Chapter 27 Fluid Therapy with Macromolecular Plasma Volume Expanders
In the late nineteenth century, Ernest Starling proposed the concept that the balance between hydrostatic and osmotic pressure gradients between the intravascular and interstitial fluid compartments governed transvascular fluid exchange.151 He postulated that a hydrostatic pressure gradient in excess of the osmotic gradient at the arterial end of the capillary bed results in a net transudation of fluid into the interstitium. At the venous end of the capillary bed, plasma proteins (which do not normally pass out of the blood vessels) exert an osmotic force in excess of the hydrostatic gradient, resulting in a net fluid flux into vessels. More than a century of research has confirmed that Starling’s hypothesis provides the foundation for microvascular fluid exchange but also has revealed that the anatomy and physiology of the microvasculature, interstitium, and lymphatic system are much more complex. Consequently, a much deeper understanding of transvascular fluid dynamics is necessary for a logical and rational approach to intravenous therapy with fluids containing macromolecules. This chapter assumes the reader is familiar with the information given in Chapter 1 explaining the fluid compartments of the body and the mechanisms of water and solute flow among compartments. Although this chapter discusses the anatomy, physiology, and biophysics of transvascular fluid dynamics in some depth, comprehensive reviews and texts are available on the subject for a more complete discussion of solute and solvent exchange among the microvasculature, interstitium, and lymphatics.5,127,154 The main aim of this chapter is to objectively address the complexities and controversies of colloid therapy while avoiding the tendency toward bias apparent in some articles dealing with the crystalloid-colloid controversy. A deeper appreciation of the relevant issues should ensure a more rational approach when deciding whether colloid therapy is appropriate. The present chapter is exhaustive in its dealing with some issues but not all-inclusive, and the reader also is referred to several reviews of colloid fluid therapy available in the veterinary31,54,98,135 and human medical literature.55,56,103,129,177
The microvascular barrier
In simple terms, the healthy microvascular barrier is a capillary wall that is relatively impermeable to protein. In addition to the endothelial cell and the capillary basement membrane, a luminal surface layer (the glycocalyx) and the interstitial matrix also contribute to the selective permeability of the microvascular barrier.5,127,180 The glycocalyx coats the luminal aspect of the endothelial cell and is composed of proteins, glycoproteins, and glycolipids that modify the permeability of the microvessel by occupying spaces within the wall or via electrostatic attraction or repulsion.93 Plasma proteins, especially albumin and orosomucoid, are thought to contribute significantly to maintaining the selective permeability of the endothelium.45–47,74,102
On a morphologic basis, capillary walls may be continuous, fenestrated, or discontinuous.122,158 Continuous capillaries, which are found in the majority of tissues and organs of the body, are so called because the wall is composed of a continuous endothelial cell and basement membrane. They are freely permeable to water and small solutes such as sodium but are relatively impermeable to macromolecules. The passage of smaller plasma proteins, such as albumin (molecular radius of 3.5 nm), is less restricted than the passage of larger plasma proteins. Fenestrated capillaries have a continuous basement membrane with regions that are only covered by thin endothelial diaphragms or are entirely devoid of endothelium. They are found in tissues characterized by large fluxes of water and small solutes such as the glomerulus and the intestine. Interestingly, the permeability of fenestrated capillaries to macromolecules is similar to that of continuous capillaries. This feature has been shown to be a result of a net negative charge of the basement membrane.12,146 Discontinuous capillaries are found in the liver, spleen, bone marrow, and some glands. They have gaps up to 1 μm between endothelial cells with no basement membrane and are therefore freely permeable to protein.
The permeability of the microvascular barrier has been explained by the presence of pores of differing sizes.111 These pore sizes often are extrapolated from experimental data regarding fluid and solute fluxes and do not always correlate with morphologic studies such as electron microscopy, implying that they represent functional rather than anatomic entities. The majority of experimental data suggest there are two effective pore sizes in the microvascular barrier in most tissues, with a high frequency of small pores that restrict efflux of macromolecules and a low frequency of large ones through which macromolecules can pass freely.127
Rather than being a free fluid space, the interstitium represents a dynamic environment that may contribute to the permeability characteristics of the microvascular barrier and modify the flow of fluid and macromolecules from the blood vessels to the lymphatics.5,10,11 The interstitium is composed of a collagen framework that contains a gel phase of glycosaminoglycans (of which hyaluronan is the most common), along with protein macromolecules and electrolytes in solution. The relative proportions of these constituents differ widely among organs and tissues, resulting in variations in the permeability and mechanical properties of the interstitium. Glycosaminoglycans are extremely long chains of repeating disaccharide subunits wound into random coils and entangled with each other and the collagen framework. They have molecular weights of the order of 107, and each molecule bears many thousand anionic moieties.5 This interstitial structure has been suggested to mechanically oppose distention (i.e., edema formation) and resists contraction during dehydration because of repulsion between the anionic moieties.71 The interstitial matrix itself is differentially permeable to macromolecules, and a colloid osmotic gradient also can exist from the perimicrovascular space across the interstitium to the lymphatics. Although the collagen network and many of the glycosaminoglycans are fixed in the interstitium, hyaluronan may be mobilized and removed via lymphatic drainage, thereby altering the permeability of the interstitium.5 Increased microvascular permeability may occur during inflammatory states thereby exacerbating macromolecule extravasation.
Transvascular fluid dynamics
or
For a solute to exert its full osmotic pressure across a membrane, the membrane must be impermeable to the solute. If the membrane is partially permeable to the solute molecule, the equilibrium concentration gradient is lower, and the solute exerts only part of its potential osmotic pressure. The realization that the microvasculature was only partially impermeable to smaller macromolecules led to the inclusion of the reflection coefficient (σ) in the fluid flux equation.155
Each different constituent of plasma may differ in its rate of efflux from a vessel depending on such factors as its molecular radius, shape, and charge, and the permeability of the microvascular barrier to the constituent in question. The two major groups of molecules with respect to transvascular fluid flux are termed the solvent phase and the solute phase, and expressions were developed to predict the egress of both major groups of molecules from the microvasculature.83,92,110,115 The solvent phase includes water and those molecules that are not significantly impeded in their passage through the microvascular barrier, whereas the solute flux equation describes the passage of molecules that do not flow freely from the vasculature.
The two major mechanisms of solute flow through the microvascular barrier are convection (i.e., carriage in a bulk flow of fluid) and diffusion (i.e., random motion resulting in net movement of molecules from an area of high concentration to an area of lower concentration).127 An analogy to illustrate the two mechanisms would be a wave breaking on a beach. Some of the sodium molecules in the wave will be moving away from the beach by diffusion; however, the forward convective flow of the wave carries them in the opposite direction.
At normal lymph flow rates, convection has been estimated to account for approximately 30% of the total flux of albumin into lymph.123 An important point that warrants further emphasis is that the rate of solute efflux is dependent on the rate of solvent efflux. Any condition that increases the rate of fluid flow across a membrane can increase the extravasation of macromolecules. Hence, intravenous fluid therapy with crystalloid or colloid can increase albumin loss into the interstitium.124
These mathematical expressions give the impression of a constant hydrostatic pressure gradient acting across a single membrane of static and uniform conductivity and permeability (homoporous), with filtration opposed by an osmotic pressure resulting from a single impermeant solute, the plasma “protein.” In fact, the hydrostatic pressure and osmotic pressure gradients vary among different tissues and at different levels of the capillary bed within the same tissue.121,156,159 In disease states, the differences among organs may be significant and the clinician must consider the possibility of individual organ edema (e.g., pulmonary, myocardial, or intestinal edema) even if there are no overt signs of a systemic edematous state. The total osmotic gradient is a summation of all the impermeant solutes present within plasma, which all have unique reflection coefficients and efflux rates.156 Furthermore, the surface area of the capillary bed may change depending on precapillary sphincter activity and the permeability of the microvascular barrier and interstitium may also vary physiologically and in disease states.8,71,113,180,181
Normal starling forces and the tissue safety factors
Plasma colloid osmotic pressure
Although in popular usage colloid is interpreted most often as referring to a macromolecule that cannot pass through a membrane, the strict definition refers to the dispersion in a gas, liquid, or solid medium of atoms or molecules that resist sedimentation, diffusion, and filtration. This definition is in contradistinction to crystalloids, which are freely diffusible. Oncotic pressure is defined as the osmotic pressure exerted by colloids in solution (hence it is redundant to use the phrase colloid oncotic pressure). Proteins in plasma are truly in solution, but they closely resemble a colloid solution and thus are referred to and treated as such. The osmotic pressure exerted by the naturally occurring colloids in plasma is higher than that calculated for an ideal solution in vitro. One of the main reasons for this discrepancy is that negatively charged proteins (such as albumin, which has a net negative charge of 17 at physiologic pH) retain cations within the intravascular space by electrostatic attraction (termed the Donnan effect).71 These cations contribute to the effective plasma protein osmotic pressure because osmotic pressure is proportional to the number of molecules present rather than their size. Therefore colloid osmotic pressure (COP) is the most correct term when referring to the osmotic pressure exerted by plasma proteins and their associated electrolyte molecules. For comparison, the oncotic pressure exerted by an albumin solution of 7 g/dL is 19.8 mm Hg, whereas the in vivo COP is actually 28 mm Hg, and the total osmotic pressure of all plasma solutes is 5400 mm Hg.71
By virtue of its relatively high concentration in the vascular space, albumin usually accounts for 60% to 70% of the plasma COP with globulins making up the remainder.108,168,176 Interestingly, the variation in COP in dogs may be because of differences in globulin concentration than in albumin concentration.65,108 Red blood cells and platelets do not contribute significantly to plasma COP.118 Serum albumin concentration is determined by the relative rates of synthesis, degradation, and loss from the body and its distribution between the extravascular and interstitial spaces. Albumin synthesis, which is unique to the liver, appears to be regulated, at least in part, by the hepatic plasma COP.53,117,130 Increases of plasma COP independent of albumin concentration, such as in hyperglobulinemia, are associated with decreased serum albumin concentration.18,131,132 The main site of albumin degradation is uncertain, but the reticuloendothelial system has been suggested. Equations have been derived to estimate plasma COP from plasma protein concentrations,108,160 but direct measurement with a colloid osmometer is more accurate.7,28,160,176
COPs measured in normal dogs and cats are given in Table 27-1.44,108,186
Table 27-1 Colloid Osmotic Pressure in Normal Cats and Dogs
Species | Colloid Osmotic Pressure Mean ± SD (mm Hg) | Reference Number |
---|---|---|
Canine (plasma) | 20.8 ± 1.8 | 185 |
Canine (plasma) | 17.5 ± 3.0 | 108 |
Canine (whole blood) | 19.9 ± 2.1 | 44 |
Feline (plasma) | 19.8 ± 2.4 | 185 |
Feline (whole blood) | 24.7 ± 3.7 | 44 |
Interstitial colloid osmotic pressure
Capillaries are permeable to protein, despite the fact that the microvascular barrier greatly restricts macromolecular flux. Of the total quantity of albumin present in the body, 40% is intravascular and 60% is extravascular.133 Furthermore, all of the albumin present in plasma circulates through the interstitium every 24 hours.114 The interstitial COP varies from tissue to tissue depending on such factors as the permeability of the capillary wall to protein, the rate of transvascular solvent flow, the retention of protein in the interstitial matrix, and the rate of lymphatic clearance of protein. The microvascular barrier of skeletal muscle or subcutaneous tissue is relatively impermeable to protein, whereas the pulmonary capillary endothelium is more permeable with a reflection coefficient to albumin of approximately 0.5 to 0.64.113 Consequently, the normal protein concentration in lymph from skin or skeletal muscle is approximately 50% that of plasma compared with 65% in pulmonary lymph.113 Hyaluronan and its associated cations also may contribute to interstitial COP.5 Because of the volume occupied by the interstitial matrix, interstitial albumin is distributed in a volume that is less than the total interstitial volume. This phenomenon is called the volume exclusion effect, and the “excluded volume” with respect to albumin may be as high as one half to two thirds of the total interstitial volume.13,112,175 Consequently, in a normally hydrated interstitium, much less protein is required to exert a given osmotic pressure, and relatively smaller volumes of extravasated fluid result in greater decrements in interstitial COP. This effect maintains the intravascular-to-extravascular COP gradient in early edema formation. Conversely, when interstitial volume is overexpanded by fluid in edematous states, a dramatic increase occurs in the volume available for albumin sequestration.71 The increase in interstitial COP that occurs with dehydration acts to restrict mobilization of interstitial fluid.76
Intravascular hydrostatic pressure
Intravascular hydrostatic pressure is the main force that determines fluid egress from the vasculature. It may vary in different tissues and at different levels within each capillary bed. The normal hydrostatic pressure in the capillary bed is controlled by local myogenic, neurogenic, and humoral modulation of the arterial and venous resistances. Precapillary arteriolar constriction may reduce flow, and therefore hydrostatic pressure, through a capillary bed or shunt flow away from that bed, resulting in changes in the total surface area available for transvascular fluid movement. The hydrostatic pressure within a blood vessel at any particular site depends in part on where resistance to flow occurs, with hydrostatic pressures decreasing most across the areas of major resistance. In most tissues, the majority of resistance has been attributed to small arterioles, but experimental studies of the lung suggest that a significant pressure decrease may occur across the capillary bed itself.15,16,143
Interstitial hydrostatic pressure
As with all the other Starling forces, normal interstitial pressure also varies among tissues. Interestingly, in many tissues the resting pressure is slightly negative (subatmospheric), tending to favor rather than oppose fluid filtration from the microvasculature.179 This finding has been postulated to be the result of the molecular structure of the interstitial matrix, such that with normal hydration the biomechanical stresses on the molecules and the repulsion among like electrostatic charges act to expand the interstitium.5 In encapsulated organs, such as the kidney, normal interstitial pressures are positive. Interstitial pressures can also change depending on the functional state of the organ. For example, interstitial pressures in the nonabsorbing intestine are negative to slightly positive, whereas intestinal interstitial pressures are positive in the absorptive state.70 As mentioned before, the molecular structure of the interstitium mechanically opposes distention. Conventionally, it is said that one third of the total body water is found in the extracellular space and that the interstitium constitutes three fourths of the extracellular space. These figures are averages for the whole body, and the relative sizes of the intravascular and interstitial spaces vary among tissues. Tissues vary in their capacity to accommodate interstitial fluid depending on the size of the interstitial space relative to the total volume of the tissue and the nature of the interstitial matrix itself, especially its distensibility. The distensibility of an organ or tissue is termed its compliance, and depending on the nature of the tissue, the compliance of the interstitium may vary widely. Extreme examples would be tendon (which is relatively noncompliant) and loose subcutaneous connective tissue (which is relatively distensible). The accumulation of edema fluid in the peribronchovascular interstitium in canine lungs is likely the result of the higher compliance of this region of the pulmonary interstitium.
An extremely important concept related to the interstitial hydrostatic pressure is that of stress relaxation. In a normally hydrated animal, the interstitium in most tissues is relatively noncompliant. Small increases in volume caused by increased fluid extravasation result in large changes in interstitial hydrostatic pressure that act to oppose further extravasation of fluid and increase lymphatic drainage pressure—two of the tissue safety factors described later.72,157 As the interstitium becomes gradually more distended, it continues to oppose distention until a critical point is reached (suggested to correspond to the disordering of the interstitial matrix). Abruptly, the resistance to distention decreases (i.e., compliance increases), and fluid then can accumulate without a corresponding protective increase in interstitial pressure and lymph flow. At this point, the distended interstitium no longer opposes the movement of fluid and protein, resulting in increased extravasation and self-perpetuation of the edemagenic process. Furthermore, the greatly increased interstitial space provides a large volume for protein sequestration.
Tissue safety factors
From the previous discussion, it should be apparent that there are three main homeostatic mechanisms that prevent or limit accumulation of fluid in the interstitium. First, extravasation of fluid into a relatively nondistensible interstitium results in an increased interstitial hydrostatic pressure that opposes further extravasation. Second, after extravasation of low-protein fluid, interstitial COP decreases because of dilution and washout of protein, thereby maintaining or even enhancing the COP gradient between the intravascular space and interstitium. Third, the increased interstitial pressure results in an increased driving pressure for lymphatic drainage. These alterations in Starling forces that act to limit interstitial fluid accumulation have been termed the tissue safety factors.72,157 Their relative importance varies depending on the characteristics of the tissue.5,33 In a tissue that is relatively nondistensible (e.g., tendon), an increase in interstitial pressure may be the most important means by which to counteract filtration. In a tissue with moderate distensibility and with a relatively impermeable microvascular barrier (e.g., skin), the decrease in interstitial COP assumes more importance in protecting against interstitial fluid accumulation. In a distensible tissue that is quite permeable to protein (e.g., lungs), increased lymph flow appears to be the most important safeguard against interstitial edema.183
Pharmacokinetics and pharmacodynamics of macromolecular plasma volume expanders
Despite this great heterogeneity, the concept that net fluid extravasation depends on the balance between intravascular COP and capillary hydrostatic pressure forms the basis for intravenous colloid therapy.64,73,90,174 By virtue of their larger molecular size, and in the absence of an increase in microvascular permeability, colloid molecules are retained within the vasculature to a greater degree than are crystalloids. Consequently, smaller volumes of colloid result in greater plasma volume expansion compared with crystalloid,51,144,145 and crystalloid is expected to leak into the interstitium to a greater degree than colloid and cause more interstitial expansion or edema.27 This may be beneficial if the animal has an interstitial fluid deficit or deleterious if there is interstitial edema. One hour after infusion of a crystalloid solution, as little as 10% of the infused volume may remain in the intravascular space.145 Some evidence indicates that tissue perfusion is better after volume expansion with colloids than with crystalloids, even when resuscitation is titrated to physiologic endpoints.63 Unfortunately larger colloids may reduce tissue perfusion by increasing plasma viscosity.22 Many factors influence the volume and duration of intravascular expansion associated with artificial colloids, including the species of animal, dose, specific colloid formulation, preinfusion intravascular volume status, and the microvascular permeability. These factors likely explain the great variability in intravascular persistence and volume expansion in published studies.
Artificial colloids are polydisperse; that is, they contain molecules of different molecular weight. In contrast, in a monodisperse colloid such as albumin, molecules are all the same size. The artificial colloids have extremely complex pharmacokinetics in part because of this large range of molecular sizes.84 The smaller molecules pass rapidly into the urine and interstitium, whereas the larger molecules remain in circulation and gradually are hydrolyzed by amylase or removed by the monocyte phagocytic system.161 This initial rapid excretion of small, osmotically active molecules followed by gradual elimination of large molecules results in an exponential decline in intravascular expansion. Manufacturer data sheets can be misleading because they may imply that a major proportion of the volume expansion lasts for 24 to 36 hours. Estimates of the degree of initial plasma volume expansion for hetastarch and dextran 70 vary from 70% to 170% of the infused volume.67,77,87,91,124 This decreases to approximately 50% of the infused volume after 6 hours. Volume expansion with hydroxyethyl starch then declines gradually from 60% to 40% of the infused volume during the next 12 to 18 hours, whereas with dextran 70 it decreases gradually from 40% to 20% of the infused volume.161 In experimental dogs, blood volume was increased by approximately 25% both immediately and 4 hours following infusion of 20mL/kg of both dextran 70 and hetastarch.147 In dogs with hypoalbuminemia of various causes receiving hydroxyethyl starch, COP was not significantly different from baseline 12 hours after infusion.106 In the authors’ experience, the duration of volume expansion with artificial colloids can be even shorter, especially with capillary leak syndromes. This relatively short duration of action and the high cost of artificial colloids have led some authors to question the cost-effectiveness of colloid infusions in veterinary patients.173
The duration of action of colloids may be expressed in terms of plasma colloid concentrations, plasma COP measurements, or degree of volume expansion. The initial volume of intravascular expansion is the result of the COP of the infused colloid, which is determined by the number of molecules, not their size. This concept is extremely important because the distribution of molecular weights is narrowed after intravenous infusion.57,58 The smaller molecules that are responsible for a large part of the COP and intravascular volume expansion are extravasated or excreted within hours. The intravascular colloid concentration (i.e., mass per unit volume) is still high due to the large molecules, but the COP is relatively low. COP and degree of volume expansion tend to decrease faster than does the plasma concentration of colloid. Data from an experimental study of euvolemic human volunteers given twice the usual dose of a high molecular weight form of hydroxyethyl starch may therefore have little bearing on the effects of commercially available hydroxyethylstarch in a dog with systemic inflammatory response syndrome in hypodynamic, septic shock.
To reiterate, the osmotic effect of macromolecules is because of their number rather than their size. Consequently, if more than 50% of the molecules leak into the interstitium, a net reduction in intravascular volume is likely as water leaves the intravascular space by osmosis along with the colloid. Therefore the difficulty is how to determine the magnitude of increase in permeability (i.e., the size of the “gaps” in the microvascular barrier). Although experimental techniques exist to detect an increase in microvascular permeability,14,25 they are not currently applicable in a clinical setting. A growing body of evidence suggests that hydroxyethyl starches can mitigate increases of microvascular permeability in several capillary leak states.34,97,109,184 The optimal molecular weight for this effect seems to be between 100 and 300 kDa.185 Unfortunately, relatively few products with molecules in this size range are available in the United States. Only 35% of the molecules in one preparation of hetastarch fall within this optimal size range.184 European formulations of hydroxyethyl starch (e.g., Haes-steril, Fresenius Kabi, Bad Homburg, Germany) contain more molecules in the optimal molecular size range.
Colloid preparations
The terms “hetastarch,” “pentastarch,” and “tetrastarch” are nonspecific terms used to describe different preparations of hydroxyethyl starches. The term “hetastarch” and the abbreviation “HES” are sometimes used interchangeably, but this should be avoided; hetastarch is just one of the hydroxyethyl starches. The abbreviation, HES, may be correctly used as an umbrella term for all hydroxyethyl starches, which are then subclassified on the basis of their molecular structure. The HES family is most precisely described by reference to their molecular weight and their degree of substitution (e.g., HES 450/0.7 or HES 130/0.4). These characteristics are described more fully later. The C2/C6 hydroxyethylation ratio is another important pharmacologic characteristic that may be used as a descriptor but it is not routinely included in product descriptions at this time.177
Molecular Weight (MW)
In general, the molecules in HES preparations show great polydispersity. The molecules can range in size from a few thousand to a few million Daltons and in any one solution will generally follow a bell-shaped distribution. Hydroxyethyl starches have been arbitrarily divided into high molecular weight (>400 kDa), medium MW (200 to 400 kDa) and low MW (<200 kDa) preparations. The quoted MW represents the weight average molecular weight (e.g., 480 kDa for Hespan, 130 kDa for Voluven) but the actual range of sizes is wide. For example, the package insert for Hextend (Hospira, Lake Forest, Ill.) states that 80% of molecules fall between 2 and 2500 kDa, which means that 20% fall outside of this range. An independent analysis found that 85% of Hespan (Teva, Irvine, Calif.) consisted of molecules smaller than 300 kDa, 50% consisted of molecules smaller than 100 kDa, and molecular masses ranged up to 5000 kDa.184 It should also be remembered that the quoted MW only applies to the solution in vitro; as soon as the product is administered to a patient, the average MW will change as the product is subjected to excretion and hydrolysis. Measurement and interpretation of MW is further complicated as the quoted weight average molecular weight is only one way of calculating the “average” weight of a polydisperse polymer, the other method being the number average molecular weight. The number average molecular weight simply represents the total weight of polymer in solution divided by the number of molecules, whereas the weight average takes into account that the polymers are of different sizes and is exaggerated by larger particles in the mixture. As the detrimental effects of the colloids have been considered to be related to the presence of the higher molecular weight polymers, the weight average may be the more appropriate measure. The weight average MW is calculated from light scattering of the solution. The polydispersity index can be calculated from the ratio of the weight average to the number average for that solution. Hydroxyethyl starch with a weight average molecular mass of 100 to 300 kDa seems to provide the best compromise between colloid osmotic volume expansion and duration of action.40 Furthermore, this size distribution has less effect on coagulation164 and is the best size for reducing the increases in permeability present in patients with vascular leaks.184
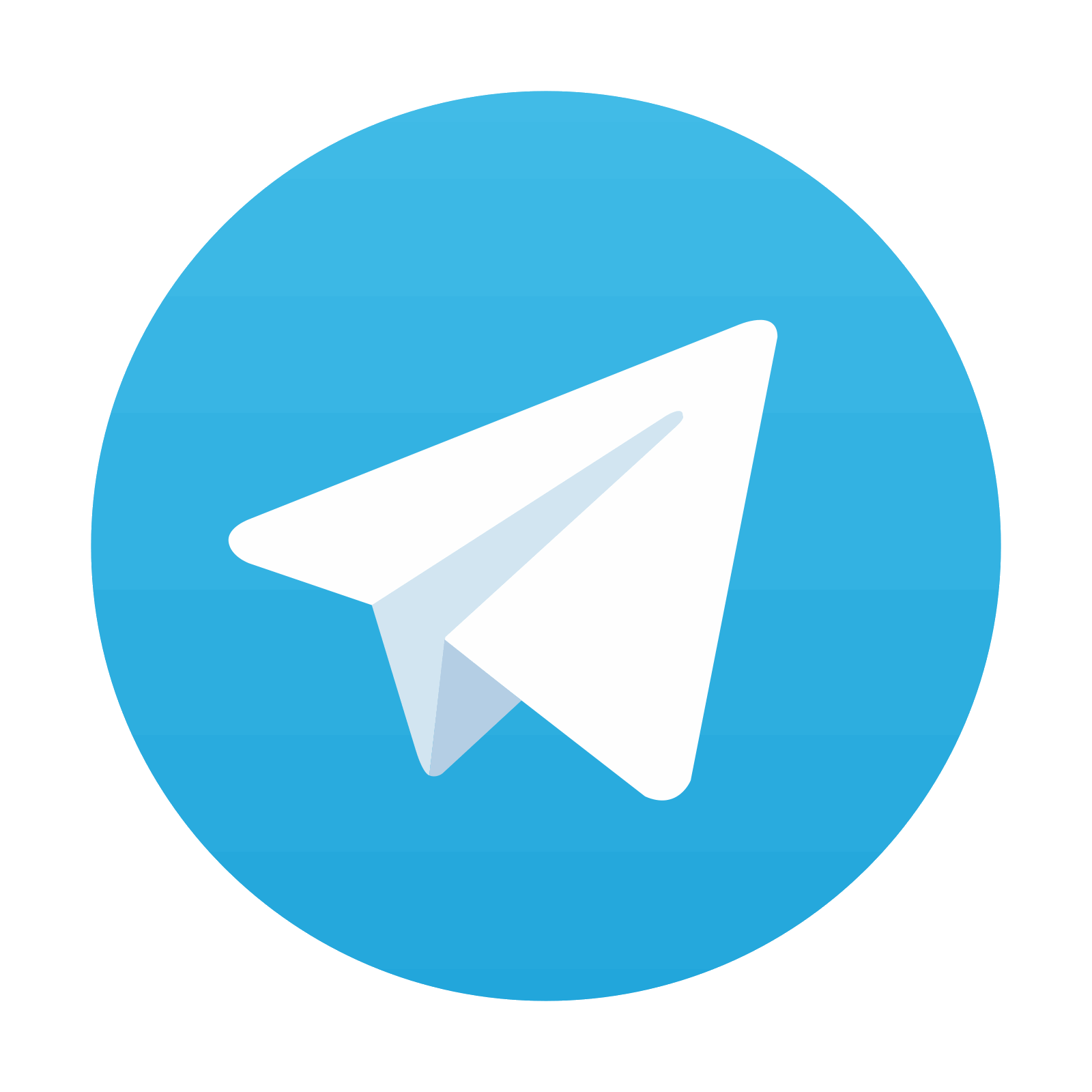
Stay updated, free articles. Join our Telegram channel
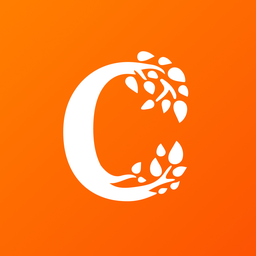
Full access? Get Clinical Tree
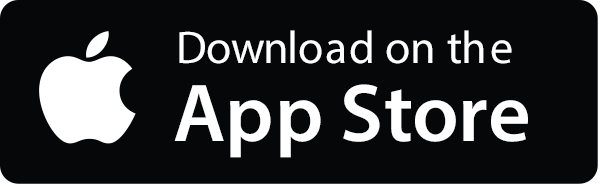
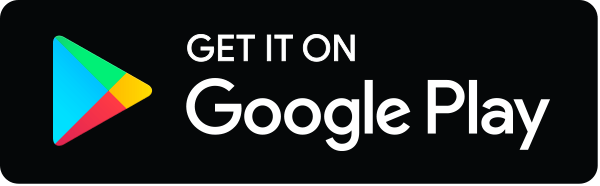