Fig. 1.
Female Wistar rats were housed in running wheel cages and had ad libitum access to chow and water (filled line, N = 8) or were subjected to an activity-based anorexia (ABA) model (dotted line, N = 8) with 1-h access to food at the onset of the dark period. (a) depicts average running wheel revolutions (averaged per hour per group) during the 4 days of the experiment. Rats were sacrificed at the start of the dark period of day 5. In (b) represents the body temperature rhythms (averaged per hour per group) of rats during ad libitum feeding or ABA.
Thus, the increase in locomotor activity preceding access to food is driven by FAA and starvation-induced hyperactivity (foraging). When food is given at the onset of the dark phase, there may even be increased locomotor activity due to anticipation of the dark phase, the period in which rats naturally start eating.
2.2 RFS
As mentioned previously, rats on a RFS have limited access to food and lack access to running wheels. Due to this intervention, circadian rhythms alter and FAA occurs (33–35). Rats are not the only species that exhibit FAA. FAA in response to a RFS has also been reported in various other species, including mice, fish, birds, hamsters, and rabbits (33). Several parameters determine the course of a RFS model.
Although the majority of RFS studies in rats have provided rats with food for 2 h in the light phase, FAA occurs under different conditions as well. Food availability in the dark period will also result in FAA (36, 37). However, as the dark period is their normal active period, the difference between FAA and “normal” activity is more difficult to distinguish than when food is offered during the light phase. Furthermore, FAA can be observed in rats that are housed under 24-h light (38–40) or 24-h dark (41) conditions.
The development of FAA depends on a diurnal schedule. Rats on a 12:12 h light:dark cycle did not show FAA when daily meals were separated by 19 h or 29 h (33, 42, 43). The range of daily mealtime intervals that will elicit FAA is thought to be 23–26 h (33). Nevertheless, the onset of FAA does not demand a single daily meal. Studies showed that rats anticipated two, but not three, meals per day, which were at least 5 h apart from each other (36, 44). Furthermore, durations of food access up to 12 h could induce FAA (45), although the amount of FAA was reduced with longer durations (46).
2.2.1 Experimental Set-up and Procedure
Male outbred Wistar WU rats are individually housed in a temperature- and humidity-controlled room under a 12:12 h dark:light cycle. They are allowed to acclimate under ad libitum food and water conditions. One week after arrival, rats receive transmitters as described in Sect. 2.1.1 in order to allow continuous measurements of general locomotor activity and body temperature. Following 2 weeks of recovery, rats are subjected to a RFS. On the first day, food is removed at zeitgeber time (ZT) 8, i.e. 8 h after the lights come on. From then on, rats have daily restricted access to food from ZT6–ZT8. Body weight and water intake are measured just before ZT6. When body weight loss exceeds 20% or when body temperature is lower than 33°C, the experiment is terminated just before access to food.
At first, rats on a RFS will not be able to consume their baseline food intake during the 2 h of food access. However, as the model continues, they gradually increase their food intake. Rats eat on average 2–3 large meals of 5–6 g.
At the beginning of a RFS, rats will lose weight due to reduced food intake. As shown in Fig. 2b, weight loss stabilizes (47), but, to a large extent, the amount of weight a rat will lose depends on the amount of locomotor activity it performs. When rats have access to running wheels, hyperactivity will cause a rapid decline in body weight. On the other hand, when rats are housed in standard cages, body weight loss will not be severe, and rats have time to adjust to the RFS.
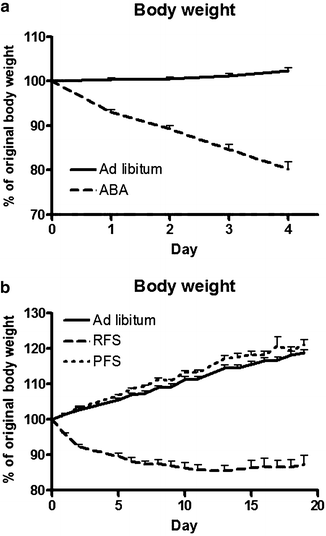
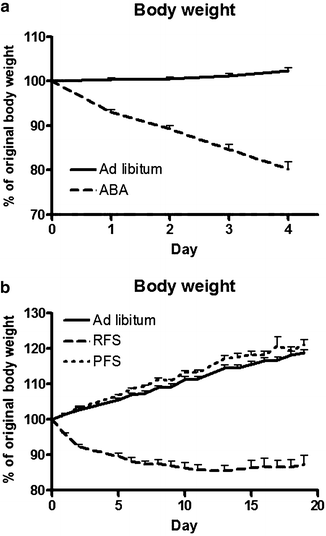
Fig. 2.
Relative body weight gain, expressed as percentage of original body weight, of rats subjected to an activity-based anorexia model (ABA) (a), restricted feeding schedule (RFS) or palatable feeding schedule (PFS) (b) compared with ad libitum fed rats.
In response to a RFS, rats will alter their rhythms of locomotor activity and body temperature (47–49). Anticipatory peaks of these parameters arise, and values of locomotor activity and body temperature are reduced during the dark phase (49). In general, FAA can be observed within 3 days of the onset of a RFS. Subsequently, the amount of FAA increases in amplitude and duration in the course of RFS (33). FAA usually ceases when rats are provided with ad libitum food access, and reestablishes when rats are food deprived (33, 50). An overview of the changes in circadian rhythms of locomotor activity and body temperature is provided in Fig. 3.
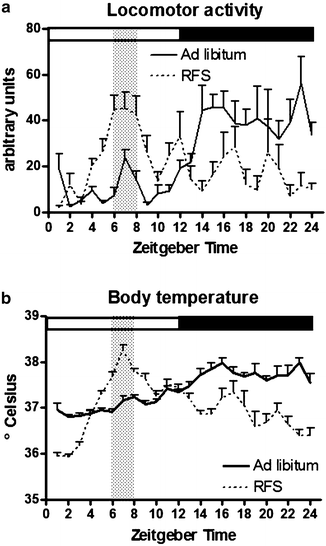
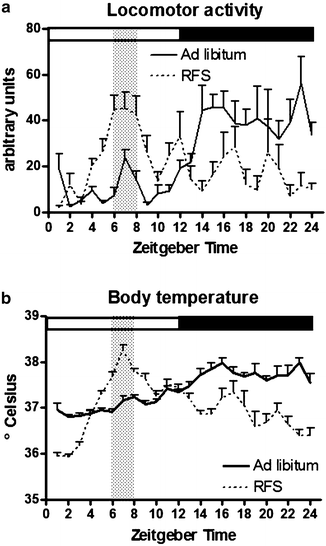
Fig. 3.
Measures of locomotor activity (a) and body temperature (b) (averaged per hour) of male Wistar rats (N = 6) were taken during ad libitum feeding (filled line) and when rats were subjected to a restricted feeding schedule (RFS, dotted line) with food available from ZT6–ZT8, as indicated by the grey vertical bar.
While ABA is a severe model mimicking features of AN, the RFS model can be maintained longer and is more suitable to identify neural mechanisms underlying FAA.
2.3 PFS
The development of anticipatory locomotor activity is not limited to animal models of food deprivation. Ad libitum chow-fed rats were shown to anticipate a daily palatable treat as well (51). Unlike the RFS model, rats anticipating a small palatable treat will not show an anticipatory increase in body temperature. However, due to the relatively large meal ingested in the middle of the light period, they will show postprandial hyperthermia, potentially diet-induced thermogenesis. In response to the palatable feeding schedule, rats will not shift their circadian rhythm of locomotor activity, unlike rats on RFS and ABA. However, a small anticipatory peak in locomotor activity can be observed in the hour preceding availability of the palatable treat (35, 50, 52, 53). Figure 4 depicts the changes due to a PFS in locomotor activity and body temperature.
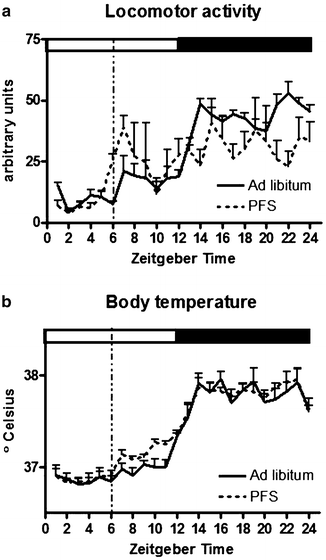
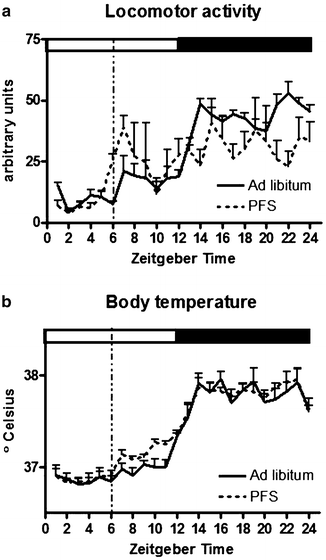
Fig. 4.
Measures of locomotor activity (a) and body temperature (b) (averaged per hour) of male Wistar rats (N = 6) were taken during ad libitum feeding and when rats were subjected to a palatable feeding schedule (PFS, dotted line) with 5 g of chocolate available at ZT6 in addition to ad libitum chow access, as indicated by the dotted vertical line.
The ability of a palatable meal to evoke FAA depends on several parameters. First, the palatable meal needs to have some nutritive value, since a palatable mash without caloric content did not induce anticipatory wheel running (51). Studies in rats indicate that carbohydrates, but not fat, have properties to induce a phase shift in the circadian food-entrained clock (54). Remarkably, FAA to a palatable treat in mice was only observed in males, and only when given a high-fat treat, but not a chocolate treat (55). In rats, chocolate (50, 52, 53), a palatable mash consisting of chow, vegetable oil, chocolate syrup and icing sugar (51), chocolate Ensure (56), and sucrose (57) have been used to evoke palatable meal-induced FAA.
Second, palatable meal size has to be reasonably large. The anticipated palatable meal was suggested to have to exceed a certain caloric threshold to be able to induce FAA, either in absolute value or relative to the total caloric intake (58). Access to a 32% sucrose solution resulted in FAA in 85% food-deprived rats, but not in ad libitum chow-fed rats (57). A 4 g palatable meal was able to induce FAA in only a minority of rats, whereas the majority of rats with a 2-h window of access to the palatable food, of which they consumed on average 9 g, exhibited FAA (51). This study used running wheel activity as a read-out parameter for FAA. Another study using this read-out parameter showed that only 37% of the rats anticipating a palatable treat exhibited FAA. However, studies that assessed FAA with general locomotor activity measurements, e.g., using infrared motion sensors, showed that 5 g of chocolate was able to evoke FAA in rats (50, 52, 53). Whereas FAA in rats on an ABA or RFS schedule started 2–3 h prior to mealtime, palatable meal-entrained rats showed a brief increase in anticipatory locomotor activity 15–30 min before access to the palatable snack (50, 53). Interestingly, once the palatable feeding schedule was discontinued and rats had ad libitum access to regular chow, FAA was still observed around palatable mealtime for several days (50).
2.3.1 Experimental Set-up and Procedure
Male outbred Wistar WU rats are individually housed in a temperature- and humidity-controlled room under a 12:12 h dark:light cycle. They are allowed to acclimate under ad libitum food and water conditions. One week after arrival, rats receive transmitters as described in Sect. 2.1.1 in order to allow continuous measurements of general locomotor activity and body temperature. Following 2 weeks of recovery, rats are subjected to a PFS. They have ad libitum access to normal rat chow and are, in addition, provided with 5 g of milk chocolate (Droste®, Vaassen, the Netherlands, per 100 g, 562 kcal, 35.4 g fat, 6.1 g protein, and 54.7 g carbohydrates) at ZT6. Body weight, chow, and water intake are measured just before ZT6.
Rats will decrease chow intake via a decrease in meal frequency in response to the extra palatable meal, especially during the light phase. When the palatable meal consists of a small chocolate bar (5 g), total caloric intake will increase slightly in the first week, but in the course of the palatable feeding schedule, total caloric intake will return to baseline levels. Others have shown as well that in free-fed rats, daily 2-h access to a dietary fat did not induce increased caloric intake (59). The body weight of the rats will not change compared to ad libitum chow-fed rats, as illustrated in Fig. 2b.
Altogether, rats anticipate a palatable treat, but to a lesser extent and at a later time of onset than rats anticipating a restricted meal. The caloric value of the palatable treat determines the amount of FAA that can be observed. Not all rats run in anticipation to a palatable treat, but general measures of locomotor activity seem to be sensitive enough to detect this form of FAA.
3 Notes
3.1 Assessing FAA
FAA can be assessed in various ways (34). Running wheel activity has been used in numerous studies and has several advantages. It is a self-reinforcing behavior (60) and provides a reliable, precise measurement of anticipatory locomotor activity. One has to take into account that rodents need to adapt to the running wheel before being put on a RFS, otherwise the running will become obsessive and rodents will run to death (30). Under ad libitum conditions, wheel running occurs almost exclusively during the dark period. Hence, the signal-to-noise ratio for increased activity during the light period is very good. On the down side, running wheel cages can be expensive and occupy more space than standard cages.
Measurements of general locomotor activity are also extensively used. They include noninvasive approaches such as tilt floors, infrared motion sensors, and photobeam systems, as well as transponders (which emit radiofrequency pulses) placed intraperitoneally. Recently, some debate arose on the validity of transponders versus infrared motion sensors to measure FAA (61–65). One study compared the ability to detect FAA of these two measurements and revealed identical locomotor activity patterns (66).
All these measurements have a worse signal-to-noise ratio than do running wheels, since general locomotor activity is not exclusive to the dark phase in ad libitum fed rats. Furthermore, these devices not only record locomotor activity, but also unrelated behaviors, such as grooming, which makes them less sensitive and precise than running wheels.
To improve the signal-to-noise ratio, nest boxes or dark pipes can be added to the home cage. Rats will seek shelter in their rest period, which reduces the background measurements of unrelated behaviors. However, when assessing FAA with running wheels or general locomotor activity, any kind of manipulation that interferes with locomotor activity might seem to reduce FAA. Others have resolved this issue by looking at food bin-directed behavior (67).
Intraperitoneal transponders require invasive surgery, but have the advantage that locomotor activity and body temperature can be assessed continuously, thereby providing additional information on the physiological state of the rat. Body temperature has been used previously as an indication of FAA. Since body temperature is strongly associated with locomotor activity (hyperactivity will result in an increase in body temperature), it is not an independent parameter. However, some studies have reported dissociated effects on anticipatory locomotor activity and body temperature (68, 69).
3.2 Assessing Underlying Mechanisms of FAA
Since there is not a clear consensus on the driving force of FAA, many studies seek to explore the underlying mechanisms of this behavior. Different strategies have been used to tackle this research question. Some of these techniques are described in this section.
3.2.1 Observational Approaches
The immediate early gene fos is frequently used as a marker of neuronal activity due to its low baseline levels and short half-life. Fos protein levels rise 60 min after the event that evoked neuronal activation. Hence, fos immunohistochemistry (IHC) of brain slices of a rat sacrificed at ZT6 reflect the neuronal activation at ZT5. To investigate whether the rhythmicity of a certain brain area is uncoupled from the suprachiasmatic nucleus (SCN)-driven circadian rhythm due to food entrainment, IHC of the clock genes Per1 and Per2 can be applied. Another way of examining potentially involved brain areas in FAA includes studying the uptake of 2-deoxyglucose as a marker for cerebral glucose utilization (70). Activation of brain areas can also be investigated with in vivo electrophysiology. This technique has an advantage over fos IHC, because neuronal activity of single neurons within a brain area can be measured directly over time, instead of one time point per rat. In addition, this approach can be combined with pharmacological interventions. Multiple unit activity has also been applied to record activity of a brain area during FAA (71, 72). The limitation of in vivo electrophysiological recordings is that only one brain area can be investigated per rat.
3.2.2 Interfering Approaches: Lesion, Genetic, and Pharmacological Studies
Genetic interference has been applied to study FAA. Several knockout mice have been examined to determine whether certain genes play a role in FAA. Unfortunately, knock out models are scarce in rats. Another disadvantage of knock out models is that the gene is lacking from development onward, thereby enabling compensatory mechanisms. Conditional knock out models in which genes can be switched off after development overcome this problem. Viral-vector-mediated knockdown or overexpression of genes enables the manipulation of local gene expression in rats.
The involvement of brain areas in the regulation of FAA has been intensively studied by lesioning or ablating brain areas. Lesions can be performed in multiple ways. First, the use of radiofrequency current as an electrolytic lesion will destroy all cells and passing fibers in a brain area. Second, neurotoxins, such as ibotenic acid, will destroy cells, but spare fibers of passages.
To determine whether a certain neurotransmitter or other signaling peptide is involved in the control of FAA, pharmacological manipulation has been applied. Acute or chronic administration of specific agonists or antagonists of a receptor can reveal the role of that receptor in FAA. Chronic application is achieved by daily injections or by an implanted osmotic mini-pump. Additionally, it is possible to distinguish central and peripheral effects by injecting via a canula in a brain area or ventricle intraperitoneally, subcutaneously, or intravenously. The involvement of specific neurotransmitters in FAA can also be investigated using intracerebral microdialysis (73). With this approach, the release of neurotransmitters and their metabolites in a brain area can be measured during FAA and food intake.
3.3 Underlying Mechanisms of FAA
Under normal conditions, daily circadian rhythms in all kinds of behaviors, such as locomotor activity, are entrained by the master clock of the brain: the SCN. This hypothalamic area receives direct input from the retina and synchronizes other brain areas and peripheral tissues to the light:dark cycle via neuroendocrine and autonomic output pathways (74–77). Rats are nocturnal animals and their feeding behavior is also coupled to the SCN-controlled circadian rhythm. Not only is food intake controlled by the circadian system, food intake can, in return, affect circadian rhythms. When access to food is restricted to a few hours in the light period (i.e., the normal resting phase for nocturnal animals), the circadian rhythm of behavior is disengaged from the central clock and cycles in relation to feeding time. Animals will develop hyperactivity preceding the feeding time, hence FAA when they would normally not be active (33–35). This FAA is reflected in several features, including general hyperactivity, exploratory behaviors, increased instrumental behaviors to obtain food, and food bin-directed behaviors. Moreover, the circadian rhythm of body temperature alters, as well as the rhythms of metabolic parameters, such as glucose, hormones, and free fatty acids (78, 79). Numerous studies aimed to identify the food entrainable oscillator (FEO), the brain structure or signaling pathway that drives FAA.
3.3.1 Involvement of Peripheral Regulation in FAA
The peripheral digestive system and the brain can communicate with each other to regulate FAA, as food intake might be a stimulus for this behavior. However, transsection of the vagus nerve, which innervates the peripheral organs and sends sensory input to the brain about the state of the organs, does not prevent the corticosterone shift observed during FAA (80). Subdiaphragmatic transsection of this nerve does not impact FAA measured by running wheel activity in SCN-lesioned rats (81). Moreover, disruption of nonvagal visceral input to the brain by intraperitoneal capsaicin injections does not hamper the development of FAA (82). Therefore, (para)sympathetic innervation is not likely to be the essential route of communication between the gut and the brain for the regulation of FAA. This implies that humoral signaling could play a role in the development of FAA. However, adrenalectomy, which prevents the secretion of corticosterone, does not attenuate FAA (83). In addition, diabetic rats with destroyed insulin-producing cells (84) and rats with a mutated leptin receptor (85) still exhibit FAA. Hence, to date, the pathway via which the brain and the periphery communicate to regulate FAA remains to be elucidated. A potential candidate is ghrelin signaling, since ghrelin levels rise prior to mealtime (86–88), and ghrelin receptor knockout mice show attenuated FAA (86, 89–91). The roles of leptin and ghrelin in FAA are discussed in more detail in Sect. 3.3.3.
3.3.2 Involvement of Neural Circuits in FAA
Hindbrain
Peripheral feeding-related input arrives at the brain at various locations. The gastrointestinal system sends information via the vagus nerve to the nucleus of the tractus solitarius (NTS) in the hindbrain. A blood brain barrier is absent at the nearby area postrema (AP), which enables the AP to detect humoral signals. Both the NTS and AP project to the parabrachial nucleus (PBN). Electrolytic and neurotoxin-induced lesions of the latter structure result in attenuated FAA as measured by food bin approach behavior (92). However, lesions of the AP (93) or NTS (94), the main inputs to the PBN, do not affect FAA. Fos immunoreactivity in these three brain areas is increased only after consumption of the anticipated meal, not during FAA (95).
Hypothalamus
The hypothalamus has been implicated in the homeostatic regulation of energy balance and autonomic behaviors, and is hence a logical candidate for mediating FAA in rats on a RFS. It has been demonstrated that dorsomedial hypothalamus (DMH), lateral hypothalamus (LH), tuberomammilary nucleus (TMN), and perifornical area (PeF) showed increased fos expression during FAA (49, 96–100). Per1 rhythms shifted or changed in DMH, arcuate nucleus (Arc), PeF, paraventricular nucleus (PVN), and ventromedial hypothalamus (VMH) (50, 101–103) in rodents on a RFS. Per2 rhythms shifted or changed in DMH, PVN, and VMH in rodents on a RFS (56, 101–103). On the other hand, rats subjected to PFS with restricted access to palatable food in addition to ad libitum access to chow did not show any hypothalamic increase in fos during FAA (52), and Per2 rhythms did not change in DMH (56). Per1 rhythms were shown to change in this paradigm in SCN, DMH, and PeF (50, 53).
The SCN, which contains the light entrainable oscillator, is located within the hypothalamus. In 1979, Stephan and colleagues demonstrated that this brain area is not the FEO, as SCN lesions did not impair FAA (83). Lesions of the PVN and LH did not attenuate FAA either (67). Although PVN lesions decreased FAA in general locomotor activity measurements, anticipatory food bin approaches were still intact (67). At first, ablations of the VMH seemed to attenuate or even abolish FAA (104, 105). However, later studies revealed that this effect was only transient and that FAA recovered eventually (106, 107). Additionally, a weak correlation between the size of VMH lesion and the reduction in FAA was reported (49). The Arc lacks a blood brain barrier, like the AP. Interestingly, lesions of the Arc induced by neonatal monosodium glutamate increased FAA (108).
A vivid discussion takes place in the literature on the role of DMH in FAA (49, 61, 62, 66, 109, 110). Electrolytic lesions of this brain structure did not impair FAA as detected by general locomotor activity and food bin-directed behavior (110). In contrast, neurotoxin-induced lesions, which spare passing fibers, were reported to attenuate FAA as measured by wakefulness, body temperature, and general locomotor activity (49). This was also observed in mice with a large mediobasal hypothalamic lesion, which included DMH (111). Differences between these studies include parameters assessed, lesion type, and cage configuration (e.g., use of dark pipes in cage). Replication of all parameters from the Gooley study (49), apart from type of lesion, by Landry and colleagues (109) still revealed no impact of DMH lesion on FAA (109). In two studies, FAA persisted after 48 h of food deprivation in DMH-lesioned rodents, indicating that the food entrainable rhythm is not impaired (103, 109). The discussion focuses on the correct way of assessing FAA and the best approach to lesion a brain area (61, 62, 66) and has yet to be resolved. Interestingly, a recent paper showed that DMH ablation diminished FAA. However, when in addition the SCN was lesioned, FAA returned. This suggests that the DMH has a role in silencing output from the SCN to permit FAA (99). In line with the modulating role of DMH in FAA, it was shown that DMH lesions might attenuate FAA to a daytime meal; but when food is provided at nighttime, FAA is intact (112).
Orexin neurons are predominantly located in the LH, and orexin is known for its orexigenic and arousal-stimulating properties. Orexin neurons are activated during FAA in rats expecting a chow meal and those that anticipate a palatable meal (113–116). Orexin knockout mice still exhibit FAA, although reduced or with a delayed acquisition (113, 116–119). Interestingly, the effect of the lack of orexin signaling on FAA seems to be dependent on the circadian phase, since anticipation to RFS in the light period was impaired to a larger extent than anticipation to RFS in the dark phase (113). However, specific ablation of orexin neurons in LH did not impair FAA (120).
Reward-Related Brain Areas
Food intake is not just a matter of balancing energy intake with energy expenditure, motivational and rewarding aspects of food influence consumption as well. Corticolimbic areas involved in the reward-related effects of food intake were shown to play a more important role in FAA in rats anticipating a palatable treat. During FAA in PFS rats, fos activation was increased in several corticolimbic areas, including the paraventricular nucleus of the thalamus (PVT), central amygdala (CeA), nucleus accumbens (NAc) core, NAc shell, and prefrontal cortex (PFC) (52, 53). In rats on a RFS, fos levels increased also in these brain areas, although to a lesser extent (52, 121, 122). In addition, circadian rhythmicity of these brain areas changed during FAA in rats on a PFS and a RFS, as observed by altered Per1 rhythms (50, 53, 121).
Although these brain areas were activated during FAA, lesioning studies did not implicate any corticolimbic area in the development of FAA. The PFC is suggested to organize adaptive autonomic alterations to an expected situation (123). Although lesions of the infralimbic cortex, which is part of PFC, prevented anticipatory and postprandial rises in body temperature, FAA remained unaffected (68). A similar effect, loss of anticipatory body temperature increase, but intact FAA, was observed after ablation of the pituitary (69). The PVT receives projections from the brainstem and hypothalamus and projects to corticolimbic and hypothalamic areas that play a role in the regulation of reward and arousal (124, 125). Lesioning of the PVT resulted in decreased FAA as measured by general locomotor activity (126). However, when examining food bin-directed behavior, rats with PVT ablations still showed robust FAA (127). Large lesions covering the hippocampus, involved in learning and memory, and a large part of the amygdala, implicated in emotional memory, did not prevent the development of FAA either (128). Discrepancies exist in the effects of NAc ablations on FAA. NAc receives dopaminergic input and could mediate increases in locomotor activity. One study determined FAA as food bin-directed activity and reported no reduction on FAA (128), whereas another study looked at general locomotor behavior and observed a reduction in FAA in NAc core-lesioned rats, but not in NAc shell-lesioned rats (122).
Taken together, the results of various lesion studies suggest that the regulation of FAA is distributed over a network of brain nuclei. When one node of this network is lesioned, FAA still persists, or is only temporarily attenuated.
3.3.3 Hormones and Transmitters Implicated in FAA
Leptin
The adipose tissue-derived hormone leptin circulates in the blood and enters the brain to provide information about body fat content (129). Leptin suppresses food intake and stimulates thermogenesis and locomotor activity, thereby increasing energy expenditure (130). At least part of this effect can be attributed to leptin signaling via its receptors in the hypothalamus, mainly the Arc (131). Leptin receptors have also been identified on dopaminergic neurons in the ventral tegmental area (VTA), a brain area which has been implicated in reward and locomotion (132).
ABA rats exhibit reduced leptin levels (73, 133, 134), and peripheral (8, 23) and central (32, 135) leptin treatment suppress hyperactivity in this model. Leptin might exert its effect on hyperactivity via the VTA, since administration of leptin in the VTA reduces hyperactivity in the ABA model (135), whereas knockdown of leptin receptors in the VTA results in increased food intake, hyperactivity, and higher sensitivity to palatable food (136). Leptin treatment in the ABA model not only reduces hyperactivity in the dark period, but also in the hours preceding food access, hence FAA (8, 32, 137). Plasma leptin levels are low preceding the anticipated meal and peak after food availability in rodents subjected to a RFS (138, 139). In line with the data obtained in ABA rats, AN patients suffer from low leptin levels, which correlate negatively with physical activity levels (23, 140, 141). Hence, a lack of leptin signaling could lead to hyperactivity and increased FAA.
Leptin-deficient ob/ob mice, show augmented FAA (142). However, mice do not exhibit the complete repertoire of FAA (118), which could be due to their general hypoactivity (118, 143). Thus, if a lack of leptin signaling elicits hyperactivity and increased FAA, this should also be represented under conditions of leptin resistance. Obese people (144, 145) and diet-induced obese rodents (146–149) have high leptin levels. However, they do not respond to these high levels of leptin by reducing food intake or increasing energy expenditure, meaning they could be considered as leptin resistant. Indeed, obese Zucker rats, which could also be considered leptin resistant because they possess a point mutation in the gene encoding the leptin receptor, still exhibited strong FAA to a RFS (85). Interestingly, rats on a high-fat diet, which have high leptin levels, showed attenuated FAA (150).
Altogether, this suggests high leptin levels reduce FAA. The lack of leptin signaling, as in Zucker rats, in ABA and RFS models might drive hyperactivity and FAA, probably via the VTA (151).
Dopamine
Dopamine is known for its involvement in the regulation of reward and motivation via the mesolimbic dopamine system (152). This system originates in the dopaminergic neurons of the VTA and substantia nigra, which projects to the NAc, which in turn projects to many other limbic areas. Ingestion of palatable food resulted in augmented dopamine release in the NAc (153, 154), which attenuates during food intake following habituation and is transferred to the food-signaling cue (155–158) in anticipation of the predicted reward. Dopamine may increase the motivational drive to forage food, which is expressed as FAA. Dopamine is thus a potential candidate for the regulation of hyperactivity and FAA in the RFS and PFS models, since local injections of dopamine in the NAc-induced locomotor activity (159). Moreover, disruption of dopaminergic neurotransmission in the NAc prevented nicotine and amphetamine induced increases in locomotor activity (160), suggesting that dopamine is an essential signal to increase locomotor activity.
Obese and AN individuals show alterations in reward signaling, especially related to dopamine. Those that are obese exhibit increased activation of brain areas involved in motivation and reward in response to pictures of high-caloric food (161). In addition, decreases in dopamine release and striatal dopamine receptor 2 (D2R) density were reported in obese individuals and rodents (162–165). AN patients show lower levels of the major dopamine metabolite homovanillic acid, even after recovery of the disease (166, 167). D2R polymorphisms have been associated with AN (168, 169). AN patients exhibited increased peripheral expression of the dopamine transporter (DAT), whereas D2R expression is decreased, accompanied by epigenetic dysregulation of DNA methylation of these genes (170). Furthermore, recovered AN patients exhibit increased D2R and D3R binding in the anteroventral striatum (171). Thus, in AN there is evidence for increased dopaminergic activity, whereas in obese people there may be decreased activity.
In mice exposed to the ABA model, D2R mRNA expression in the caudate putamen region is increased (172). Dopamine depletion reduces hyperactivity in restricted rats with periodic food access (173). This is in line with the finding that dopamine antagonism reduces hyperactivity in the ABA model, although levels of FAA remain stable (174). In addition, in ABA rats, dopamine release in the NAc increases during food intake, but not during FAA (73). This is in contrast to previously mentioned observations that dopamine is initially released upon food intake, but transfers to the food-signaling cue (anticipation). However, in these studies (73, 174), the ABA model lasted for a few days, which might be too short for this transfer to have taken place. Others have shown that dopamine antagonism or NAc ablation did not affect FAA in restricted rats anticipating normal chow (128, 175). However, dopamine antagonism in rats anticipating a palatable meal reduces FAA (175–177).
In summary, dopamine is suggested to play a role in the hyperactivity observed in the ABA and RFS models. Although its role in FAA is less clear, data indicate that dopamine signaling could be important for FAA, particularly in anticipation of a palatable food source. However, studies on the role of dopamine, where dopamine signaling is reduced pharmacologically or by lesions, are compromised by the role dopamine plays in general locomotor activity and in all motivational behaviors.
Ghrelin
Ghrelin is an orexigenic hormone secreted by the oxyntic cells of the stomach and subsequently released in the blood (178). Posttranslational modification by ghrelin O-acyltransferase (GOAT) is essential for ghrelin to bind the growth hormone secretagogue receptor 1a (GHS-R1a) (179, 180). Apart from stimulating growth hormone release (178), ghrelin acts as a potent stimulator of appetite when injected peripherally (181, 182) or centrally (181–186) and can cause an increase in fat mass (185). Plasma ghrelin levels are increased in AN patients and after fasting (187), while weight gain and obesity reduce plasma ghrelin levels (187, 188). The central effect of ghrelin on food intake is at least partly mediated via GHS-R1a on neuropeptide Y (NPY)/Agouti-related Protein (AgRP) neurons in the Arc of the hypothalamus (183, 189). In addition to the Arc, GHS-R1a is expressed in other hypothalamic regions such as the VMH, DMH, and in the VTA (190–192).
Ghrelin stimulates the consumption of and motivation to work for palatable food, probably via the VTA (184, 192–196). Furthermore, ghrelin induces locomotor activity and accumbal dopamine release via its presynaptic and postsynaptic binding to GHS-R1a in the VTA (192, 197, 198). Alcohol-, nicotine-, and cocaine-induced stimulation of locomotor activity and dopamine release in the NAc were prevented in GHS-R1a −/− mice and by GHS-R1a antagonism (199–201). Based on these studies, it has been found that ghrelin could play a role in FAA. Indeed, Plasma ghrelin levels showed entrainment to habitual meal patterns in humans and rats (87, 88, 202). Plasma ghrelin levels were increased in ABA and RFS rats, and correlated with FAA (86, 88, 134). Central administration of ghrelin increased FAA in RFS rats (90), whereas a GHS-R1a antagonist reduced anticipatory locomotor activity in ABA rats (86). Moreover, GHS-R1a −/− mice showed attenuated FAA in RFS and ABA models, without affecting general locomotor activity (86, 89–91). In contrast, ghrelin −/− mice exhibit normal levels of FAA (118, 203), which could suggest that ghrelin signaling does not play a pivotal role in FAA and that a still unknown ligand of GHS-R1a can modulate FAA via this receptor. Taken together, GHS-R1a signaling likely contributes to the development of FAA.
4 Conclusion
FAA reflects the physiological adaptation to restricted access to food in the ABA and the RFS models. Circadian rhythms in behavior and neuronal activation in hypothalamic areas uncouple from the light:dark cycle. In addition, altered plasma levels of hormones and increased expression of orexigenic neuropeptides signal hunger. In contrast, the PFS model elicits temporary increases in locomotor activity and arousal, without changing circadian rhythms in behavior or hypothalamic activation. As corticolimbic structures are activated during FAA in PFS rats, FAA might be mediated via these areas. The magnitude of FAA is less in the PFS than in the RFS or the ABA models, suggesting that FAA in the RFS and the ABA models involves hunger and motivational components, whereas FAA in the PFS model is mainly driven by motivational aspects.
However, to date, no brain area, hormone, or signaling molecule has been proven to be both essential and sufficient to mediate FAA. Therefore, a redundant, distributed network likely mediates this behavior. Lack of leptin signaling and increased ghrelin signaling (hence hunger) augment anticipatory behavior. Hunger signals arriving in the hypothalamus could be the initiator of anticipatory behavior in ABA and RFS rats. The dopaminergic VTA-NAc projection is a prominent candidate to execute FAA. As the hypothalamus is not activated during FAA in PFS rats, initiation of FAA is probably mediated via corticolimbic systems directly in this paradigm.
Further research is required to determine whether common regulatory pathways are involved in FAA in response to the RFS, ABA, or PFS models. In addition, it remains unknown whether and how the hypothalamus communicates to other brain areas to regulate FAA. Could this be via a LH (orexin)- VTA (dopamine)- NAc connection? Other areas of research needing further attention include: whether ghrelin is the endogenous ligand that drives FAA, or whether a still unknown ligand of GHS-R1a is involved; which brain area GHS-R1a is involved in FAA, within the hypothalamus or the VTA; and the possibility that different neural circuits mediate FAA due to the RFS, ABA, and PFS models. Answering these questions will help us understand the regulatory pathways that can shift our metabolism and circadian rhythms to the presence of food, and the neural circuitry that makes us ready to eat when starved or take a palatable snack when satiated.
Acknowledgements
The authors were supported by NWO Toptalent (MM) and the EU FP7-KBBE-2009-3-245009 (NeuroFAST) and FP7-KBBE-2010-4-266408 (Full4Health) (RAH).
References
1.
2.
Barsh GS, Farooqi IS, O’Rahilly S (2000) Genetics of body-weight regulation. Nature 404:644–651PubMed
3.
4.
5.
7.
8.
9.
Casper RC et al (1991) Total daily energy expenditure and activity level in anorexia nervosa. Am J Clin Nutr 53:1143–1150PubMed
10.
11.
12.
13.
14.
15.
16.
Cornell CE, Rodin J, Weingarten H (1989) Stimulus-induced eating when satiated. Physiol Behav 45:695–704PubMedCrossRef
< div class='tao-gold-member'>
Only gold members can continue reading. Log In or Register a > to continue
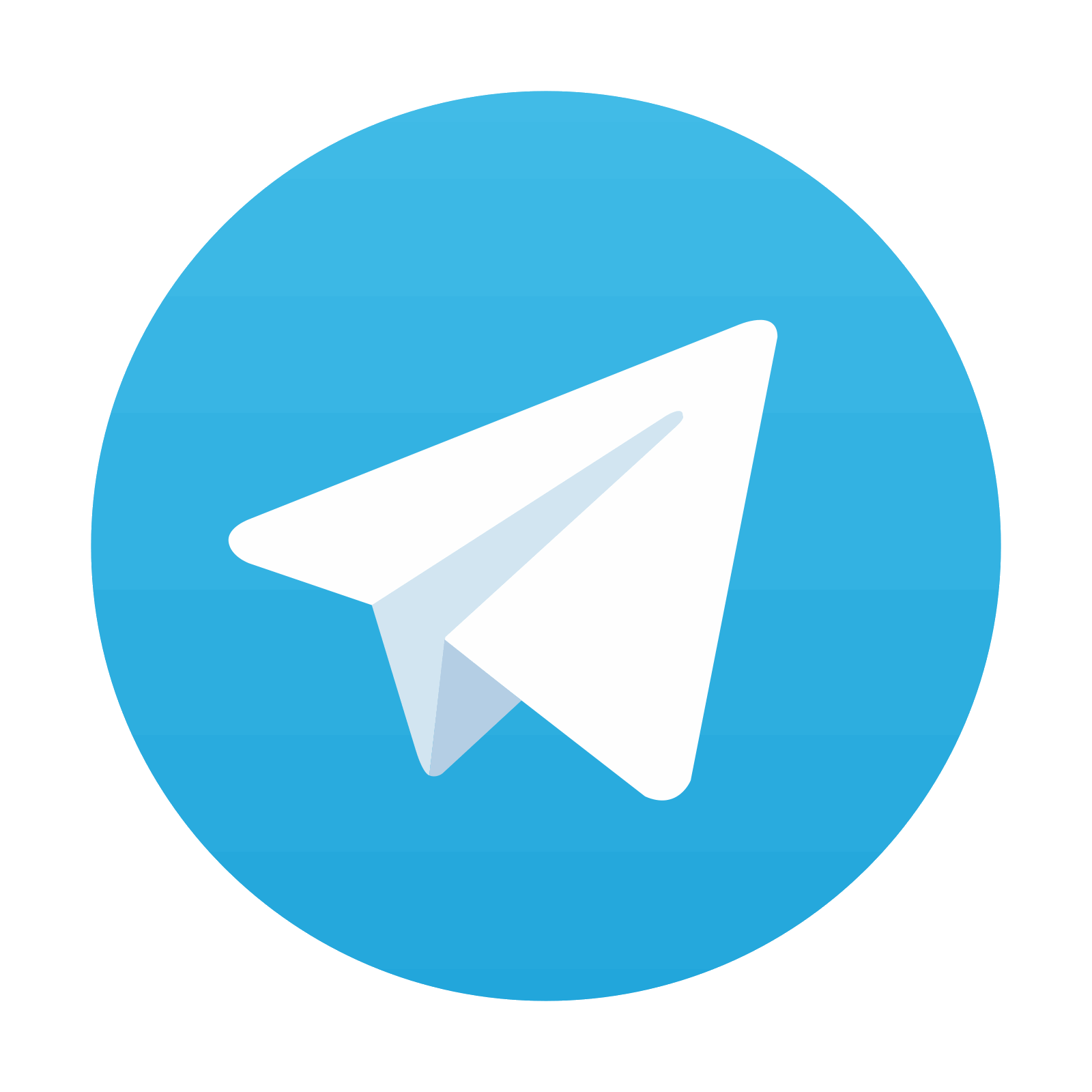
Stay updated, free articles. Join our Telegram channel
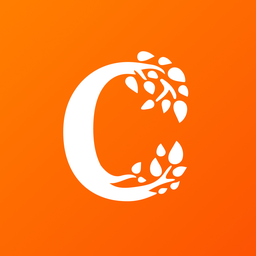
Full access? Get Clinical Tree
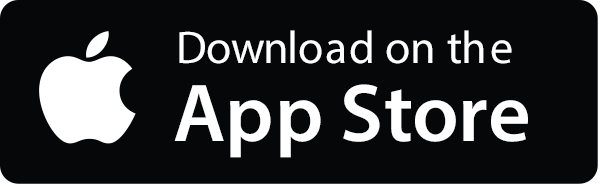
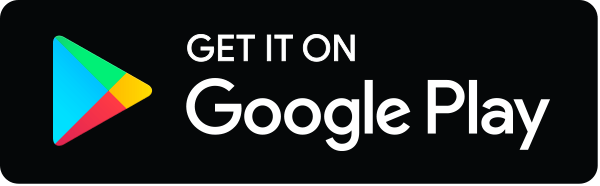