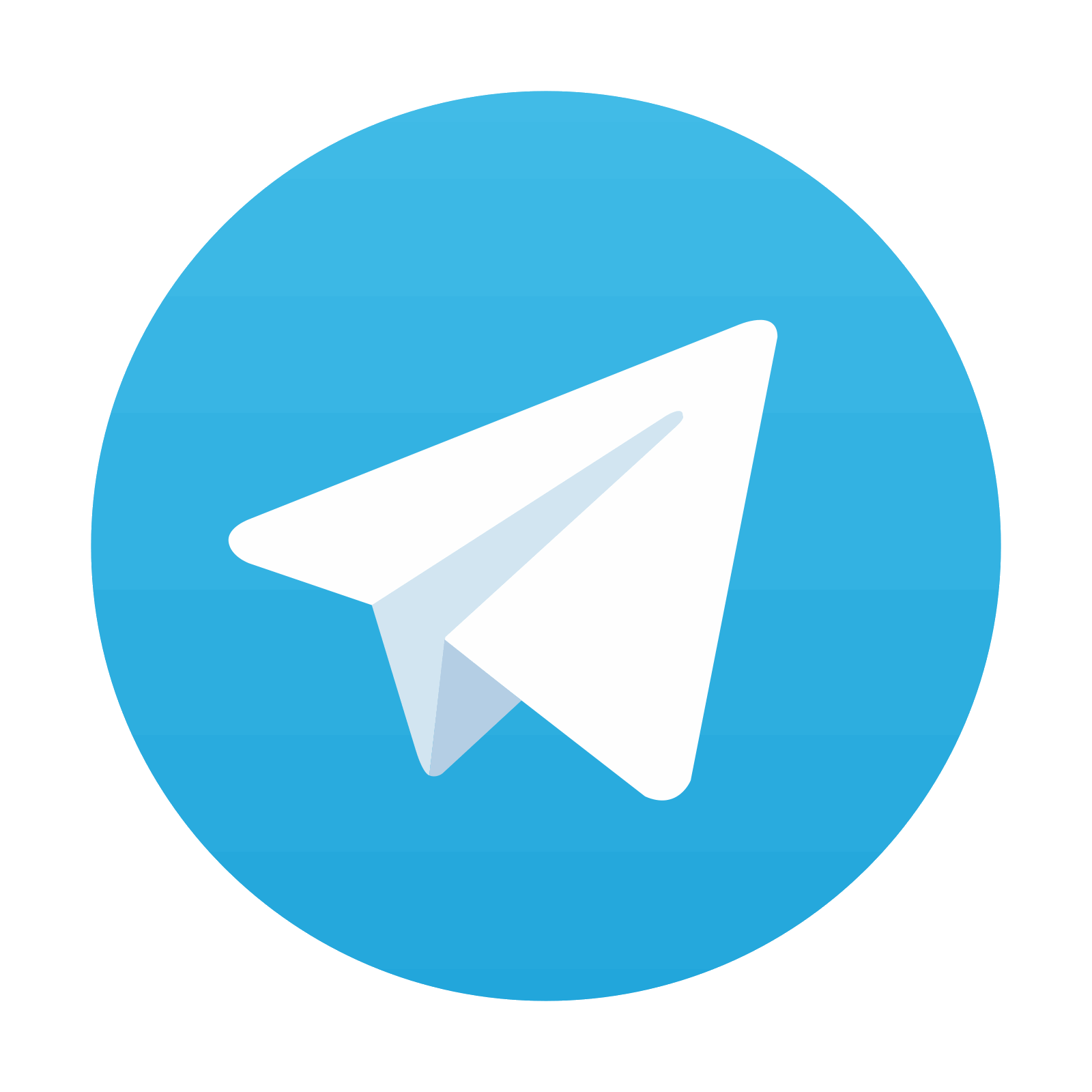
Stay updated, free articles. Join our Telegram channel
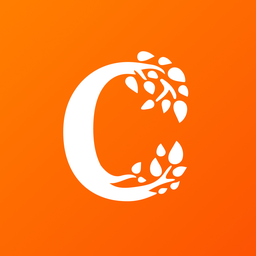
Full access? Get Clinical Tree
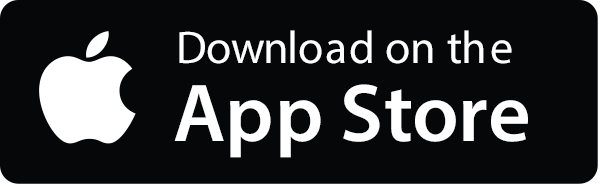
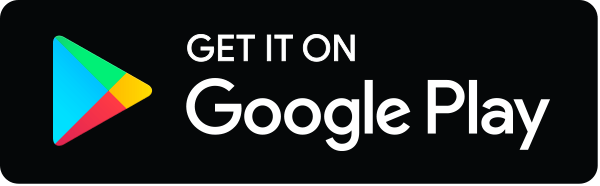