Chapter 17 Fluid, Electrolyte, and Acid-Base Balance II. PHYSIOLOGY OF FLUID AND ELECTROLYTE BALANCE IV. REGULATION OF BODY FLUIDS AND ELECTROLYTES A. Effective Circulating Volume V. PHYSIOLOGY OF ACID-BASE BALANCE H. Buffer Base, Standard Bicarbonate, and Base Excess or Base Deficit I. Nontraditional or Strong Ion Approach to Acid-Base Balance J. Dietary Factors in Acid-Base Balance VII. CLINICAL FEATURES OF FLUID AND ELECTROLYTE BALANCE VIII. CLINICOPATHOLOGICAL INDICATORS OF FLUID AND ELECTROLYTE IMBALANCE A. Packed Cell Volume and Total Plasma Protein The body fluids are arranged in dynamic, but orderly, functional compartments. Maintenance of these compartments in terms of volume and composition is essential for sustaining normal physiological and biochemical events. The electrolytes dissolved in body fluids fulfill vital roles in virtually all of life’s processes. Transmembrane movements of electrolytes are responsible for the electrical events that result in nerve conduction and muscular contraction, and the electrical stability of membranes is highly dependent on the concentration of electrolytes on both sides. Electrolytes also serve as essential cofactors in many enzymatically mediated metabolic reactions. The pH of body fluids is maintained within narrow limits. This fine control is necessary to maintain the structure and function of proteins essential for normal progression of metabolic events. Virtually every organ system participates in the maintenance of fluid and electrolyte balance or is adversely affected by imbalances. In many disease states, impaired fluid intake, excessive fluid losses, or organ damage and dysfunction will lead to a state of altered fluid and electrolyte balance. Regardless of whether fluid and electrolyte alterations are the primary problem or simply represent secondary manifestations of some other disease process, successful patient management depends on correct evaluation and appropriate therapy. To achieve this goal, one must have a clear understanding of the anatomy and physiology of the body fluids, the pathological mechanisms by which normal processes become deranged, the means by which these disturbances can be identified accurately, and finally the procedures that can be used to correct such disturbances in a prompt, safe, and effective manner (Tasker, 1980). A variety of units have been used in the quantitative evaluation of biological specimens. To avoid confusion, this chapter describes the units of measure that apply directly to the body fluids and electrolytes. An international standard for clinical chemistry units, the “Systeme Internationale d’Unites” (SI units), was developed to provide consistent terminology and usage. Although SI units are the international standard, they may not be familiar to all students or clinicians. All solute concentrations are expressed in moles or millimoles per l, blood gas partial pressure in kilopascals, and osmolality in millikelvins of freezing point depression. Electrolytes are substances that exist as positive or negative charged particles in aqueous solution. The positively charged particles are “cations,” and the negatively charged particles are “anions.” For univalent ions such as sodium, potassium, chloride, and bicarbonate, 1 mole equals 1 equivalent. For multivalent ions, 1 equivalent is equal to the molecular weight in grams (i.e., 1 mole) divided by the charge on the particle. To maintain electrical neutrality in biological fluids, there must be an equal number of equivalents or milliequivalents of anions and cations in solution. Electrolytes in solution combine equivalent for equivalent, not on a gram for gram or mole for mole basis. The osmotic properties of a solute in solution are related to the number of particles in solution and not to its weight or its charge. One osmole of a nondissociable substance is equal to its molecular weight in grams. One osmole of any substance that dissociates in solution into two or more particles is equal to the molecular weight in grams divided by the number of particles into which each molecule dissociates. Osmolarity is defined as the number of osmoles per l of final solution, whereas osmolality is the number of osmoles per kilogram of water. Although the expressions are similar, osmolality more correctly describes the osmotic properties as measured in the clinical laboratory. Most solutes in biological fluids are present in relatively dilute concentrations, and it is more convenient to express these concentrations as millimoles, milliequivalents, or milliosmoles. These simply represent one-thousandth of the standard unit. Conventional terms are milligrams per deciliter (mg/dl), millimoles per l (mmol/l), milliequivalent/l (mEq/l), and milliosmole per kg water (mOsm/kg). The concentrations of the principal anions and cations in plasma are presented in Table 17-1 as expressed in these conventional terms. Before discussing the assessment of fluid deficits or imbalances, it is necessary to consider the organization and composition of the fluid compartments from which these losses occur. An understanding of the forces that govern the relative volume and composition of the body fluid compartments is central to understanding both the clinical and clinicopathological manifestations of altered fluid balance. Water is the most abundant compound in the body, and most of life’s essential processes take place in this aqueous environment. Although there is substantial variation, the total body water (TBW) of most domestic animals is approximately 60% of body weight (0.60 l/kg). In a 500-kg horse, this amounts to approximately 300 l (Carlson, 1983c), whereas in a 20-kg dog, it amounts to just over 12 l (Kohn and DiBartola, 1992). Adipose tissue contains little water, and the amount of body fat has a major impact on the relative TBW. The average body water content of women is 0.45 to 0.50 l/kg as compared to 0.55 to 0.60 l/kg for men (Edelman et al., 1958; Elkinton and Danowski, 1955). This difference is largely the result of the larger fat deposits in the adult woman and the larger muscle mass of the adult man (Elkinton and Danowski, 1955). Clear sex-associated differences in body fat are not appreciated in domestic animals. However, certain species of domestic animals such as fattened swine or sheep have a large amount of body fat. Although lighter sheep had a TBW of near 0.65 l/kg (Wade and Sasser, 1970), the TBW of these fattened animals may be less than 0.50 l/kg (English, 1966b; Hansard, 1964), whereas the TBW of the athletic horse is generally greater than 0.65 l/kg (Dieterich and Holleman, 1973; Judson and Mooney, 1983; Robb et al., 1972). The relative water content of newborn animals is much higher than adults. Data in human infants, calves, foals, and lambs suggest a water content in excess of 75% of body weight at birth (Bennett, 1975; Dalton, 1964; Edelman and Leibman, 1959; Phillips et al., 1971; Pownall and Dalton, 1973). The large TBW is primarily the result of the very large extracellular fluid (ECF) volume, which exceeds 0.40 l/kg at birth in most species (Bennett, 1975; Kami et al., 1984; Spensley et al., 1987; Tollertz, 1964). There is an initial rapid decline during the first few days to weeks of life with TBW, and ECF volumes approach adult levels by 6 months of age (Spensley et al., 1987). The TBW consists of two major compartments, the intracellular fluid (ICF) volume and the ECF volume. The distribution of body water is illustrated in Figure 17-1 indicating the normal fluid balance of a 450-kg horse. The ICF accounts for approximately one-half to two-thirds of the TBW, and the ECF accounts for the remainder. Although these two compartments differ markedly in electrolyte composition, they are in osmotic equilibrium, and water is freely diffusible between them. The relative volume distribution of water between these two compartments is largely governed by the number of osmotically active particles in each compartment. The ECF sodium content determines the ECF volume, whereas ICF volume is a function of ICF potassium content. The relationship between the exchangeable cation content (sodium in the ECF, potassium in the ICF) and the total body water was defined by Edelman et al. (1958): TABLE 17-1 Plasma Electrolyte Concentrations Expressed in Different Unitsa aFrom Tasker (1980). bOnly ionized calcium and magnesium have been considered here. cPhosphate concentration is that of organic phosphorus. Because a variable equilibrium exists between FIGURE 17-1 Body fluid compartments in a normal 450-kg horse. Serum sodium concentration is 140 mEq/l (140 mmol/l). Extracellular fluid (ECF) volume is 1001 and intracellular fluid (ICF) volume is 2001. Because most cell membranes are very permeable to water, there are no major osmotic gradients between the ECF and the ICF, and serum sodium concentration and osmolality reflect the osmolality of the ICF compartment as well as that of the ECF compartment (Edelman et al., 1958; Saxton and Seldin, 1986; Scribner, 1969). The ECF should be viewed as a physiological rather than a strictly definable anatomical space (Carlson et al., 1979a). The ECF volume of adult animals ranges from 0.15 to 0.30 l/kg body weight (Carlson et al., 1979a; English, 1966b; Evans, 1971; Hankes et al., 1973; Hix et al., 1953; Kohn, 1979; Spurlock et al., 1985; Thornton and English, 1977; Zweens et al., 1975), depending on the species and the volume dilution procedure used. Regulation of ECF volume is a complex process in which a variety of factors interact. The ECF consists of all the fluids located outside the cells and includes the plasma (0.05 l/kg), interstitial fluid and lymph (0.15 l/kg), and the transcellular fluids (Edelman and Leibman, 1959; Rose, 1984; Saxton and Seldin, 1986). The transcellular fluids, which include the fluid content of the gastrointestinal tract, are generally considered a subcomponent of the ECF. In small animal species, the fluid content of the gastrointestinal tract is relatively small (Strombeck, 1979). In the large animal herbivore species, a substantial volume of fluid is normally present within the gastrointestinal tract. In the horse, this may amount to 30 to 45 l (Carlson, 1979a), and in cattle, the forestomach may contain as much as 30 to 60 l of fluid (Phillipson, 1977). During periods of water restriction and certain other forms of dehydration, this gastrointestinal fluid reservoir can be called on to help maintain effective circulating volume (McDougall et al., 1974). All of the fluids of the ECF contain sodium in approximate concentrations of 130 to 150 mEq/l H2O. Sodium provides the osmotic skeleton for the ECF, and the sodium content is the single most important determinant of ECF volume (Rose, 1984). Sodium deficits result in decreases in ECF volume, whereas sodium excess is most often associated with water retention and results in edema (McKeown, 1986; Rose, 1984). The ICF volume represents the fluid content within the body’s cells. This volume cannot be measured directly but is calculated as the difference between the measured TBW and the measured ECF volume. Potassium provides the osmotic skeleton for the ICF in much the same way that sodium provides the osmotic skeleton for the ECF. Because water is freely diffusible into and out of the cell, changes in the tonicity of the ECF are rapidly reflected by similar changes in ICF tonicity (Saxton and Seldin, 1986). This is largely the result of the movement of water across the cell membrane with resultant changes of ICF volume. Thus, whereas plasma sodium concentration decreases in response to water retention, ICF volume increases (Humes, 1986). On the other hand, with water depletion resulting in hypernatremia, ICF volume decreases (Humes, 1986). Relatively little is known about the organization of intracellular water into the various subcellular compartments and organelles. The effective circulating volume refers to that part of the ECF that is within the vascular space and is effectively perfusing the tissues (Rose, 1984). Effective circulating volume tends to vary with ECF volume, and both parameters vary with the total body sodium stores (Rose, 1984). Sodium loading produces volume expansion, whereas sodium depletion leads to volume depletion. Effective circulating volume is not a quantitatively measurable entity but refers to the rate of perfusion of the capillary circulation. Effective circulating volume is maintained by varying vascular resistance, cardiac output, as well as renal sodium and water excretion (Rose, 1984). Decreases in effective circulating volume result in decreased venous return, decreased cardiac output, and decreased blood pressure. Decreased volume and pressure are recognized by special volume receptors in the cardiopulmonary circulation and kidney, which trigger increased sympathetic tone resulting in increased arterial and venous constriction as well as increased cardiac contractility and heart rate. These responses tend to correct for the volume deficit by increasing cardiac output and systemic blood pressure. Volume and pressure changes associated with decreases in effective circulating volume also result in activation of the renin-angiotensin system with subsequent enhancement of aldosterone secretion by the adrenal cortex (Brobst, 1984). Aldosterone acts to enhance renal sodium resorption, which is a critical factor for maintaining and eventually restoring effective circulating volume. Additional factors that influence sodium resorption in response to changes in fluid volume include alterations in glomerular filtration rate, renal hemodynamics, atrial natriuretic factor, and plasma sodium concentration. Antidiuretic hormone (ADH) plays a primary role in the regulation of the osmolality of the body fluids. Antidiuretic hormone is synthesized in the hypothalamus, stored in the neurohypophysis, and released in response to changes in plasma osmolality. Because sodium concentration is the primary determinant of plasma osmolality, ADH-release is closely correlated to plasma sodium concentration. Special sensors in the hypothalamus recognize increases in plasma osmolality, and the normal response is increased thirst to enhance water intake and the release of ADH, which increases water reabsorption by the renal collecting tubules. Antidiuretic hormone exerts its activity on the collecting tubules by activating adenyl cyclase; this results in the generation of cyclic adenosine monophosphate (cyclic AMP) and protein kinases, which in turn alter the permeability of the tubules to water (Rose, 1984). Antidiuretic hormone also is released in response to decreases in effective circulating fluid volume, although the renin-angiotensin system exerts primary control over volume changes. Antidiuretic hormone acts extrarenally as an arterial vasoconstrictor, thus increasing blood pressure. Plasma osmolality decreases in response to a water load, and ADH release is inhibited. The resultant reduction in ADH-mediated reabsorption of water in the collecting tubules allows for appropriate renal excretion of the water load and a return of plasma osmolality toward normal. This highly sensitive system responds rapidly to small changes in osmolality, and as a result, plasma osmolality is normally maintained within a relatively narrow range. The renin-angiotensin system plays an important role in the maintenance of effective circulating fluid volume. Renin is a proteolytic enzyme produced by special juxtaglomerular cells of the glomerular afferent arteriole. Renin is released in response to reduced renal perfusion produced by hypotension, volume depletion, or increased sympathetic activity. Renin converts the circulating globulin angiotensinogen to angiotensin I, which is subsequently converted by an enzyme in the lung and vascular endothelial cells to the biologically active form, angiotensin II. Angiotensin II exerts a variety of systemic effects that tend to correct hypovolemia and hypotension. Angiotensin II increases renal retention of sodium and water by enhancing secretion of aldosterone from the adrenal cortex as well as having direct effects on the renal tubule. Angiotensin II exerts hemodynamic effects, which tend to increase blood pressure by inducing arteriolar vasoconstriction. Aldosterone plays a central role in the maintenance of effective circulating fluid volume and potassium balance largely through its effects on renal resorption of sodium in exchange for potassium and hydrogen ion. Aldosterone is produced in the adrenal cortex and exerts its effects on sensitive cells, such as the renal collecting tubules, by interacting with specific cytoplasmic receptors. The aldosterone receptor complexes subsequently enhance RNA-mediated production of specific proteins, which actually mediate the physiological effects of the hormone. There is evidence for aldosterone-mediated effects on gastrointestinal sodium and potassium absorption as well as effects on sweat glands to alter the electrolyte composition of sweat in response to sodium depletion (Michell, 1974). Aldosterone secretion is enhanced by the renin-angiotensin system in response to changes in effective circulating fluid volume. Atrial natriuretic factor (ANF), also known as atrial natriuretic peptide (ANP), exists as a group of diverse peptide hormones produced in the heart and released into the circulation in response to processes that increase central venous pressure and thereby stretch the atrial wall (Inagami, 1994). The actions of ANF that tend to reduce cardiac output and systemic blood pressure are mediated by transmembrane receptors, which result in the production of cyclic guanidine monophosphate generated by guanylyl cyclase (Inagami, 1994). ANF results in natriuresis and diuresis by the kidneys. ANF also causes vasodilatation and reduces fluid volume by acting directly on vascular smooth muscle and inhibiting the release of aldosterone from the adrenal cortex and norepinephrine from peripheral adrenergic neurons. Additionally, ANF has been found in the brain, and centrally medicated effects on fluid volume regulation may be important. Elevated plasma ANF has been noted in humans with a variety of diseases ranging from congestive heart failure to obstructive lung disease to chronic renal failure. However, the physiological importance of ANF in these disease processes is not completely resolved. In humans, Bartter’s syndrome and Gordon’s syndrome are thought to be due to an excess or deficiency of ANF, respectively (Christensen, 1993). In horses, the elevation of plasma ANF has been associated with treadmill exercise (McKeever et al., 1991). The hydrogen ion concentration of the ECF is maintained within remarkably narrow limits and is normally approximately 40 nmol/l. This concentration is roughly one-millionth the concentration of other common electrolytes. Even at these extremely low concentrations, hydrogen ions have profound effects on metabolic events largely through interaction with cellular proteins. These interactions alter protein configuration and thus alter protein function. Most enzymatic reactions have a narrowly defined range of pH optimum, and changes in hydrogen ion concentration have direct effects on the rates of reaction and, thus, many basic biological processes. Although the hydrogen ion concentration can be expressed in nmol/l a more useful expression is that of pH. The pH of a solution is equal to the negative logarithm of the hydrogen ion concentration. It is important to remember that the pH varies inversely with hydrogen ion concentration. When hydrogen concentration in the blood increases, pH decreases, and the animal develops an acidosis. When the hydrogen ion concentration in the blood decreases, the pH rises, and the animal develops an alkalosis. The traditional view of acid-base balance involves the following: 1. Extracellular and intracellular buffering 2. Regulation of the rate of alveolar ventilation to control carbon dioxide concentration 3. Regulation of renal hydrogen excretion A buffer system consists of a weakly dissociated acid and the salt of that acid. The body buffers are able to take up or release hydrogen ions so that changes in hydrogen ion concentration are minimized. According to the law of mass action, the dissociation of a weak acid, HA, into H+ and A– can be written where Ka is the dissociation constant for the reaction. The equation can be rewritten Taking the negative logarithm of both sides of the equation yields Substituting pH for –log [H+], log [A–]/[HA] for –log [HA]/[A–] and defining pKa as –log Ka, the formula now reads This formula is the familiar Henderson-Hasselbalch equation for the dissociation of a weak acid. The buffering capacity of the body includes the extracellular buffers, intracellular buffers, and bone. The extracellular buffers include the bicarbonate (HCO3/H2CO3) and phosphate (HPO4/ Large amounts of carbon dioxide are produced by oxidative metabolism each day. Although carbon dioxide is not an acid, it combines with water as it is added to the bloodstream resulting in the formation of carbonic acid. The enzyme carbonic anhydrase in red blood cells and other cells facilitates this reaction: The ensuing elevation in the hydrogen concentration is minimized because most of the excess H+ ions combine with the intracellular buffers, particularly hemoglobin (Hb): The bicarbonate generated by this reaction then leaves the erythrocyte and enters the extracellular fluid in exchange for extracellular chloride ion. The net effect is that CO2 is primarily carried in the venous circulation as From a clinical and physiological standpoint, the bicarbonate-carbonic acid buffer pair is clearly the most important. Bicarbonate is present in relatively high concentrations, it is relatively easy to measure, and it is the buffer system over which the body has the greatest control. The Henderson-Hasselbalch equation applied to this buffer pair becomes where 6.1 = pKa for the Plasma pH is determined by the concentration of bicarbonate and carbonic acid or, more importantly, the ratio between bicarbonate and carbonic acid. An equilibrium exists between the partial pressure of CO2 in the alveolar air, the partial pressure of gaseous CO2 dissolved in the blood, and the carbonic acid concentration of the blood. The conventional method of evaluating carbonic acid is the determination of the partial pressure of carbon dioxide (pCO2) in the blood. The carbonic acid concentration can be calculated by multiplying pCO2 by 0.03 (0.03 is the solubility constant for carbon dioxide in plasma). The Henderson-Hasselbalch equation for this buffer pair then becomes The four primary acid-base imbalances and their compensating responses are presented in Table 17-2. Acidosis is associated with a decrease in pH resulting from an increase in hydrogen ion concentration. Alkalosis is due to a decrease in hydrogen ion concentration, which is reflected by an increase in pH. In the metabolic disorders, the primary imbalance is due to changes in bicarbonate concentration. The compensating response is mediated by the respiratory system, which, within limits, alters the pCO2 so as to counterbalance the primary imbalance and to partially restore the pH toward normal. As the name implies, the primary imbalances of the respiratory disorders are related to alterations in alveolar ventilation, which result in increases in pCO2 in respiratory acidosis and decreases in pCO2 with respiratory alkalosis. The compensating responses for these primary respiratory imbalances are mediated by the kidney through alterations in the excretion or retention of hydrogen ions or bicarbonate. Blood samples drawn for acid-base evaluation must be drawn anaerobically and sealed so as to avoid alteration in the blood gas tension. Heparin is the anticoagulant of choice. The rectal temperature of the patient should be taken so that appropriate temperature corrections can be made. For evaluation of blood gases during intense exercise, central blood temperature should be used for this correction because rectal temperature may not accurately reflect blood temperature under these non-steady-state conditions. The temperature correction usually has a greater impact on the pO2 and pCO2 than bicarbonate or base balance. Arterial blood samples are generally preferred and are essential for the evaluation of primary respiratory disorders or the patient’s status under general anesthesia. Venous blood samples provide reliable data on metabolic acid-base abnormalities, and because they are easier to obtain, they are routinely used. Acid-base parameters of venous blood are significantly influenced by the acid-base status of the tissues from which the blood is draining. Central veins (e.g., vena cava) provide blood that better reflects the acid-base status of the body as a whole. As a general rule, blood gas determination should be made as soon after collection as possible. However, appropriately collected blood samples can be held in ice water for as long as 4 h and still yield reliable results. The effects of various sampling sites (arterial, venous, and capillary blood) on blood gas determination have been evaluated in dogs (Ilkiw et al., 1991; van Sluijs et al., 1983), horses (Littlejohn and Mitchell, 1969; Speirs, 1980), and swine (Hannon et al., 1990). Consistent differences were demonstrated between arterial and venous blood. Arterial blood samples yield higher values for pH and lower values for pCO2 and bicarbonate than venous blood, but calculated base balances tend to be similar for both arterial and venous blood samples. In horses, venous-arterial differences in bicarbonate can exceed 10 mEq/l during intense exercise. The higher venous bicarbonate reflects the important role of bicarbonate as a means of CO2 transport in the venous circulation (Carlson, 1995). At rest and during exercise, more than 70% of the CO2 produced in the tissues is transported in the venous circulation to the lungs as bicarbonate. This process is greatly facilitated by erythrocyte carbonic anhydrase and the mechanism of the “chloride shift.” The effects of temperature on oxygen content of dog blood have been studied (Hedley-Whyte and Laver, 1964), as has the oxygen affinity and Bohr coefficient of dog blood (Reeves et al., 1982). Recumbency in neonatal patients and body position during general anesthesia may have a significant impact on both arterial and mixed venous blood gas data (Madigan et al., 1992; Mason et al., 1987; Steffey et al., 1977). Nomograms have been developed relating the effects of temperature, CO2 content, and hemoglobin saturation for dog blood (Rossing and Cain, 1966). The use of blood gas data for the evaluation of acid-base imbalances for clinical problems has been reviewed (DiBartola, 1992a, 1992b, 1992c; George, 1994; de Morais, 1992a, 1992b). Metabolic acidosis is characterized by a decrease in pH and bicarbonate. Metabolic acidosis, as traditionally viewed, can be produced by the addition of hydrogen ions or a loss of bicarbonate ions. The initial buffering of an acid load is by the ECF buffers, primarily the bicarbonate-carbonic acid buffer pair (Rose, 1984). Intracellular buffers, particularly protein and phosphate, assist in the buffering process. The intracellular movement of hydrogen in exchange for potassium helps to prevent an excessive increase of the ECF hydrogen ion concentration in the face of an acid load. This exchange is called the “cation shift” and can result in hyperkalemia even though the total body potassium stores have been depleted due to renal or gastrointestinal losses. a Causes of Metabolic Acidosis The most common causes include lactic acidosis; ketoacidosis; gastrointestinal problems, such as indigestion colic or diarrhea; and renal failure, which may result in a decreased ability to excrete hydrogen and thus to retain bicarbonate (Emmett and Nairns, 1977). It has long been held that the acidosis in calves associated with diarrhea was largely due to the loss of bicarbonate into the bowel. This view has been challenged in an elegant paper utilizing a quantitative strong ion approach to assess the mechanism of the acid-base abnormality (Constable et al., 2005). A profound metabolic acidosis without dehydration leading to depression, recumbency, and death has been described in goat kids (Tremblay et al., 1991), which appears to be similar to reports in calves (Kasari and Naylor, 1984, 1986). The cause was undetermined, but the acidosis was usually associated with an increased anion gap. Sodium bicarbonate therapy, if initiated early in the course of the disease, was often curative. The acidosis observed in these calves may have been associated with increases in both D and L isomers of lactate generated by bacterial fermentation as described in several recent clinical and research studies of calves with indigestion associated with milk ingestion (Ewaschuk et al., 2003, 2004; Lopez et al., 2004; Lorenz et al., 2005; Omole et al., 2001; Stampfli, 2005). Additional causes of a metabolic acidosis include ingestion of certain medications or toxic compounds such as salicylate, methanol, ethylene glycol, or paraldehyde, which result in the accumulation of exogenous anions (DiBartola, 1992b). b. Compensation A metabolic acidosis is recognized quickly, and the compensating respiratory response of increased ventilation will begin reduction of the pCO2 within minutes. In dogs, the anticipated respiratory response is a reduction of pCO2 by 0.7 mmHg for each mEq/l decrease in bicarbonate (de Morais, 1992a, 1992b). This minimizes the fall in pH, but the protective effects of the respiratory response are relatively short lived, lasting only a few days. Long-term correction of a metabolic acidosis requires renal bicarbonate retention and enhanced renal acid excretion, primarily as ammonium ion because there is little ability to increase the titratable acidity, which consists primarily of phosphate buffers (Rose, 1984). Complete correction of a metabolic acidosis may be difficult in patients with intrinsic renal disease or diseases that would impair the kidney’s ability to excrete acid or retain bicarbonate such as renal tubular acidosis. A respiratory acidosis is characterized by a decrease in pH and an increase in pCO2. Respiratory acidosis develops because of decreased effective alveolar ventilation or breathing an atmosphere with elevated CO2. The initial buffering of the acid load produced by a respiratory acidosis is almost exclusively by the intracellular buffers. The principal ECF buffer, the bicarbonate-carbonic acid buffer pair, cannot buffer a respiratory acidosis. Carbon dioxide diffuses through the lung much more readily than O2; thus, diseases that compromise ventilation normally result in decreases in pO2 before significant increases in pCO2 develop. The respiratory center is extremely sensitive to minor changes in pCO2, and increased pCO2 normally provides the major stimulus to ventilation (Rose, 1984). In contrast, hypoxemia does not begin to promote enhanced ventilation until the arterial pO2 is substantially decreased. If, however, the arterial pCO2 is held at normal values or is elevated because of intrinsic lung disease, then ventilation begins to be enhanced as the arterial pO2 falls below 70 to 80 mmHg (Rose, 1984). a. Causes of Respiratory Acidosis Any disorder that interferes with normal effective ventilation may produce a respiratory acidosis. The most common causes are primary pulmonary diseases ranging from acute upper respiratory obstruction, to pneumonia, to pneumothorax, and chronic obstructive lung disease. Diseases or drugs that affect the central nervous system may inhibit the medullary respiratory center and can produce a profound respiratory acidosis. An additional cause of special importance in veterinary medicine is general anesthesia with volatile agents using a closed system. Under these conditions, ventilation may be seriously reduced without producing hypoxia. The high oxygen content of the gas mixture maintains high pO2 in the blood, but depression of the respiratory center may result in insufficient alveolar ventilation so that CO2 accumulates. This problem can be overcome through the use of a positive pressure ventilatory apparatus and careful monitoring of arterial blood gases during general anesthesia. b. Compensation The compensating response for a respiratory acidosis is renal retention of bicarbonate and increased excretion of hydrogen ion. This response requires several days, and thus the response is seen only in a chronic respiratory acidosis. In dogs with chronic respiratory acidosis, a compensating increase of 0.35 mEq/l of bicarbonate is anticipated for each mmHg increase in pCO2 (de Morais, 1992a, 1992b). The extent of the rise in the plasma bicarbonate concentration in chronic respiratory acidosis is determined by increased renal hydrogen secretion (Rose, 1984). Exogenous bicarbonate is unnecessary, and should bicarbonate be administered to patients with a respiratory acidosis, it would be excreted without affecting the final plasma bicarbonate concentration. Metabolic alkalosis is characterized by an increase in pH and bicarbonate. Metabolic alkalosis occurs with some frequency in domestic animals and is commonly observed in association with digestive disturbances in ruminants. The development of a metabolic alkalosis requires an initiating process capable of generating an alkalosis and the additional factors that are necessary for maintaining the alkalosis (Rose, 1984). Generation of a metabolic alkalosis can be due to excessive hydrogen loss, bicarbonate retention, or as a contraction alkalosis. A contraction alkalosis occurs with reduction of ECF fluid volume resulting from a loss or sequestration of sodium and chloride containing fluid without commensurate loss of bicarbonate (Garella et al., 1975). Excessive hydrogen ion losses can result in a metabolic alkalosis. a. Causes of Metabolic Alkalosis The most common causes of increased hydrogen loss are gastrointestinal losses of chloride-rich fluids associated with vomiting in small animals (Strombeck, 1979) or sequestration of chloride-rich fluid in the abomasum and forestomach of ruminants (Gingerich and Murdick, 1975b; McGuirk and Butler, 1980). Excessive renal hydrogen loss associated with mineralocorticoid excess, diuretic usage (particularly the loop diuretics such as furosemide), and low chloride intake may cause or contribute to the generation of a metabolic alkalosis (Rose, 1984). Most of these disorders are also associated with the development of significant sodium and chloride deficits and resultant decreases in effective circulating volume. These deficits and the responses that decreased effective circulating volume induce are central features of the processes that maintain and perpetuate a metabolic alkalosis. Hydrogen loss from the ECF can also occur with hydrogen movement into the cells in response to potassium depletion (Irvine and Dow, 1968). Excessive bicarbonate administration is an additional potential cause of metabolic alkalosis. Most normal animals can tolerate large doses of bicarbonate, and excesses are rapidly eliminated by renal excretion (Rumbaugh et al., 1981). However, patients with decreases in effective circulating blood volume or with potassium or chloride deficits may not tolerate a bicarbonate load because renal clearance of excess bicarbonate is likely to be impaired. The factors that are responsible for the maintenance of a metabolic alkalosis all impair renal bicarbonate excretion. These factors may include decreased glomerular filtration of bicarbonate seen in some types of renal failure. However, the most common factor is increased renal tubular bicarbonate resorption, which is associated with the renal response to decreases in the effective circulating fluid volume, potassium depletion, or chloride depletion (Rose, 1984). Sodium resorption is enhanced in response to hypovolemia to help restore normal effective circulating fluid volume. The maintenance of electroneutrality requires that sodium resorption in the proximal tubule must be accompanied by a resorbable anion such as chloride, whereas in the distal tubule, sodium resorption is associated with the secretion of a cation, usually hydrogen or, to a lesser extent, potassium. The only resorbable anion normally present in appreciable quantities in the proximal tubular fluid is chloride. In a metabolic alkalosis, plasma bicarbonate is increased and chloride concentration is generally decreased as the result of disproportionately high chloride losses that result from vomiting, sequestration of gastric fluid (Whitlock et al., 1975b), diuretic usage, or heavy sweat losses in exercising horses (Carlson, 1975, 1979b). The relative lack of the resorbable anion, chloride, in the proximal tubule thus allows a larger amount of sodium to reach the distal tubule where the action of aldosterone enhances hydrogen loss into the tubular lumen in exchange for sodium. The maintenance of effective circulating volume is so critical that the body chooses to maintain circulating volume by enhanced sodium resorption by whatever means necessary, even at the expense of extracellular pH. Renal hydrogen excretion is directly linked with bicarbonate resorption. Thus, it is not possible to eliminate the excess bicarbonate, and the metabolic alkalosis is maintained (Rose, 1984). This mechanism is the reason for the paradoxic acid urine seen in some patients with metabolic alkalosis (Gingerich and Murdick, 1975a, 1975b; McGuirk and Butler, 1980). Hypokalemia is another factor that contributes to the maintenance of a metabolic alkalosis. Hypokalemia is associated with an increase in intracellular hydrogen ion concentration. Increased renal tubular cell hydrogen ion concentration may enhance hydrogen secretion and thus bicarbonate reabsorption by the tubular cells. b. Compensation Chemoreceptors in the respiratory center sense the alkalosis, and the respiratory response to a metabolic alkalosis is hypoventilation resulting in an increase in pCO2. In dogs, the expected compensating response is an increase of pCO2 of 0.7 mmHg for each mEq/l increase in bicarbonate. Respiratory alkalosis is associated with an increase in pH and a decrease in pCO2. a. Causes of Respiratory Alkalosis Respiratory alkalosis is due to hyperventilation, which may be stimulated by hypoxemia associated with pulmonary disease, congestive heart failure, or severe anemia. Hyperventilation may also be associated with psychogenic disturbances or neurological disorders that stimulate the medullary respiratory center such as salicylate intoxication or Gram-negative sepsis. Respiratory alkalosis may be seen in animals in pain or under psychological stress. Hyperventilation may occur in dogs and other nonsweating animals as they employ respiratory evaporative processes for heat loss to prevent overheating (Tasker, 1980). b. Compensation The initial compensating response to an acute respiratory alkalosis is a modest decline in ECF bicarbonate concentration as the result of cellular buffering. Subsequent renal responses result in decreased ECF bicarbonate concentration through reduced renal bicarbonate reabsorption. These responses require 2 to 3 days for completion. The decline in bicarbonate is partially offset by chloride retention in order to retain electroneutrality. Thus, hyperchloremia and decreased pCO2 may be associated with compensated respiratory alkalosis as well as compensated metabolic acidosis. Compensating responses for chronic respiratory alkalosis lasting several weeks may actually be sufficient to return pH to normal. In dogs, anticipated renal compensation for a chronic respiratory alkalosis results in a decrease of bicarbonate of 0.55 mEq/l for each mmHg decrease in pCO2 (de Morais, 1992a, 1992b). Mixed acid-base disorders occur when several primary acid-base imbalances coexist (de Morais, 1992a). Metabolic acidosis and alkalosis can coexist and either or sometimes both of these metabolic abnormalities may occur with either respiratory acidosis or alkalosis (Nairns and Emmett, 1980; Wilson and Green, 1985). Evaluation of mixed acid-base abnormalities requires an understanding of the anion gap, the relationship between the change in serum sodium and chloride concentration, and the limits of compensation for the primary acid-base imbalances (Saxton and Seldin, 1986; Wilson and Green, 1985). Clinical findings and history are also necessary to define the factors that may contribute to the development of mixed acid-base disorders. The following are important considerations in evaluating possible mixed acid-base disorders: 1. Compensating responses to primary acid-base disturbances do not result in overcompensation. 2. With the possible exception of chronic respiratory acidosis, compensating responses for primary acid-base disturbances rarely correct pH to normal. In patients with acid-base imbalances, a normal pH indicates a mixed acid-base disturbance. 3. A change in pH in the opposite direction to that predicted for a known primary disorder indicates a mixed disturbance. 4. With primary acid-base disturbances, bicarbonate and pCO2 always deviate in the same direction. If these parameters deviate in opposite directions, a mixed abnormality exists. Although mixed acid-base abnormalities undoubtedly occur in animals and have been documented in the veterinary literature, they are often overlooked (Wilson and Green, 1985). An appreciation of the potential for the development of mixed abnormalities is essential for the correct interpretation of clinical and clinicopathological data, which would otherwise be quite confusing. Care should be taken when evaluating suspected mixed acid-base abnormalities that sufficient time has elapsed so that anticipated compensating responses could have occurred (de Morais, 1992a). The anion gap can be calculated as the difference between the major cation (sodium) and the measured anions (chloride + bicarbonate) (Emmett and Narins, 1977). Some investigators prefer to use the following formula: TABLE 17-3 Causes of Alterations in Anion Gap The addition of potassium to the equation, however, adds little to the diagnostic utility of this calculation (Emmett and Narins, 1977; Epstein, 1984; Oh and Carroll, 1977); the anion gap calculated with the inclusion of potassium concentration will be about 4 mEq/l higher. Because most of the published data on the anion gap in animal species are the result of calculations using the second equation (Eq. 17–11), this form will be used in Table 17-3. Provided the component determinations are valid, the calculated anion gap provides an approximation of the so-called “unmeasured anions.” Normally, these unmeasured anions consist primarily of negatively charged plasma proteins because the charges of the unmeasured cations (potassium, calcium, and magnesium) tend to balance out the charges of the unmeasured anions (phosphate, sulfate, and organic ions). The anion gap is most useful in situations where the concentrations of phosphate and plasma proteins, particularly albumin, are within the normal range (see Section V.I). The anion gap for most species of domestic animals appears to be similar to that defined for human subjects (i.e., approximately 10 to 20 mEq/l) (10 to 20 mmol/l). However, there do appear to be significant differences in the normal rang of the anion gap of different species as indicated in Table 17-4 (Adrogue et al., 1978; Bristol, 1982; Feldman and Rosenberg, 1981; Gossett and French, 1983; Polzin et al., 1982; Shull, 1978, 1981). Age-related changes in anion gap have been reported in horses (Gossett and French, 1983), with young foals having a significantly larger anion gap than adults. Further experimental data will be necessary to more clearly establish the normal range for the anion gap of animals under varying conditions. The simple calculation of anion gap can be employed in the categorization of acid-base disorders with regard to potential causal factors and may serve as a prognostic guide in a variety of circumstances (Bristol, 1982; Garry and Rings, 1987; Shull, 1978). Decreases in anion gap can be seen with increases in cationic proteins associated with polyclonal gammopathy or multiple myeloma. Decreases in anion gap resulting from decreases in unmeasured anions occur most commonly with hypoalbuminemia and hyperchloremic metabolic acidosis, but they also may be noted with overhydration. The causal factors associated with a hyperchloremic metabolic acidosis with a normal to low anion gap can often be differentiated based on the serum potassium concentration. Hyperchloremic metabolic acidosis associated with gastrointestinal fluid losses from diarrhea or renal causes such as renal tubular acidosis most often manifests a hypokalemia (Saxton and Seldin, 1986; Ziemer et al., 1987a, 1987b). Hyperchloremic metabolic acidosis associated with decreased mineralocorticoid secretion or activity such as seen in Addison’s disease or renal failure generally presents with a hyperkalemia (Saxton and Seldin, 1986). There are indications that changes in hydrogen ion concentration may alter protein equivalency and thus alter the anion gap in either an acidosis or alkalosis (Adrogue et al., 1978; Madias et al., 1979). Dehydration and alkalosis are potential, but minor, causes of increased anion gap. Most commonly, elevations of anion gap are associated with the development of a metabolic acidosis in which there is an increase in anions, which are not routinely measured in the clinical laboratory. This is called a high anion gap acidosis and may be associated with an accumulation of metabolizable acids as in a lactic acidosis associated with anaerobic exercise, grain overload, or hypovolemic shock or ketoacidosis resulting from diabetes or ketosis or with the accumulation of nonmetabolizable acids as in uremic acidosis or various intoxications (see Table 17-3). The presence of a metabolic acidosis with a high anion gap thus provides grounds to undertake a thorough investigation of disease processes capable of producing an accumulation of these unmeasured anions. The anion gap also may be useful in the identification of mixed acid-base imbalances. When the change in the anion gap does not approximate the change in bicarbonate, a mixed metabolic acid-base imbalance should be suspected. In cases of grain overload in herbivores, a large ion gap may be due to increased ECF levels of D-lactic acid, which is not detected by the usual assays for lactic acid because they detect only the L-isomer produced in mammalian metabolism. Either a special assay for D-lactate must be performed, or an increased level of D-lactate may be assumed based on history and other clinical data. If respiratory disturbances can be eliminated, the metabolic component of acid-base balance is indicated by the bicarbonate concentration. Bicarbonate is usually estimated by determination of the “CO2 content” or “total CO2” of plasma or serum samples. Bicarbonate actually accounts for approximately 95% of the measured total CO2, and thus the total CO2 provides a measure of metabolic changes in acid-base balance. The bicarbonate determined in this fashion will be decreased in a metabolic acidosis and increased in a metabolic alkalosis. Estimates of bicarbonate are often provided in automated chemistry profiles. These determinations may indicate the metabolic acid-base status. However, if acid-base abnormalities are suspected, a proper blood gas evaluation should be undertaken. These values are mathematically derived from the measurements of blood pH and pCO2 and provide an indication of the metabolic component of acid-base balance. It should be noted that the metabolic changes indicated by these parameters do not always reflect the primary acid-base imbalances but may represent compensating responses for primary respiratory disorders. The buffer base indicates the sum of all the buffer anions in blood under standardized conditions. The standard bicarbonate is the plasma bicarbonate concentration that would be found under specific conditions, which eliminate respiratory influences on the values obtained. The base excess, which is sometimes considered as the base deficit when the value is negative, indicates the deviation of the buffer base from normal. This derived value is often supplied in routine assessment of acid-base balance and is generally taken as an indication of the deviation of bicarbonate from normal. In an animal with a metabolic acidosis, the calculated base deficit provides a means of estimating the amount of bicarbonate required to correct acid-base balance to normal. This estimate is calculated by multiplying the base deficit by the probable bicarbonate space (which is variably estimated from 0.25 to 0.55 l/kg body weight). In newborn animals, the bicarbonate may be even higher, 0.40 to 0.65 l/kg body weight. The usual figure used is 0.3 to 0.4 l/kg. For a 20-kg animal with a base deficit of 10 mEq/l (10 mmol/l), the bicarbonate required would be calculated as follows: This calculation provides only a crude guide to bicarbonate requirements, but it can be a useful step in the quantitative approach for correcting a serious primary metabolic acidosis. Peter Stewart (Stewart 1981, 1983) was the first to describe a quantitative physiochemical approach to acid-base balance. In this approach, the acid-base status of the aqueous solutions of the body is determined not only by the Henderson-Hasselbalch equation but also by a series of seven other relationships, all of which could be represented by equations which must be satisfied simultaneously. Acid-base balance is determined by three independent variables: (1) strong ion difference [SID], (2) the partial pressure of CO2, and (3) the total concentration of nonvolatile weak acids [Atot], the principal component of which is the plasma proteins but also includes inorganic phosphate. Bicarbonate and hydrogen ion concentration, and thus pH, are dependent variables determined by the independent variables listed here. The appeal of Stewart’s approach is the focus on factors that are causally related to acid-base balance, the independent variables. The interested reader is referred to Stewart’s original work. A number of authors have attempted to adapt Stewart’s approach for practical application in human and veterinary medicine (de Morais, 1992b; Fencl and Leith, 1993; Fencl and Rossing, 1989; Frischmeyer and Moon 1994; Gilfix et al., 1993; Jones, 1990; Kowalchuk and Scheuermann, 1994; Whitehair et al., 1995). Many of these early papers directed to animal species used the human values for Atot and Ka, which may not be appropriate. Species-specific data are now available. In a landmark paper, Peter Constable (1997) refined Stewart’s model and developed an approach that he called the simplified strong ion model of acid-base equilibrium. The simplified strong ion model was developed from the assumption that plasma ions act as strong ions, volatile buffer ions ( Strong electrolytes are completely dissociated in aqueous solution and chemically nonreactive. The [SID] is simply the difference between the total concentration of strong cations (sodium, potassium, and magnesium) and the total concentration of strong anions (chloride, sulfate, lactate, acetoacetate, and 3-OH-hydroxybutyrate). Because they are present in higher concentrations in the body fluids, sodium, potassium, and chloride are normally the principal determinants of [SID]. The [SID] is synonymous with buffer base as described by Singer and Hastings (1948) and, as such, can be considered as roughly equivalent to the metabolic component of the traditional approach to acid-base balance. In fluids such as the CSF, which are normally devoid of protein, bicarbonate concentration is the same as the [SID]. Abnormalities in pCO2 are viewed in essentially the same manner in both the traditional and nontraditional approach to acid-base balance as described earlier. The contribution of plasma proteins to acid-base balance is not considered in the traditional approach to acid-base balance. The plasma proteins, or, perhaps more correctly, plasma albumin, make up the majority of [Atot], whereas inorganic phosphate normally accounts for less than 5% of [Atot]. The [Atot] in body fluids exists in both dissociated [A–] and undissociated [HA] forms. A decrease in [Atot] because of hypoalbuminemia causes an alkalosis with an increase in bicarbonate, whereas hyperalbuminemia has the opposite effect. Hypoalbuminemia is one of the most common causes of alkalosis in older human patients (McAuliffe et al., 1986). Changes in A– associated with changes in albumin concentration also have a direct and frequently overlooked effect on anion gap. Increases in A– result in an increase in anion gap, whereas decreases in A– cause a decrease in anion gap (McAuliffe et al., 1986). Change in protein concentration may potentiate or ameliorate the effects of alterations in SID on acid-base balance. As an example, in a vomiting dog, the elevated plasma protein associated with dehydration may reduce the bicarbonate increase anticipated for a given change in chloride concentration and SID. Protein and inorganic phosphate remain within the normal range in many clinical situations, and acid-base balance is then largely controlled by changes in pCO2 mediated by the respiratory system, whereas changes in [SID] are largely under the control of the kidneys. Renal compensation for primary respiratory disorders and respiratory compensation for primary metabolic acid-base disturbances are thought to be similar in both the traditional and nontraditional approach. Precise quantification of the anticipated compensating responses to primary acid-base disturbances based on change in SID has not yet been determined. Calculation of SID is simple and provides useful insight in patients with metabolic acid-base disturbances. Factors that influence SID range from changes in free water, to sodium-chloride imbalances that result from excessive losses or disproportionate retention of sodium or chloride, to the accumulation of strong organic anions. Organic acidosis can be produced by the accumulation of exogenous as well as endogenous organic anions. Examples of exogenous anions include salicylate, glycolate and formate associated with the ingestion of aspirin, ethylene glycol, and methanol, respectively. Many of these endogenous and exogenous organic anions are not routinely monitored in the diagnostic laboratory. This situation can create problems when calculating the SID because the presence of these unmeasured strong anions may not be appreciated. Although the anion gap can be helpful, it does not always accurately predict the presence of these compounds. More sophisticated mathematical methods have been suggested as a means for the detection of unmeasured anions the strong ion gap (Constable et al., 1998; Stewart, 1981) and, more recently, the simplified strong ion gap (Constable and [192]Stampfli, 2005). In animals with major changes in protein or albumin concentration, the primary concern must be a thorough investigation of the cause of the increase or decrease in protein. The acid-base consequences of change in protein and albumin concentration tend to be modest but are a potential source of confusion when evaluating acid-base data. The traditional approach and the nontraditional or strong ion approach to acid-base balance each has its supporters, and discussion over the benefits and limitations of either approach has at times been strident. However, this need not be an either-or situation. Both approaches have proven useful to address practical problems in both research and medical settings. The traditional approach based on the Henderson-Hasselbalch equation is simpler, more user friendly, and more widely accepted. Bicarbonate concentration estimates the severity of the acid-base disorder, but the strong ion approach may provide a better understanding as to why the bicarbonate is changing because it integrates acid-base and electrolyte disorders (de Morais, 2005). The strong ion approach has been gaining acceptance from the critical care community, which finds it useful in the analysis of the complex fluid, electrolyte, and acid-base problems presented to intensive care units. A number of computer programs adaptable to hand-held electronic devices have been developed and take some of the mathematical fear out of using the strong ion approach. Dietary factors, particularly the dietary cation-anion balance (DCAB), have been extensively studied in cattle, swine, poultry, and horses. The calculation of DCAB of a dry feed ration is remarkably similar to the calculation SID of body fluids. The DCAB in mEq is generally represented as (sodium + potassium) – (chloride + sulfate) per kg of dry matter of the diet. Diets with a high DCAB, such as alfalfa hay, have an alkalinizing effect and are an important factor in the alkaline urine of most herbivores. High grain rations tend to have a lower DCAB. Manipulation of the DCAB has been employed to enhance milk yield in dairy cattle, to reduce the incidence and severity of gastric ulceration in swine, to decrease the incidence of milk fever in cattle, and to alter the urine pH and calcium balance in horses. The addition of sodium bicarbonate to the ration of lactating dairy cattle to raise the DCAB from –100 to + 200 mEq/kg diet DM resulted in an increase of milk production of over 8%, which was due, in part, to more effective ruminal digestion (Block, 1984). On the other hand, supplementation of the diet of late-term cattle with calcium chloride or ammonium chloride so as to lower the DCAB and have an acidifying effect has been shown to reduce the incidence of milk fever by enhancing the mobilization of calcium from the bone (Block, 1984). As the application of these dietary practices becomes more widespread, it is essential that we appreciate the implications of dietary factors and electrolyte supplementation on mineral metabolism and acid-base balance (Fredeen et al., 1988). Sodium bicarbonate supplementation has been used as a prerace ergogenic aid in racehorses. Relatively large doses, 500 g or more of sodium bicarbonate, often mixed with sugar and water and referred to as “milk shakes,” have been given via nasogastric tube and result in a marked metabolic alkalosis. Although experimental studies have often failed to detect a measurable performance benefit from sodium bicarbonate supplementation, practical experience suggests that some horses, particularly standardbreds, show marked improvement in race times. Administration of any substance with intent to alter the performance is illegal in most racing jurisdictions. In many racing states, horses must meet specific guidelines for venous blood pH and bicarbonate or risk disqualification. It is important to understand the difference between volume regulation and osmoregulation. Osmoregulation is governed by osmoreceptors influencing ADH and thirst, whereas volume disturbances are sensed by multiple volume receptors that activate effectors such as aldosterone. Antidiuretic hormone increases water resorption (and therefore urine osmolality), but it does not affect sodium transport directly. Aldosterone enhances sodium reabsorption but not directly that of water. Thus, osmoregulation is achieved by changes in water balance and volume regulation mostly by changes in sodium balance. Water balance is achieved when water intake from all sources is equal to water output by all routes. Water is available as drinking water, as water content of feedstuffs, and as metabolic water derived by oxidative metabolism. Oxidation of 1g of fat, carbohydrate, or protein results in the production of 1.07, 0.06, or 0.41g of water, respectively. Water is normally lost from the body by four basic routes: urine, feces, insensible loss (respiratory and cutaneous evaporation), and sensible perspiration (sweat) in some animal species. Water intake and output may vary considerably from day to day, but normal animals are able to maintain water balance within remarkably narrow limits and, at the same time, maintain the critical interrelationship between water balance and electrolyte balance. For human subjects, there are well-established normal values for water intake and output via various routes. Although there is a substantial amount of data on water balance for many domestic animals (English, 1966a; Fonnesbeck, 1968; Hinton, 1978; Kamal et al., 1972; Leitch and Thomson, 1944; Sufit et al., 1985; Tasker, 1967a; Yoshida et al., 1967), these data vary markedly from species to species and are valid only for the specific experimental conditions under which they were collected. Animals eat to meet their caloric requirements. The nursing or grazing animal may have a feed intake that is greater than 90% water as compared to animals on dry hay or dried prepared pet food, which may contain less than 10% water. Some desert rodents are so well adapted that they are able to maintain water balance without water intake and rely on the water content of feedstuffs and metabolic water derived from oxidative metabolism. The koala in its native state in Australia obtains virtually all its water from the leaves of specific species of eucalyptus trees, which constitute its entire diet. Dehydration because of water restriction with and without heat stress has been studied widely in a variety of animal species (Bianca et al., 1965; Brobst and Bayly, 1982; Carlson et al., 1979a; Elkinton and Taffel, 1942; Genetzky et al., 1987; Hix et al., 1953; Kamal et al., 1972; Rumbaugh et al., 1982; Rumsey and Bond, 1976; Schultze et al., 1972; Tasker, 1967b). Quantitative assessment of the compartmental distribution of TBW between the ECF and the ICF has largely been based on the volume dilution of certain compounds or isotopic tracers. These studies require steady-state conditions and take a substantial period of time; as such, they are not well suited to the dynamic and often rapidly changing fluid balance characteristic of many clinical situations. Bioelectrical impedance analysis (BIA) may prove a useful tool in this regard. Bioelectrical impedance has been used for the assessment of body fat and fluid balance in humans and many animal species. Multifrequency bioelectrical impedance analysis has been successfully employed to monitor the rapid fluid changes in small animal patients undergoing dialysis. Algorithms for multifrequency BIA have been developed for use in the horse (Fielding et al., 2004). FIGURE 17-2 Body fluid compartments in a 450-kg horse with a pure water loss of 301. Hypertonic fluid volume contraction is indicated by the increase in serum sodium concentration from 140 mEq/l (140 mmol/l) to 155 mEq/l (155 mmol/l). Fluid losses are shared by the extracellular fluid (ECF) volume and the intracellular fluid (ICF) volume. Dehydration is a relatively common problem in domestic animals. Dehydration results from inadequate fluid intake in the face of normal to increased fluid losses. When water losses occur with little or no electrolyte losses (i.e., panting or feed and water restriction), serum sodium concentration and osmolality increase. This situation is called a hypertonic dehydration and occurs when water losses exceed losses of the exchangeable cations sodium and potassium (Carlson, 1987). This imbalance between total body water and exchangeable cations is best characterized as a relative water deficit (Scribner, 1969). The effects of a pure water loss of 30 l in a 450-kg horse are illustrated in Figure 17-2. In this theoretical example, there has been a 10% loss of body water but no change in electrolyte balance. Plasma sodium concentration has increased from a normal of 140 mEq/l (140 mmol/l) to 155 mEq/l (155 mmol/l). Fluid losses are shared proportionately by the ICF and ECF, and few other clinical or clinicopathological abnormalities will be noted until the fluid losses become more severe. Hypernatremia is associated with contraction of the ICF volume and shrinkage of the cells. When water losses are associated with proportionate losses of exchangeable cations (i.e., 130 to 150 mEq [130 to 150 mmol] of sodium plus potassium per l of water lost), an isotonic fluid volume contraction develops. Despite what at times may be large water and electrolyte losses, plasma sodium concentration and osmolality remain unchanged. The ECF and ICF share the fluid losses in a distribution that reflects the relative losses of sodium and potassium as proportional of the initial content in their respective fluid compartments. In many instances, isotonic dehydration may occur in which sodium loss exceeds potassium loss. This type of dehydration is observed with heavy sweat loss in horses, with acute diarrhea in most species, and with inappropriate diuretic administration. The effects of an isotonic fluid loss of 30 l of water, 3800 mEq (3800 mmol) of sodium, and 400 mEq (400 mmol) of potassium in a 450-kg horse are illustrated in Figure 17-3. With this type of isotonic fluid loss, plasma sodium concentration remains within normal limits despite the development of large sodium deficits. These animals manifest clinical signs of inadequate circulating fluid volume reflecting the sodium deficit and the associated decrease in plasma and ECF volume as has been shown in human subjects (McCance, 1937, 1938). When a substantial portion of the water deficit is replaced by water consumption or free water administration in these animals, the serum sodium concentration and osmolality decline, and a hypotonic, hypovolemic dehydration can develop (Sufit et al., 1985). The hyponatremia noted in this circumstance is best considered as an indication of a relative water excess (Scribner, 1969). FIGURE 17-3 Body fluid compartments in a 450-kg horse with an isotonic fluid loss of 301 of water, 3800 mEq (3800 mmol) sodium, and 400 mEq (400 mmol) potassium. Serum sodium remains unchanged at 140 mEq/l (140 mmol/l) despite the development of the substantial sodium deficit. Fluid losses are borne primarily by the extracellular fluid (ECF) volume. FIGURE 17-4 Body fluid compartments in a 450-kg horse after administration and retention of 301 of water. Although no sodium or potassium losses have occurred, serum sodium concentration has declined from 140 mEq/l (140 mmol/l) to 127 mEq/l (127 mmol/l) reflecting a relative water excess. Water retention results in proportionate expansion of extracellular fluid (ECF) volume and intracellular fluid (ICF) volume. The effects of the administration and retention of 30 l of water in a 450-kg horse are illustrated in Figure 17-4. In this example, total body water has been increased by 30 l, and there is an absolute as well as relative water excess. The primary effect of this water load is to dilute the electrolytes in the body fluids producing a substantial decline in plasma sodium concentration and osmolality. These changes occur despite the fact that there has been no change in sodium or potassium balance. In this example, the ECF and ICF share the water excess proportionately. The hyponatremia is associated with expansion of ICF and thus swelling of the cells. Overhydration rarely occurs in normal individuals. The large water load described in Figure 17-4, if administered to a normal animal, would produce only transient changes, and the excess water would be eliminated by renal excretion. Even animals with psychogenic polydipsia ordinarily are able to maintain normal water balance through appropriate renal water excretion unless sodium depletion and renal medullary washout occur. However, overhydration can occur iatrogenically as the result of excessive fluid administration to patients with compromised renal function. If these fluids provide free water, as with 5% dextrose, plasma sodium concentration will decrease reflecting the change in relative water balance. If these fluids consist of isotonic sodium-containing replacement fluids such as saline or lactated Ringer’s solution, there will be little or no change in plasma sodium concentration (Carlson and Rumbaugh, 1983; Cornelius et al., 1978), but there will be an increase in plasma and ECF volume with the potential for cardiovascular overload, pulmonary edema, or generalized edema formation. In this instance, the primary problem is sodium retention, and the changes in water balance are secondary. The ECF volume contains approximately one-half to one-third of the body’s sodium. Most of the remaining sodium is bound in skeletal bone, relatively little of which is rapidly exchangeable (McKeown, 1986). The ECF volume thus contains essentially all of the body’s readily available and exchangeable sodium. The exchangeable sodium content is the principal determinant of ECF volume, and sodium deficits are the principal causes of decreased ECF volume (Saxton and Seldin, 1986). Increases in sodium content result in expansion of ECF volume, which may lead to the development of hypertension or edema formation (Dow et al., 1987b; McKeown, 1986). In either instance, the observed plasma sodium concentration will primarily depend on relative water balance. Because monitoring daily electrolyte balance is difficult in most animal species, urinary fractional excretion or creatinine clearance ratios have been useful to provide an index of daily intake or potential deficits of sodium, potassium, chloride, and other electrolytes. Normal values have been established for dogs, cats, horses (Morris et al., 1984), and cattle (Fleming et al., 1992). Sodium depletion is rarely the result of dietary sodium deficiency (Aitken, 1976). This is true even for herbivores whose feedstuffs are normally very low in sodium. Chronic sodium depletion has been reported in lactating dairy cows on a low salt diet (Whitlock et al., 1975a), but the sodium deficit was most probably the result of sodium losses in milk (Michell, 1985). Mastitis markedly enhances sodium loss in milk and could play a role in sodium depletion in lactating cows maintained on a low-salt diet (Michell, 1985). Sodium depletion almost invariably is associated with excessive losses of sodium-containing fluid (McKeown, 1986; Rose, 1984), most often occurring as the result of gastrointestinal losses through vomiting or diarrhea (Fisher and Martinez, 1976; Lakritz et al., 1992). Excessive renal sodium losses can occur with intrinsic renal disease or as the result of diuretic therapy (Rose, 1984; Rose and Carter, 1979; Rose et al., 1986). Cutaneous losses via sweating are important in the exercising horse and may occur in any animal with extensive exfoliative dermatitis or burns. Salivary losses as the result of esophagostomy have been reported as a cause of sodium depletion in horses (Stick et al., 1981). A history suggestive of excessive loss of sodium-containing fluid is thus an extremely important criterion for the diagnosis of sodium depletion in domestic animals. The foregoing discussion applies indirectly to the so-called third space problems associated with a sequestration or compartmentalization of a portion of the ECF volume (Rose, 1984). This situation can occur with obstructive bowel disease or with the sudden accumulation of fluid within the abdomen or thorax resulting from peritonitis, ruptured bladder, ascites, or pleural effusion. These fluids have an electrolyte composition similar to the ECF and initially are drawn from the plasma volume and interstitial fluids. The resultant change in plasma volume and effective circulating fluid volume produce the same clinical and clinicopathological changes as those observed with excessive external losses of sodium containing fluid (Billig and Jordan, 1969). In these cases, fluids have not been lost from the body, but there has been an internal sequestration of fluid which can be mobilized if treatment is effective. Sodium excess occurs most often in association with an increase in body water leading to an isotonic expansion of ECF volume and the development of hypertension or generalized edema (Saxton and Seldin, 1986). Congestive heart failure, hypoalbuminemia, and hepatic fibrosis may lead to a failure to maintain effective circulating volume, which then, in turn, results in compensating renal sodium retention (Rose, 1984). In these cases, expanded ECF volume represents an attempt to restore effective circulating fluid volume, and, at least initially, plasma volume may decrease whereas ECF volume expands. An expansion of ECF volume of 20% to 30% may be necessary before edema is first evident (Scribner, 1969). Sodium excess and edema can develop iatrogenically as the result of the excessive administration of sodium-containing fluid to patients with severely compromised renal function. Most domestic animals can tolerate a large sodium intake provided they have adequate drinking water (Aitken, 1976; Buck et al., 1976; Pierce, 1957). Excessive salt intake may occur when animals that had been on salt-restricted diets are first allowed free access to salt (Michell, 1985). Salt intoxication can occur in cattle that are feeding in reclaimed salt water marshes or on pastures contaminated by oil field wastes when they are deprived of fresh water (Aitken, 1976; McCoy and Edwards, 1979; Michell, 1985; Pierce, 1957; Sandals, 1978). Salt poisoning in swine also occurs in association with water restriction followed by access to water (Aitken, 1976; Buck et al., 1976). Salt intoxication is generally associated with increases in plasma or cerebrospinal fluid sodium concentrations. Neurological signs associated with excessive salt consumption coupled with water restriction are related to development of cortical edema and, in swine, a characteristic eosinophilic meningoencephalitis (Aitken, 1976). Potassium is largely an intracellular ion with over 98% of the exchangeable potassium located intracellularly (Brobst, 1986; Strombeck, 1979). This distribution of potassium is coupled with the active extrusion of sodium from the cells, which is maintained by an energy-dependent sodium:potassium pump at the cell membrane (Brobst, 1986; Tannen, 1986). Potassium distribution across the cell membrane plays a critical role in the maintenance of cardiac and neuromuscular excitability. Changes in potassium concentration that alter the ratio of intracellular to extracellular potassium alter membrane potential (Tannen, 1986). In general, hypokalemia increases membrane potential, producing a hyperpolarization block resulting in weakness or paralysis, whereas hyperkalemia decreases membrane potential causing hyperexcitability (Patrick, 1977). These features depend on the state of total body potassium content but also depend on the speed with which hypokalemia or hyperkalemia develops (Saxton and Seldin, 1986). As an example, acute potassium depletion may result in hypokalemia before the development of marked change in intracellular potassium, producing a substantial alteration in the ratio of intracellular-to-extracellular potassium. Acute hypokalemia can produce a much greater alteration in membrane potential and thus more marked clinical signs than a gradually developing hypokalemia of the same magnitude (Saxton and Seldin, 1986). Potassium homeostasis involves regulation of internal balance (i.e., the distribution of potassium between the ECF and ICF) as well as external balance (i.e., the relation of potassium input to output) (Brobst, 1986; Rose, 1984). Internal potassium balance is influenced by changes in acid-base status, glucose and insulin administration, exercise, and catecholamine release and will be discussed in greater detail in the section on factors that influence plasma potassium concentration. Potassium depletion is the result of altered external balance in which potassium losses by all routes exceed intake. Potassium is present in relatively high concentrations in most animal feeds. Therefore, dietary deficiency alone is not a common cause for potassium depletion (Tasker, 1980). However, dietary factors were associated with hypokalemia in hospitalized cats, particularly when associated with diseases linked to increased potassium loss (Dow et al., 1987a). Herbivores, such as the horse, fed an all-hay diet may have a daily potassium intake of 3000 to 4000 mEq (3000 to 4000 mmol) per day (Hintz and Schryver, 1976; Tasker, 1967a). Most of this potassium is absorbed in the small intestine and colon with subsequent renal excretion of more than 90% of the daily potassium intake (Hintz and Schryver, 1976). These animals are thus highly adept at excretion of a large daily potassium intake. However, renal compensation for deficient potassium intake is not efficient, and renal conservation of potassium may be delayed for several days when animals normally fed a high-potassium diet are suddenly taken off feed or develop anorexia (Tasker, 1967b). Potassium depletion most commonly develops as the result of gastrointestinal losses from vomiting or diarrhea. Excessive renal losses can occur as the result of diuretic usage, mineralocorticoid excess, renal tubular acidosis, and in the diuresis state that follows relief of urinary obstruction (Saxton and Seldin, 1986). Potassium depletion may result in decreased ICF volume, altered membrane potential, altered intracellular pH, and alterations of potassium-dependent enzymatically mediated reactions (Elkinton and Winkler, 1944). Clinical features include muscle weakness, ileus, cardiac arrhythmias, rhabdomyolysis, and renal dysfunction (Dow et al., 1987a, 1987b; Earley and Daugharty, 1969; Tannen, 1986). Potassium excess occurs relatively rarely and is generally a consequence of some alteration of renal excretion of potassium. Potassium excess may be associated with Addison’s disease, some forms of renal disease, and clinical situations associated with hypovolemia and renal shutdown (Rose, 1984; Weldon et al., 1992). Care should be taken to avoid the rapid administration of large amounts of potassium salts orally or intravenously to patients with severely compromised renal function. Even normal animals can develop significant electrocardiographic abnormalities with potassium (Dhein and Wardrop, 1995; Epstein, 1984; Glazier et al., 1982) or calcium infusions (Glazier et al., 1979). Modest changes in hydration tend to produce roughly proportional changes in plasma sodium and chloride relative to sodium concentration. Acid-base alterations are associated with disproportionate changes in plasma chloride concentration (Saxton and Seldin, 1986). A disproportionate hyperchloremia is seen in association with a low to normal anion gap metabolic acidosis. Chloride concentration increases in this type of acidosis as the result of proportionately smaller losses of chloride than bicarbonate and enhanced renal chloride resorption in response to decreased bicarbonate (Saxton and Seldin, 1986). Disproportionate hypochloremia is a consistent feature of metabolic alkalosis (Rose, 1984). Chloride depletion develops in these animals as a result of excessive loss or sequestration of fluids with high chloride content. Changes in water balance can result in modest alterations in the relative concentrations of plasma sodium and chloride. A pure water deficit produces increased sodium concentration, which exceeds the increases in chloride. This contributes to the development of a contraction alkalosis with an increase in bicarbonate. A pure water excess has the opposite effect and can result in an expansion acidosis. It is convenient to discuss the theoretical ramifications of pure water loss or specific electrolyte deficits. However, in practical clinical situations, the issue is almost never so clearly defined, and most often, there is a combination of fluid, electrolyte, and acid-base alterations. Many medical problems result in a consistent pattern of fluid and electrolyte loss with predictable changes in fluid volume, electrolyte concentration, and acid-base balance. A clear understanding of the interrelationships between specific deficits and their clinicopathological consequences is essential if an appropriate initial diagnosis is to be made. Once treatment has been initiated, all clinical and clinicopathological data will be influenced not only by the primary medical problem and compensating responses but also by the effects of chemotherapeutic agents and fluid therapy as well. Therapeutic intervention may not always be appropriate, organ function may be impaired, and thus the anticipated clinicopathological responses become less predictable. These situations represent the bulk of clinical case material and laboratory data evaluated by clinicians and clinical pathologists. Under these circumstances, it is essential to understand the basic mechanisms that underlie changes in clinicopathological data and how these changes relate to specific imbalances. Rational fluid therapy depends on accurate evaluation of the fluid and electrolyte deficits, the associated acid-base alterations, and the primary disease processes that underlie these imbalances. Evaluation must include an accurate history, a complete physical examination, and, of course, laboratory evaluation of appropriate parameters. An accurate history is absolutely essential for the evaluation and management of the patient with fluid and electrolyte imbalances. Basic signalment factors of age, sex, breed, pregnancy, and stage of lactation are important because these factors influence the incidence and severity of many disorders. The presence of a preexisting or coexisting disease process and an accurate drug history can be exceedingly important not only in the evaluation of fluid and electrolyte disorders but also in fluid selection and patient management. Of particular importance is the history of prior renal disease, diuretic usage, or exposure to potentially nephrotoxic drugs. Status of feed and water intake is exceedingly useful. Most animals that continue to eat and drink normally are able to maintain fluid balance even in the face of excessive fluid losses. However, reduced or restricted fluid intake in the face of normal to enhanced fluid losses can quickly result in dehydration. Inadequate fluid intake may result from neurological disorders or traumatic injuries to the head or neck, whereas painful or obstructive lesions in the mouth, pharynx, or gastrointestinal tract may restrict feed and water intake. Inadequate water intake is often the result of management errors, broken or frozen water lines, and other factors. A history of polydipsia suggests that excessive fluid losses have occurred. Vomiting and diarrhea are obvious causes of fluid and electrolyte loss, but these findings also reflect gastrointestinal disorders, which may contribute to inadequate fluid and electrolyte intake or absorption. Excessive fluid losses may be associated with vomiting, diarrhea, polyuria, excessive salivation, copious drainage from cutaneous wounds or burns, and as the result of heavy sweat losses in exercising horses. The water losses that occur in these situations are generally associated with significant sodium depletion and subsequent decreases in the effective circulating fluid volume. Vomiting in small animals (Clark, 1980), gastrointestinal stasis in ruminants (Gingerich and Murdick, 1975b), and excessive sweat losses in endurance horses (Carlson, 1983b) are associated with large losses or compartmentalization of chloride-rich fluids, which contribute to the metabolic alkalosis that frequently accompanies these disorders. Dehydration is defined as a loss of body water. Clinical signs of dehydration are said to be first apparent with fluid losses equivalent to 4% to 6% of body weight. Moderate dehydration is said to be present with fluid losses of 8% to 10%, and severe dehydration is present when fluid losses exceed 12% of body weight. It is important to realize that although these guidelines have been clinically useful, there is relatively little documentation of this precise quantitative relationship in most animal species. Accurate measurement of fluid intake from all sources and output by all routes is not possible in most clinical situations. In acute situations, changes in body weight provide the most accurate guide to change in net water balance. Repeated measurement of body weight is a key component of the monitoring of patients on fluid therapy. The clinical signs of dehydration include weight loss, altered skin turgor, sunken eyes, and dry mucous membranes. If control of renal function is normal, urine volume is generally markedly reduced. The clinical consequences of dehydration depend much more on the pattern of electrolyte loss than the absolute water deficit. Clinical signs associated with acute sodium deficits are largely related to hypovolemia and decreases in the effective circulating volume. These signs include increased pulse rate, decreased pulse pressure, delayed jugular distensibility, increased capillary refill time, and decreased blood pressure. Urine output is generally decreased and urine sodium and chloride concentrations are normally reduced. Decreases in ECF volume are always reflected by decreases in plasma volume, but the reverse is not always true. In the absence of blood or protein loss, the PCV and TPP concentrations increase, reflecting the decrease in plasma and ECF volume as will be discussed more fully in Section VIII.A. If it is assumed that the quantity of plasma proteins within the plasma volume remains constant (this assumption is not always correct), it is possible to calculate the percentage change in plasma volume based on protein concentration (Boyd, 1981): where PV indicates plasma volume, PP1 indicates initial plasma protein concentration, and PP2 indicates final plasma protein concentration. It is also possible to calculate the change in plasma volume based on the change in PCV provided it is assumed that the number of erythrocytes within the circulating blood volume and the mean corpuscular volume remain constant (Boyd, 1981): where PV indicates plasma volume, PCV1 indicates the initial PCV, and PCV2 indicates the final PCV. Using this approach, the calculated increase of PCV and plasma protein concentration associated with a progressive reduction in plasma volume in a 20-kg dog is presented in Figure 17-5. In this example, the dog was assumed to have had an initial plasma volume of 5% of body weight (0.05 l/kg), an initial PCV of 40% (0.4 l/l), and an initial TPP concentration of 6.5 g/dl (65 g/l). A 50% reduction in plasma volume results in 100% increase in TPP from 6.5 to 13.0 g/dl, whereas PCV increased only from 40 to 57, a relative increase of just over 40%. Note that as plasma volume declines, the percentage change in PCV is always less than the percentage change in plasma protein concentration. This statement is true whether the changes are due to an increase or decrease in plasma volume. FIGURE 17-5 Effects of decreasing plasma volume on PCV and plasma protein concentration as calculated using Eqs. 17–13 and 17–14. In most clinical situations, the initial PCV and plasma protein concentration are not known, and to make these estimates, it must be assumed that these values were within the normal range before dehydration. A large disparity in the change in PCV and TPP concentrations in the face of a history of loss of sodium-containing fluid and clinical evidence of reduced effective circulating volume suggests either erythrocytes or protein has been lost from the circulation. Marked increases in PCV with normal to low plasma proteins are frequently encountered in animals with acute toxic enteritis because of losses of protein into the gastrointestinal tract (Merritt et al., 1977). This finding suggests erosive bowel disease but also could be seen in animals with low protein as a result of preexisting renal or hepatic disease. Increases in plasma protein with little or no change in PCV are seldom seen in dehydrated animals but could occur with a preexisting anemia or hyperproteinemia. Blood loss generally results in a decrease in both PCV and TPP concentration. Sodium deficits result in decreases in ECF volume, which are usually reflected by changes in plasma volume (Hopper et al., 1944; Saxton and Seldin, 1986). As plasma volume decreases, the PCV and TPP concentrations increase. Changes in PCV and TPP are thus particularly useful in evaluating the need for and the response to sodium-containing replacement fluid (Carlson, 1983a). When sodium-containing fluids are administered to dehydrated volume-depleted subjects, plasma volume reexpansion is reflected by the return of PCV and TPP toward the normal range (Hayter et al., 1962). Declining PCV and TPP in response to fluid therapy are the two most useful laboratory indicators of return of effective circulating fluid volume and should be correlated with clinical evidence such as return of normal pulse pressure, capillary refill time, and jugular distensibility. TABLE 17-4 Reference Values for Blood Gas and Electrolyte Determinationsa aIn part from Tasker (1980).
I. INTRODUCTION
II. PHYSIOLOGY OF FLUID AND ELECTROLYTE BALANCE
III. BODY FLUID COMPARTMENTS
A. Total Body Water
and
the actual valence and milliequivalents must be estimated. The same variability is true of protein anions as well.
B. Extracellular Fluid Volume
C. Intracellular Fluid Volume
IV. REGULATION OF BODY FLUIDS AND ELECTROLYTES
A. Effective Circulating Volume
B. Antidiuretic Hormone
C. Renin-Angiotensin
D. Aldosterone
E. Atrial Natriuretic Factor
V. PHYSIOLOGY OF ACID-BASE BALANCE
A. Definition of pH
B. Buffers
) buffer pairs as well as the plasma proteins. The intracellular buffers include protein; organic and inorganic phosphates; and in the red cell, hemoglobin. Cation exchange involving the intracellular movement of hydrogen ion in exchange for potassium and (to a much lesser extent) sodium is an additional and important means whereby cellular mechanisms buffer an acid load. The carbonate in bone provides a large and often overlooked buffer store. Although difficult to accurately measure, it has been estimated that bone carbonate may contribute up to 40% of the buffering capacity of an acute acid load. It is important to remember that a change in hydrogen ion concentration will affect all the body’s buffer pairs. Thus, evaluation of any one buffer pair reflects the changes that occur in all of the buffer pairs.
1. Bicarbonate/Carbonic Acid Buffer System
with little change in the extracellular pH. These processes are reversed in the alveoli. As HHb is oxygenated, H+ is released and combines with
to form H2CO3, which dissociates to CO2 and H2O. Carbon dioxide then is excreted by alveolar ventilation.
/H2CO3 buffer pair.
C. Acidosis
1. Metabolic
2. Respiratory
D. Alkalosis
1. Metabolic Alkalosis
2. Respiratory Alkalosis
E. Mixed Acid-Base Imbalances
F. Anion Gap
Decreased anion gap:
Increased cationic protein
Polyclonal gammopathy (IgG)
Hypoalbuminemia
Hyperchloremic acidosis
Altered protein anionic equivalents
Laboratory error
Increased anion gap:
Metabolic acidosis
Organic acids (lactic, keto acids)
Hypovolemic shock
Anaerobic exercise
Diabetes
Grain overload
Ketosis
Nonmetabolizable acids
Inorganic acids (sulfate, phosphate)
Uremic acidosis
Intoxication or poisoning
Salicylate
Paraldehyde
Metaldehyde
Methanol
Ethylene glycol
Laboratory error
G. Bicarbonate and Total CO2
H. Buffer Base, Standard Bicarbonate, and Base Excess or Base Deficit
I. Nontraditional or Strong Ion Approach to Acid-Base Balance
), or nonvolatile buffer ions. Plasma pH is determined by five independent variables: pCO2, strong ion difference, concentration of individual nonvolatile plasma buffers (albumin, globulin, and phosphate), ionic strength, and temperature. The simplified strong ion model conveys, on a fundamental level, the mechanism for change in acid-base status, explains many of the anomalies when the Henderson-Hasselbalch equation is applied to plasma, and is conceptually and algebraically simpler than Stewart’s strong ion model. The model has provided an in vitro method for determination of species-specific values for [Atot] and Ka, which has been applied to the plasma of horses, dogs, cattle, pigeons, and humans (Constable, 1997; Constable and [192]Stampfli, 2005; Stampfli et al., 1999, 2006; Stampfli and Constable, 2003).
J. Dietary Factors in Acid-Base Balance
VI. EVALUATION OF IMBALANCES
A. Water
1. Depletion-Dehydration
2. Water Excess-Overhydration
B. Sodium
1. Sodium Depletion
2. Sodium Excess
C. Potassium
1. Potassium Depletion
2. Potassium Excess
D. Chloride
VII. CLINICAL FEATURES OF FLUID AND ELECTROLYTE BALANCE
A. History
B. Clinical Signs
VIII. CLINICOPATHOLOGICAL INDICATORS OF FLUID AND ELECTROLYTE IMBALANCE
A. Packed Cell Volume and Total Plasma Protein
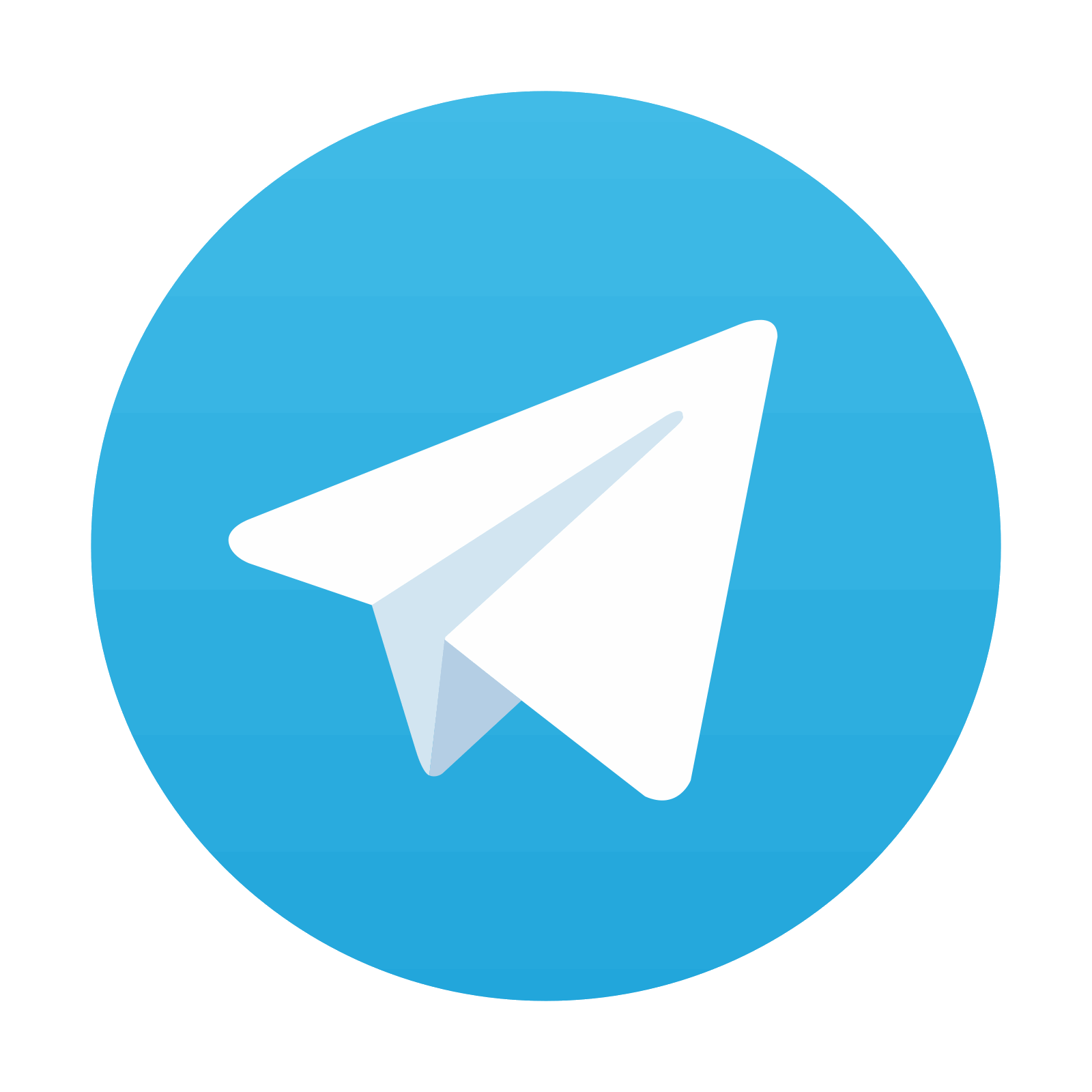
Stay updated, free articles. Join our Telegram channel
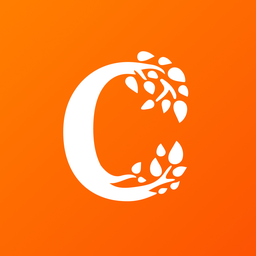
Full access? Get Clinical Tree
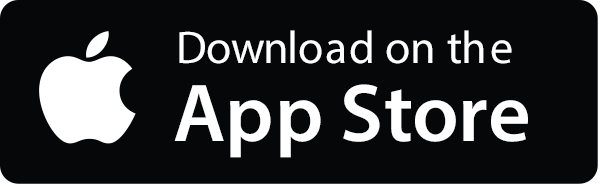
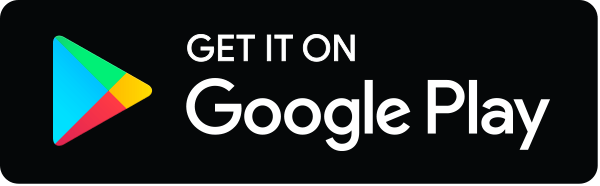