Chapter 5 In the nineteenth century the pioneers of fluid therapy for the management of surgical patients were Albert Landerer from Germany and Rudolph Matas from the United States.72,79 Only severely ill patients received intravenous fluids and proctoclysis (rectal administration of fluid), while less critical patients were given subcutaneous and intraperitoneal fluid therapy.6 Over the next century, colloids, blood products, and hypertonic solutions were discovered and researched. Although research and debate regarding the “best” fluid for patients in need are ongoing, advancements in both veterinary and human intravenous fluid therapy are remarkable. Water comprises ≈60% of body weight in the nonobese adult dog or cat. Total body water is distributed between two major compartments: intracellular and extracellular fluid (Figure 5-1).115,50 Each compartment consists of solutes, primarily electrolytes, dissolved in water, and all compartments have the same osmolarity (≈290 to 310 mOsm/L) in health. The size of each compartment is determined primarily by the quantity of solutes it contains. A decrease in total body water occurs as an animal ages, following acute exercise, in obese animals, and following chronic exposure to high altitudes. Very young (<3 months), emaciated, or pregnant animals have an increase in total body water.53 The intracellular fluid compartment is the larger of the two compartments and accounts for 66% of the total body water and 40% of body weight. The cell membrane separates the intracellular fluid from the extracellular fluid and is very permeable to water, but not solutes. The cell membrane contains different types of proteins, some of which are channels that allow certain solutes to pass through, while others actively transport solutes across the membrane. The most important transporting protein is the Na+-K+/ATPase; it extrudes Na+ from the cell and transports K+ into the cell, consuming adenosine triphosphate (ATP) as it moves the solutes against their concentration gradients. This movement of Na+ and K+ contributes to the large disparity in Na+ and K+ concentrations between intracellular fluid and extracellular fluid: Na+ concentration is very high in the extracellular fluid and very low in the intracellular fluid, and K+ is very high in the intracellular fluid and very low in the extracellular fluid. The major cation in the intracellular fluid is K+, with much smaller contributions made by Mg++ and Na+. Major anions in the intracellular fluid include PO4−2 and the polyanionic charges of the intracellular proteins.50,53 The remaining 33% of total body water (TBW) and 20% of body weight make up the extracellular fluid. It is subdivided into plasma (plasma is considered to be synonymous with intravascular and is 25% of extracellular fluid) and interstitial (75% of extracellular fluid) fluid compartments. Interstitial fluid bathes all cells and includes lymph. Movement of fluid from the intravascular to extravascular (interstitial and intracellular) space occurs at the level of the capillary. The capillary membrane is composed of endothelial cells and a subendothelial matrix that separates the intravascular space from the interstitial space. The endothelium of the capillary is freely permeable to water and small-molecular-weight particles (e.g., ions, glucose, acetate, lactate, bicarbonate), and gases such as oxygen and carbon dioxide readily diffuse across the capillary wall according to the pressure gradient. The interstitial space between the vessels and the cells is made up of protein-rich fluid and tissue matrix. The primary cation in the extracellular fluid is Na+, and major anions are Cl− and HCO3−. However, proteins in plasma and the interstitial space also contribute to the negative charges. The ratio of proteins between the intravascular and interstitial space determines the oncotic pressure gradient between compartments.53 The rate of fluid administration in the preoperative surgical patient with volume depletion depends primarily on the clinical status of the animal, based on the physical examination and laboratory parameters. For animals with evidence of chronic dehydration on physical examination but stable cardiovascular parameters, fluid deficits are typically replaced over 6 to 24 hours. However, preoperative patients requiring more emergent surgery that have evidence of dehydration will benefit from a more rapid fluid replacement plan. Isotonic replacement fluids should be administered according to the patient’s estimated dehydration, maintenance needs, and anticipated ongoing losses. Physical examination findings in animals with evidence of dehydration can be found in Table 5-1. General guidelines for rehydrating patients with evidence of interstitial dehydration (loss of water and solutes from the interstitial space most commonly seen in animals with 5% to 8% dehydration) use the following formula: Table • 5-1 Physical Examination Findings in Dehydrated Patients Ongoing losses include those caused by vomiting, diarrhea, polyuria, open wounds or burns, fever, third-spacing, or blood loss. Although these losses are only estimates and may be challenging to quantify, weighing diapers, bandages, or blue pads may prove helpful. Maintenance fluid rates are estimated at 2 to 4 mL/kg/hr, with larger or overweight animals using the lower end of the range and smaller or thin patients the upper end. More exact requirements can be found in Table 5-2. For those patients with evidence of cardiovascular compromise or “shock,” a more rapid fluid administration protocol is indicated (see Chapter 6 for further details). Treatment of an animal in shock requires restoration of organ perfusion to provide adequate oxygen delivery to the tissues. Oxygen delivery to the tissues (DO2) is determined by several factors (Figure 5-2). Animals requiring surgery often need fluid therapy before receiving general anesthesia. It is important to ensure that preoperative patients are well hydrated and cardiovascularly stable, and have adequate oxygen content. Severe electrolyte or acid-base abnormalities should be corrected before surgery is begun. Correction of clinical anemia, volume deficits, or electrolyte and acid-base derangements is especially important in the presurgical patient population because anesthetic drugs commonly have negative effects on the heart, blood pressure, and baroreceptor response. In addition, blood loss during surgery can exacerbate the cardiovascular effects of the drugs, and these animals cannot compensate normally to acute hypovolemia. Tissue ischemia can interfere with wound healing and normal tissue defenses and should be avoided. The only exception to this recommendation may be seen in animals with uncontrollable bleeding. It may even be advantageous to perform “hypotensive resuscitation” (to a mean arterial pressure of ≈60 mm Hg or a systolic blood pressure of ≈90 mm Hg) in these animals until the hemorrhage is surgically controlled, because aggressive fluid therapy in this setting can worsen bleeding and outcome.39 During anesthesia, most animals are given 5 to 10 mL/kg/hr of isotonic crystalloids (without added electrolytes) intravenously to maintain intravascular volume and pressures. Although this rate is based on human research in the 1960s,100,113 it was not found to be harmful in two feline veterinary studies.47,12 Additional fluid requirements are typically determined by the length and complexity of the procedure that is done. Close monitoring of the animal’s vital signs, blood pressure, and pulse oximetry readings (to ensure adequate oxygen saturation of the blood) will help to ensure adequate tissue perfusion and oxygen delivery. Some animals will also benefit from central venous pressure monitoring during surgery. Infusion of isotonic crystalloid fluids does not significantly change the osmolarity of the vascular or extravascular (both interstitial and intracellular) space. These fluids are typically used to expand the intravascular and interstitial spaces and to maintain hydration. Isotonic crystalloids most commonly used contain mixtures of electrolytes, water, ±acid-base components, ±dextrose. The constituents of frequently used isotonic fluids can be found in Table 5-3. Most available isotonic crystalloids, other than 0.9% NaCl, contain a bicarbonate precursor such as lactate, acetate, or gluconate. The lactate is metabolized by gluconeogenesis or oxidation. This metabolism occurs primarily in the liver, although peripheral metabolism in the kidney and muscle tissues also occurs. Hydrogen is consumed during metabolism of lactate, and an alkalinizing effect in the blood ensues around 30 minutes following administration. Lactated Ringer’s solution contains either just L-lactate or a racemic mixture of D– and L-lactate. Because D-lactate is not readily metabolized in dogs, the alkalinizing effect is not as profound as that seen with acetate.56 Acetate is metabolized most often in muscle tissue, whereas gluconate can be metabolized by most cells in the body. Isotonic saline is unbalanced in that it contains higher concentrations of sodium (154 mEq/L) and chloride (154 mEq/L) compared with normal plasma, and will cause proportionate changes (increases) in a normal animal’s electrolytes. Therefore, large amounts of 0.9% NaCl will cause a mild increase in sodium, a marked increase in chloride, and a moderate decrease in bicarbonate and potassium. The kidneys will typically compensate, if possible, by excreting the excess electrolytes and conserving potassium. Animals with hypochloremia, hyponatremia, or a metabolic alkalosis will often benefit from the administration of 0.9% NaCl. Excessive fluid administration should be avoided and can be harmful to the small animal surgical patient (see Table 5-2). Interstitial fluid gain can lead to interstitial edema, pulmonary edema, and cerebral edema. Surgical patients that have low colloid osmotic pressure, pulmonary contusions, cerebral trauma, fluid nonresponsive renal disease, or cardiac disease are at highest risk for complications. In addition, substantial hemodilution of red blood cells, plasma proteins, clotting factors, and platelets can occur. Therefore, anemia, hypoproteinemia, and hypocoagulability may result after administration of large volumes of crystalloids. Animals with recently lacerated or ruptured blood vessels are susceptible to rebleeding following aggressive fluid therapy. In those animals suffering blood loss, hypotension may contribute to cessation of bleeding. During fluid resuscitation, the increase in intravascular volume can lead to a rapid rise in intravascular hydrostatic pressure that “pops the clot.” Before surgical correction of the bleeding, hypotensive fluid resuscitation (to a mean arterial pressure of 60 mm Hg, or a systolic pressure of ≈90 mm Hg) may help prevent rebleeding while maintaining perfusion to vital organs. 1. Surgical patients with head trauma should be resuscitated with 0.9% NaCl, if possible, because this fluid has the highest sodium concentration and therefore is least likely to cause a decrease in osmolarity and subsequent water movement into the brain interstitium. Postoperative monitoring and maintenance of normal sodium levels are also important to prevent marked changes in osmolarity. 2. Perioperative animals with severe hyponatremia or hypernatremia should receive crystalloid fluids that most closely match the patient’s sodium concentration during resuscitation to avoid a rapid increase or decrease in serum osmolarity and subsequent central pontine myelinolysis (often delayed in onset) or cerebral edema, respectively. Gradual normalization of sodium concentration (increase of 0.5 mEq/L/hr or decrease of 1 mEq/L/hr) should be performed once the animal is cardiovascularly stable. 3. Surgical patients with a hypochloremic metabolic alkalosis will benefit from 0.9% NaCl because this is the highest chloride-containing fluid. It will help to normalize blood pH by dilution and by increased chloride, with a subsequent decrease in bicarbonate concentration. 4. Surgical animals that are severely acidotic may benefit from a crystalloid that contains a buffer agent such as acetate, gluconate, or lactate (i.e., NOT 0.9% NaCl because this fluid tends to be acidifying). Large quantities of acetate can cause vasodilation and a decrease in blood pressure in animals with preexisting hypovolemia.64,104 This occurs secondary to adenosine release from muscle tissue, and adenosine is a potent vasodilator. Maintenance fluids are hypotonic and refer to the volume of fluid and quantity of electrolytes that must be consumed on a daily basis to keep the volume of total body water and electrolyte content within the normal range. They are especially useful in perioperative patients that are not eating or drinking but are otherwise stable and do not have ongoing fluid losses beyond those of a normal animal. These fluids are also beneficial for animals with a free water deficit as evidenced by mild to moderate hypernatremia. Obligate fluid losses in a normal animal are hypotonic and low in sodium, but contain relatively more potassium than does the concentration of the extracellular fluid. These obligatory fluid losses include insensible losses, such as those due to respiratory evaporation and normal fecal losses, as well as sensible losses, which refers to normal urinary losses. Maintenance fluids are hypotonic crystalloids that are low in sodium, chloride, and osmolarity, but may be high in potassium compared with normal plasma concentrations (Table 5-4). Maintenance solutions include 0.45% sodium chloride, 2.5% dextrose with 0.45% saline, 2.5% dextrose with half-strength lactated Ringer’s solution, Normosol M, and Plasmalyte 56. The dextrose, if included, is rapidly metabolized to CO2 and H2O. These fluids are distributed into all body fluid compartments and therefore are contraindicated as bolus therapy in animals with hypovolemia that require rapid extracellular fluid resuscitation. Large volumes of hypotonic maintenance fluid administration can lead to a rapid decrease in osmolarity and subsequent cerebral edema. Hypertonic (7.0% to 7.5%) sodium chloride administration causes a transient osmotic shift of water from the extravascular to the intravascular compartment. Small volumes of ≈4 to 6 mL/kg can be administered over 10 to 20 minutes. Rates exceeding 1 mL/kg/min may result in osmotic stimulation of pulmonary C-fibers, which leads to vagally mediated hypotension, bradycardia, and bronchoconstriction and should be avoided. Although hypertonic saline is given primarily to shift extravascular water into the intravascular space, evidence suggests that it may also help to reduce endothelial swelling, increase cardiac contractility, cause mild peripheral vasodilation, modulate inflammation, and decrease intracranial pressure.74,90–93 Hypertonic saline is especially useful for the treatment of head trauma or cardiovascular shock in animals >30 kg that require large amounts of fluid for resuscitation and in which time is of the essence (e.g., patients with gastric dilatation volvulus). Because of the osmotic diuresis and rapid redistribution of sodium cations that ensue following administration of hypertonic saline, the intravascular volume expansion is transient (<30 minutes), and additional fluid therapy must be used to maintain intravascular volume and prevent dehydration. For example, combinations of hypertonic saline and synthetic colloid solutions have shown beneficial effects and are described below. Although 25% mannitol could also be used as a hypertonic fluid, it is less effective at increasing intravascular volume because the osmolarity is approximately half that of 7.5% saline. The use of 23.4% saline intravenously has been reported, but the safety margin is small and this practice is discouraged. See Table 5-5 for specific characteristics of these fluids. Synthetic colloid solutions contain primarily large molecules (molecular weight >20,000 daltons) that do not readily sieve across the vascular membrane. Colloidal particles generally range from a few thousand to several million daltons and are suspended in an isotonic crystalloid fluid. When administered intravenously, they increase the colloid osmotic pressure of the plasma, making it hyperoncotic to the extravascular fluid, and therefore pull fluid into the intravascular space. The resultant increase in blood volume is greater than that of the infused volume, and the colloid particles help to retain this fluid in the intravascular space in the animal with normal capillary permeability. Synthetic colloid solutions are commonly used for the treatment of shock and in patients with moderate to severe hypoproteinemia and a decrease in colloid osmotic pressure. Postoperative patients often benefit from these fluids because intraoperative and postoperative fluid losses commonly lead to a low colloid osmotic pressure, which may negatively affect wound healing and predispose patients to bacterial translocation from the gastrointestinal tract into the bloodstream.102 Transvascular movement of fluid in animals with low oncotic pressure can lead to interstitial edema, decreased tissue perfusion, and increased distance for the diffusion of oxygen and nutrients. Potential side effects of synthetic colloid use are related primarily to disruption of normal coagulation. These include a decrease in factor VIII and von Willebrand factor concentrations (decrease beyond a dilutional effect), impairment of platelet function, and interference with the stability of fibrin clots, which makes the clot more susceptible to fibrinolysis.22,106,118 The clinical manifestations of these changes are variable and depend on the status of the patient. Patients with preexisting coagulaopathies, von Willebrand disease (vWD), or moderate to severe thrombocytopenia/thrombocytopathia are at highest risk for developing a side effect. Monitoring of the activated partial thromboplastin time (aPTT) may be helpful in assessing the adverse effects and risk level associated with the use of synthetic colloids, although no precise guidelines have been put forth, and it is difficult to predict which animals will develop clinical bleeding after synthetic colloid administration. In general, appropriate use of synthetic colloid solutions is deemed worth the risk, but judicious use of natural colloids, such as plasma, also may prove necessary to prevent bleeding complications, especially perioperative complications. Caution should be exercised to avoid volume overload or excessive hemodilution when large volumes of synthetic colloids are given to a patient. Additional side effects of synthetic colloids in people include renal impairment and allergic reactions, but similar problems in animals have not been documented. Hydroxyethyl starch preparations contain high polymeric glucose compounds that are manufactured by modification of the highly branched starch, amylopectin. Replacement of hydroxyl groups with hydroxyethyl groups at the C2, C3, or C6 carbon position of the constituent glucose molecules prevents rapid degradation by amylase. The ratio of substitution at the C2 versus C6 position (known as the C2:C6 ratio) also prolongs the half-life of the solution. Hydroxyethyl starch solutions are further characterized by their Mw (low Mw 70 kD, medium Mw 130 to 270 kD, and high Mw 450 kD), their concentration (3%, 6%, or 10%), and their degree of substitution (0.4, 0.5, 0.6, or 0.7). The degree of substitution refers to the number of hydroxyethyl groups per molecule of glucose; the higher the number of substitutions, the slower is the breakdown and elimination of the molecule. However, a higher degree of substitution means greater potential effects on coagulation. Table 5-6 displays the various characteristics of each type of available synthetic colloid. To pull fluid into the vascular space and prolong the effects of intravascular volume expansion, a hypertonic saline/synthetic colloid mixture is commonly used for the resuscitation of animals with noncardiogenic shock. A 1 : 2.5 ratio of 23.4% sodium chloride to hydroxyethyl starch (e.g., Hextend) or hypertonic saline Dextran 70 will make a 7.5% saline mixture (i.e., 17 mL of 23.4% saline added to 43 mL of Dextran 70). Several veterinary studies have supported the beneficial use of hypertonic saline Dextran for resuscitation in dogs with traumatic shock, pyometra with septic shock, burns, hemorrhagic shock, endotoxemia, and gastric dilatation volvulus.* The use of hypertonic, hyperoncotic small-volume resuscitation with hypertonic saline and hypertonic saline Dextran has repeatedly been shown to restore hemodynamic stability, restore splanchnic organ perfusion, attenuate neutrophil migration, reduce increases in postresuscitation intracranial pressure, decrease cardiac troponin I and cerebral protein S-100 levels, and lower pulmonary capillary wedge pressures.4,37,55,71,84 The use of hypertonic saline/colloid solution mixtures perioperatively in small animals deserves further study. The most commonly used and studied hemoglobin-based oxygen-carrying (HBOC) fluid used in small animals is Oxyglobin. It is a sterile, ultrapurified, stroma-free, polymerized bovine hemoglobin solution. It is nonantigenic and therefore does not require blood typing or cross-matching before administration. Oxyglobin has an Mw of 200,000 Daltons and a colloid osmotic pressure of 40 mm Hg. The hemoglobin concentration is 13 g/dL, and it is suspended in a modified lactated Ringer’s solution. The solution has an osmolarity of 300 mOsm/L and a pH of 7.8 (see Table 5-6). The plasma half-life ranges from 18 to 43 hours, depending on the dose administered (10 to 30 mL/kg, respectively). It can be stored at room temperature for up to 3 years from the date of manufacture, but an open bag must be used within 24 hours. It is purple and will cause a yellow-orange discoloration to the animal’s skin, urine, serum, sclera, and mucous membranes. After administration of Oxyglobin, the animal’s hemoglobin must be measured to estimate oxygen-carrying capacity because the hematocrit of the animal is unaffected (or diluted) by Oxyglobin. Several laboratory parameters are invalidated by discoloration of the serum. Although Oxyglobin can be lifesaving in specific animals (i.e., severe, acute anemia as seen with massive blood loss or hemolytic disease), some medical concerns about the safety of this product have been put forth (e.g., it can cause marked vasoconstriction through its nitric oxide–scavenging effect), and it is not superior to natural blood products (and may be more expensive). The recommended dose is 10 to 30 mL/kg (dogs), and it is important to remember that this product is a colloid and can easily contribute to intravascular volume overload (especially in cats). Although the product’s safety and efficacy have not been studied in cats, two retrospective reports have described its use in this species.48,117 Biopure, the manufacturer of Oxyglobin, filed for bankruptcy in July of 2009; therefore the future availability of this product is not known. The need for blood products in the surgical patient is dependent on the patient’s disease process. Most patients can tolerate acute hemodilution to a hematocrit of <20% during the perioperative period, although it is recommended that the hematocrit be kept above 24% in critically ill human patients to ensure adequate oxygen delivery.52 Although similar studies have not been performed specifically for dogs and cats, it is reasonable to believe that the threshold is similar. In surgical patients with hypotension despite aggressive fluid therapy, the hematocrit target may be increased to >30% to maximize oxygen-carrying capacity. Excessive increases in hematocrit should be avoided because a hematocrit above the normal range will increase blood viscosity, subsequently leading to sludging of blood and decreased microvascular circulation. If a surgical patient is expected to lose a large amount of blood during an elective surgery, the animal can donate blood in advance to have autologous blood available. Because it is often difficult to collect blood several weeks or months before surgery in small animals, a single donation technique has been described in cats preparing to undergo a craniectomy.46 Alternatively, acute normovolemic hemodilution can be performed in animals with a normal hematocrit and total protein concentration. This is accomplished by collecting blood immediately before surgery and replacing the withdrawn volume with three times the volume of isotonic crystalloid solution or the same amount of a colloid solution (natural colloid products may induce less of a coagulopathy compared with synthetic colloid solutions). This approach is based on the premise that blood that is lost during surgery will contain less protein and red cell volume (because of hemodilution following blood collection and volume replacement) than collected blood, and collected blood will then be available to replace that loss whenever needed during or after surgery.16,114 This technique tends to be expensive and minimally beneficial, however. Current transfusion guidelines in human medicine recommend that preoperative autologous blood donation, intraoperative and postoperative blood recovery, acute normovolemic hemodilution, and avoidance of excessive blood loss be attempted, as appropriate, to decrease the need for autologous transfusions.81 Blood volume is 90 mL/kg in the dog and 50 mL/kg in the cat. Whole blood is used most appropriately for animals that require all of the components present in blood. Nonetheless, it is often used because it is more convenient than individual component therapy. Fresh whole blood contains all of the clotting factors and platelets, but platelets are best administered within 8 hours of collection and are no longer present after 24 hours. Stored whole blood no longer supplies functional clotting factors after 24 hours as well. Stored whole blood is used most commonly in patients that are actively bleeding, have lost large amounts of blood, or are severely hemodiluted from the use of synthetic intravascular volume expanders. Whole blood transfusions may have effects on the immune system and on cancer growth, and therefore should be used only when truly needed, not just for convenience.13,14 Although all blood products should be administered through a filter over at least 1 to 2 hours to monitor for a transfusion reaction and avoid volume overload, it may be necessary to bolus these products in surgical patients with life-threatening hemorrhage and hypovolemia. If blood products are administered quickly, it is important to monitor serum calcium concentration because use of citrate-containing products may lead to clinical hypocalcemia secondary to chelation.1 Animals with vWD that require surgical intervention should be transfused before surgery with cryoprecipitate, fresh frozen plasma, or plasma from donors that received desmopressin (deamine 8-D-arginine vasopressin [DDAVP]) before the time of blood donation. Cryoprecipitate is the most effective therapy, however.20 DDAVP administration (1 mcg/kg subcutaneously) before surgery is performed may prove beneficial in less severely affected animals with vWD.105 Animals with severe thrombocytopenia or thrombocytopathia may require platelet-rich transfusion products preoperatively to minimize blood loss. Because platelets are fragile and have a short half-life, transfusions for these animals are typically obtained on a patient-by-patient basis. Examples of thrombocytopenic or thrombocytopathic patients that may benefit from platelet-rich products include those that must undergo major surgery with the potential for blood loss, animals with platelet counts less than 50 × 109/L, those with clinical evidence of bleeding from thrombocytopenia or thrombocytopathia, and those that suffer from severe trauma or central nervous system injury and concurrent thrombocytopenia. Because transfused platelets are short-lived post transfusion, the goal of platelet transfusion therapy is to stop or prevent potentially life-threatening bleeding, but not to increase platelet numbers per se. Human guidelines are often used as a template for platelet transfusion guidelines.52 Platelet-rich plasma or fresh whole blood is used most commonly in small animal patients requiring platelet transfusions. These preparations should be used within 24 hours of collection. The total amount of whole blood required (TV) is dependent on the platelet count of the patient (PE), the platelet count of the donor animal (PD), the target platelet count (Pt), and the blood volume (BV) of the patient: In animals with recent (<24 hours), large-volume hemorrhage into the pleural or peritoneal cavity, autotransfusion of whole blood may be considered. It is especially useful for animals with intraoperative or trauma-induced hemorrhage to maintain the animal’s PCV and oxygen-carrying capacity until additional red blood cell products can be administered, or in place of allogenic transfusions. Autotransfused blood is not a dependable source of clotting factors because depletion of fibrin and other clotting factors occurs rapidly after hemorrhage into a body cavity. Although the risk of a transfusion reaction and resource depletion and expense associated with allogenic blood transfusions are minimized, it is typically a lifesaving procedure and is not superior to allogenic blood products. Cell salvage is an effective method of autotransfusion that is typically used in people with massive intraoperative blood loss. Shed blood is collected from the operative field and is mixed with an anticoagulant. It is concentrated and washed or filtered, then returned to the patient.63 Harmful contaminants, such as potassium, fat, and free hemoglobin, are removed from the salvaged blood; washed blood is returned via a 40-µm blood filter. Blood obtained from the thoracic cavity via chest tubes in a closed system can be processed and autotransfused in a similar manner. Administration of 25% human albumin has recently gained popularity, although its potential adverse effects have been recognized. Because albumin accounts for 80% of plasma oncotic pressure, animals with severe hypoalbuminemia (i.e., <1 to 1.5 g/dL) that are expected to have continued ongoing losses may benefit from its use. Preliminary studies in dogs show that human albumin administration in dogs will increase circulating albumin concentrations, total solids, and colloid osmotic pressure, although its effect on mortality remains unknown.110 Current and future studies will give us more information about the use of this product. Potential risks include potentially fatal acute or delayed hypersensitivity reactions, volume overload, and coagulopathy.76,44 An increase in immunoglobulin (Ig)G against human albumin does occur in normal and critically ill dogs; therefore repeated exposures are not recommended.76 Canine and feline albumin is now available for purchase, but the research data have not yet been published; thus the use of these products cannot be recommended at this time. Sodium is the primary extracellular cation in the body. The low intracellular concentration of sodium is maintained by the sodium-potassium ATPase on the cellular membrane. Sodium is the primary determinant of extracellular osmolarity. Hypernatremia is always associated with hyperosmolarity; however, hyponatremia usually, but not always, implies hypoosmolarity. Serum sodium concentration is a reflection of serum sodium content relative to extracellular water rather than total body sodium. Patients with high or low serum sodium concentrations can have a high, low, or normal total body concentration of sodium.18,25,26,33,34,49,60,86,101 Normal serum osmolarity and sodium concentration are regulated by free water balance. Vasopressin (antidiuretic hormone) release and thirst are the primary mechanisms responsible for free water balance in the body. Both osmoreceptors and baroreceptors stimulate antidiuretic hormone release and thirst in response to increased plasma osmolarity or hypovolemia, respectively. A hypovolemic state will result in continued antidiuretic hormone release, even in the face of hypoosmolarity, to maintain tissue perfusion. Renal sodium retention or excretion regulates extracellular fluid volume. The juxtaglomerular apparatus of the kidney senses decreased extracellular fluid volume as decreased renal perfusion and responds by releasing renin, which ultimately results in increased serum aldosterone levels (through the renin-angiotensin-aldosterone system). Aldosterone stimulates increased renal reabsorption of sodium and water. In response to a sodium load and increase in effective circulating volume, the kidney senses the increase in renal perfusion and renin release is inhibited, decreasing aldosterone levels and increasing atrial natriuretic peptide, allowing for increased sodium excretion via the kidneys.18,25,26,33,34,49,60,101 Hyponatremia is defined as serum sodium concentration less than 140 mEq/L in dogs, or less than 149 mEq/L in cats. Causes of hyponatremia are listed in Box 5-1. Hyponatremia can occur in conditions of low plasma osmolarity, high plasma osmolarity, or normal plasma osmolarity. Hyperlipidemia or hyperproteinemia can be associated with hyponatremia despite a normal plasma osmolarity because of the method of sodium measurement. This phenomenon is called pseudohyponatremia and is less common now that ion selective electrodes are used instead of flame photometry to measure sodium concentrations. It reflects a problem with measurement rather than a true decrease in serum sodium. Hyponatremia with increased plasma osmolarity is found commonly after mannitol administration or in diabetic patients with severe hyperglycemia. The presence of osmotically active glucose or mannitol in the intravascular space results in movement of water from the extravascular compartment into the intravascular space, diluting the serum sodium concentration.18,26,33,34,86 Hyponatremia in conditions of hypovolemia results from impaired water excretion by the kidneys caused by a decreased glomerular filtration rate, activation of baroreceptors that stimulate antidiuretic hormone release, and activation of the thirst mechanism, which increases water intake. Causes of hypovolemic hyponatremia include gastrointestinal or third space losses (e.g., drainage of chylothorax), hypoadrenocorticism, or diuretic administration.15,18,26,33,34,86 Hyponatremia associated with hypervolemia is found in clinical conditions associated with a perception of volume depletion by the body, described as a decrease in effective circulating blood volume. Examples include heart failure, severe liver disease, nephrotic syndrome, or advanced renal failure. These patients often have ascites or clinical evidence of edema. Decreased renal blood flow in these patients stimulates the body’s regulatory mechanisms for volume expansion, resulting in decreased water excretion. Baroreceptors sense an ineffective circulating fluid volume and activate antidiuretic hormone and thirst mechanisms to retain free water, resulting in low serum sodium concentrations.18,26,33,34,86 Hyponatremia in conditions of normovolemia is uncommon but can be due to psychogenic polydipsia or the syndrome of inappropriate antidiuretic hormone release (SIADH), antidiuretic drugs (narcotics, nonsteroidal antiinflammatory drugs, barbiturates, vincristine), hypothyroid-induced myxedema coma, or administration of hypotonic fluids (iatrogenic). SIADH refers to antidiuretic hormone release in the absence of hyperosmolarity or hypovolemia. In human patients, SIADH occurs secondary to certain drugs, pulmonary disease, various malignancies, or central nervous system disease, but has been described only occasionally in dogs. Antidiuretic drugs result in increased antidiuretic hormone release or increased sensitivity of the kidney to the action of antidiuretic hormone, resulting in increased water retention and lower sodium concentrations. The exact mechanism of hyponatremia in myxedema coma is unclear but may be related to decreased distal tubular fluid delivery, as well as nonosmotic stimulation of antidiuretic hormone release and water retention. Some animals with hypoadrenocorticism will develop hyponatremia but will maintain normal hydration.18,26,22,34,86 Clinical signs of hyponatremia are related more to the rapidity of onset than to the degree of abnormality. Clinical signs of acute hyponatremia include central nervous system depression, ataxia, coma, or seizures secondary to cerebral edema as fluid from the extracellular space moves intracellularly. Clinical signs of chronic hyponatremia are similar but are rarely seen in small-animal medicine. Signs of cerebral edema most commonly develop at concentrations less than 120 mEq/L, or with rates of decrease greater than 0.5 mEq/L/hr.18,26,33,34,86 Asymptomatic chronic hyponatremia can be treated with careful water restriction and close monitoring of sodium concentrations. Symptomatic chronic hyponatremia can be corrected using crystalloid solutions with frequent monitoring of sodium concentrations. Chronic hyponatremia should be corrected very slowly, as increasing the sodium concentration more quickly than 0.5 mEq/L/hr may result in osmotic demyelination syndrome of the central nervous system, also known as central pontine myelinolysis.17,33,34,83,86 Hypernatremia is defined as a serum sodium concentration greater than 150 mEq/L in dogs and greater than 160 mEq/L in cats. Causes of hypernatremia are listed in Box 5-2. Hypernatremia results from free water loss or increased sodium ingestion. Increased sodium ingestion is uncommon but can include causes such as salt ingestion, homemade play dough ingestion, or iatrogenic administration of sodium-containing fluids (hypertonic saline, sodium bicarbonate). Ingestion of sodium-containing substances results in hypervolemic hypernatremia, as the increased extracellular osmolarity shifts fluid from the intracellular space into the extracellular space. Hyperaldosteronism and hyperadrenocorticism are disease processes that may result in hypervolemic hypernatremia.5,18,25,33,49,60,65,86,101 Free water losses can be divided into hypotonic water loss and pure water loss. Hypotonic water losses result in hypovolemic hypernatremia. Both water and electrolytes are lost through vomiting, diarrhea, osmotic diuresis, or third space fluid losses. Ingestion of paintballs has been reported to cause hypernatremia secondary to hypotonic fluid losses into the gastrointestinal tract from the osmotically active ingredients in the balls. Because electrolytes are lost from the extracellular space along with water, less osmotic pull is present for fluid to move from the intracellular space to the extracellular space, and hypovolemia results. Physical examination findings consistent with hypovolemia can help determine the possible causes of hypernatremia.25,33,49,60,86,101 Pure water loss is less common and usually does not result in hypovolemia because as the osmolarity of the extracellular space increases, water shifts from the intracellular to the extracellular space, preventing hypovolemia. Pure water loss can occur in patients with central or nephrogenic diabetes insipidus, heatstroke, burns, fever, lack of access to water, or hypodipsia.25,33,49,60,86,101 In animals with hypotonic or pure water losses, administration of isotonic sodium-containing fluids may cause a rise in serum sodium concentration if access to or ability to consume water is limited, or if sodium-water regulatory mechanisms are not functioning properly. This is particularly important in critically ill patients with large fluid losses, such as those with peritonitis or renal disease, or in patients with head trauma who may have impaired thirst mechanisms. An acute rise in serum sodium in patients receiving intravenous fluids can be avoided by frequent monitoring of serum sodium levels and adjustment of fluid therapy accordingly.66,101 Clinical signs of hypernatremia usually are not seen until the serum sodium concentration exceeds 170 mEq/L in dogs and 175 mEq/L in cats. Clinical signs are related more to the rapidity of onset of hypernatremia than to the degree of hypernatremia. Clinical signs are related to neuronal dehydration secondary to fluid shifts out of the intracellular compartment. Slowly developing, chronic hypernatremia allows neuronal cells to accumulate idiogenic osmoles (e.g., amino acids, inositol) to maintain intracellular osmolarity similar to that of the extracellular compartment and to prevent fluid from moving out of the cell. Tissue shrinkage can result in vascular damage and hemorrhage within the brain. Neuronal dehydration and intracranial hemorrhage may result in such clinical signs as anorexia, lethargy, vomiting, muscle weakness, vocalization, ataxia, seizures, coma, or death. Patients with hypovolemic hypernatremia will present with clinical signs of hypovolemia as well.18,25,33,49,60,65,66,86,88,101 Free water is most commonly replaced using 5% dextrose in water intravenously, as the dextrose is rapidly metabolized, effectively leaving only free water. Alternatively, water can be given orally or through a feeding tube, but caution should be exercised to prevent rapid shifts in osmolarity. Loop diuretics can facilitate natriuresis, particularly in animals with hypervolemic hypernatremia.5,18,33,49,60,65,66,86,88 Potassium is the major intracellular cation in the body, and approximately 95% of total body potassium is contained in the cells. High intracellular concentrations of potassium are maintained by the Na+-K+/ATPase pump in the cell membrane. Maintenance of high intracellular potassium concentrations relative to extracellular concentrations is critical to cell resting membrane potential and neuromuscular transmission, particularly in excitable muscle and cardiac cells. Because the intracellular concentration is much greater than the extracellular concentration, potassium moves down the concentration gradient across the cell membrane and into the extracellular space. As potassium moves out of the cell, it does not take an anion with it, creating a net negative charge within the cell. This negative charge inside the cell relative to the outside of the cell is the resting membrane potential and is typically −90 mV.31,68,69,86,87 Extracellular potassium concentration is strictly regulated between 3.5 and 5.5 mEq/L because changes in extracellular potassium concentration affect cell membrane potential and therefore excitability and muscle contraction. Potassium balance in the body is a function of intake through the gastrointestinal tract, excretion via the kidneys under the influence of aldosterone, and transcellular distribution. The kidneys are so efficient at excreting potassium that hyperkalemia is uncommon if kidney function and urine output are normal. Potassium shifts from the extracellular to the intracellular space in the presence of glucose, insulin, catecholamines, and metabolic alkaloses. Potassium moves extracellularly as the result of nonorganic metabolic acidosis or hyperosmolarity.31,68,69,86,87 Hypokalemia may be due to decreased total body potassium or translocation of potassium from extracellular to intracellular space. Causes of hypokalemia are listed in Box 5-3. Total body potassium depletion results from decreased intake or more commonly from increased renal or gastrointestinal losses. Decreased intake of potassium may be iatrogenically induced through administration of potassium-free fluids or glucose-containing fluids to an anorexic patient. Excessive loss of potassium via the gastrointestinal tract is common during vomiting of large volumes of gastric fluid (or removal of gastric fluid via a nasogastric or gastrostomy tube). Gastric fluid contains potassium ions along with hydrochloric acid (HCl) and sodium ions. The loss of gastric fluid and electrolytes results in sodium retention by the kidneys. In the face of low chloride levels, the kidney must reabsorb sodium in exchange for hydrogen and potassium ions, rather than with chloride, perpetuating the hypokalemia and metabolic alkalosis (this phenomenon is termed paradoxical aciduria). For example, hypokalemia is a common finding in dogs with gastrointestinal foreign bodies.15 Excessive renal losses are most commonly due to chronic renal failure, postobstructive diuresis, renal tubular acidosis, hyperaldosteronism, hyperadrenocorticism, hyperthyroidism, hypokalemic periodic paralysis, or drug administration (e.g., diuretics, penicillins, amphotericin B, β-adrenergic agonists).80 Hypokalemia appears to be more commonly recognized in cats than in dogs with chronic renal failure, and it may contribute to a further decline in renal function. Hypomagnesemia may also lead to a refractory hypokalemia secondary to intracellular efflux of potassium and subsequent renal excretion.31,69,86,87,89
Fluid Therapy
Body Fluid Compartments and Rehydration Versus Resuscitation
PERCENT DEHYDRATION
CLINICAL SIGNS
<5
No detectable abnormalities
5-8
Decreased skin turgor, dry mucous membranes
8-10
Decreased skin turgor, dry mucous membranes, eyes may be sunken in orbits, slight prolongation of capillary refill time
10-12
Severe skin tenting, prolonged capillary refill time, dry mucous membranes, eyes sunken in orbits, possibly signs of shock
>12
All of the above, plus signs of shock, often life threatening
Perioperative Fluid Therapy
Fluid Types and Uses
Isotonic Crystalloids
Hypotonic Solutions
Hypertonic Solutions
Synthetic Colloid Solutions
Hypertonic Saline/Colloid Solutions
Hemoglobin-Based Oxygen-Carrying Fluids
Blood Products
Blood Storage and Administration
Electrolytes
Hyponatremia
Hypernatremia
Potassium
Hypokalemia
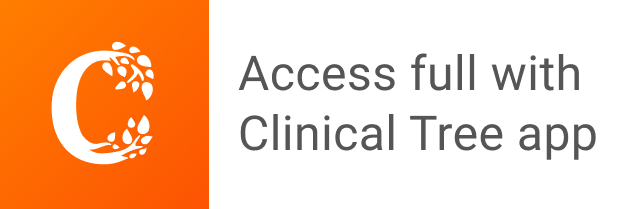