Exercise Physiology of the Canine Athlete
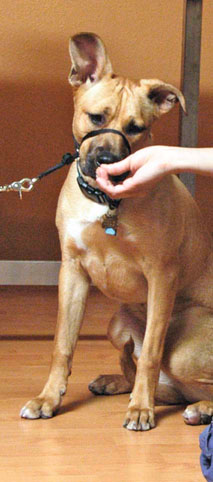
Exercise physiology is the study of the effects of exercise and training on normal functions of the body. When implementing a rehabilitative exercise or a conditioning program it is important to have a thorough understanding of normal and abnormal physiologic functions of the body. Sufficient knowledge of physiologic functions of the body increases understanding of the body’s response to exercise and allows one to maximize the effectiveness of a rehabilitative program. Exercise has many profound effects on the musculoskeletal system. There is a vast amount of coordination and integration of individual body systems, and, although exercise programs and our rehabilitative efforts mainly focus on improving the function of the musculoskeletal system, it is important to understand the response of other major body systems to the influence of exercise. Physical rehabilitation is typically used to treat or condition areas of the body that are injured or are suffering from abnormal function. Because there may be limitations during healing of an injury, one should understand the body’s limitations during injury as well as compensatory methods used by the body. A good general understanding of physiology is very beneficial to the clinician performing rehabilitation, in designing a rehabilitation program and understanding how exercise and training affect the body.
Skeletal Muscle
Muscle Physiology
Skeletal muscle plays a large role in exercise and determining athletic performance, and it is important to understand its structure and function. The muscular system is a collection of contractile units that provide the forces for various functions to take place such as locomotion, posture, respiration, and circulation. The three types of muscle in the body are skeletal, smooth, and cardiac muscle. This section focuses on skeletal muscle physiology.
The functional unit of skeletal muscle is the muscle fiber, or myofiber (Figure 9-1). Most skeletal muscles contain muscle fibers that span the entire length of the muscle, cross over at least one joint, and are typically innervated by only one alpha motor neuron. The sarcolemma is the cell membrane that surrounds each muscle fiber. Each muscle fiber contains several hundred to several thousand myofibrils. Myofibrils are composed of contractile protein filaments called myosin (thick filaments) and actin (thin filaments). Myofibrils are divided into segments called sarcomeres, each of which lies between two Z discs (filamentous protein different from actin and myosin). From each Z disc actin filaments extend in both directions to interdigitate with myosin filaments.
Contraction of a muscle fiber is first initiated by an action potential, which starts at the central nervous system, travels down an alpha motoneuron to its own axon and the neuromuscular junction. The action potential causes a large influx of calcium ions through calcium channels. The calcium influx results in the release of acetylcholine into the extracellular space between the motoneuron and the neuromuscular junction of the muscle fiber. The acetylcholine then activates nicotinic acetylcholine receptors, opening sodium-potassium channels, triggering an action potential. The action potential travels along a muscle fiber’s T-tubule network, depolarizing the muscle membrane. The action potential causes the sarcoplasmic reticulum to release large quantities of calcium into the cytoplasm. A protein called tropomyosin is associated with each actin filament. In a resting state tropomyosin covers the active sites on the actin filaments so that attraction between an actin and myosin filament cannot occur and cause contraction. Calcium released from the sarcoplasmic reticulum binds to a protein called troponin, which causes the tropomyosin to shift positions and uncover the binding site between actin and the cross bridge portion of myosin filaments. Prior to binding of actin and a myosin cross bridge, adenosine triphosphate (ATP) bound to myosin is cleaved; however, the adenosine diphosphate (ADP) and phosphate ion remain bound to the cross bridge. When the myosin head portion of the cross bridge bonds with the active site on the actin filament, a conformational change is created, causing a power stroke or pulling of the actin filament. The previously cleaved ATP supplies the energy for a power stroke. After the power stroke has been completed, the ADP and phosphate ion are released from the myosin head, allowing for binding of a new ATP molecule. The binding of a new ATP molecule causes detachment of the myosin head from the actin filament. The ATP is then cleaved to begin a new power stroke. This process is repeated again and again to contract a muscle.
Skeletal muscle cells are classified into types based on the specific individual contractile and metabolic properties of each cell. A general classification scheme divides muscle fibers based on their speed of contraction, which is a result of their contractile and metabolic properties. Generally speaking, muscle fibers are considered to be either slow twitch (type I) or fast twitch (type II). Type I fibers contain higher levels of oxidative enzymes, myoglobin, and mitochondria, and are well suited to perform aerobic activities and resist fatigue. Type II fibers have smaller amounts of oxidative enzymes and limited aerobic capabilities. However, type II fibers have large amounts of glycolytic enzymes for anaerobic metabolism and are capable of generating higher levels of tension and shortening velocity, but are much less resistant to fatigue than type I fibers.
Dogs are rather unique in that, along with the aerobic abilities of slow twitch fibers, their fast fibers also have significant oxidative capabilities. In fact, there is significant evidence that suggests an absence of purely glycolytic muscle fibers in the skeletal muscle of the trunk and limbs of dogs.1 It has been suggested that the only location of type IIB muscle fibers in dogs is in laryngeal muscles.1–3 It is believed that previous studies that relied on immunostaining alone have resulted in misclassification of muscle fibers. Newer studies have implemented electrophoresis, immunoblots, immunohistochemistry, and image analysis to characterize canine muscle fibers.1,3
Currently, five major fiber types are described in the trunk and limb muscles of dogs, which include three pure fibers (I, IIA, and IIX) and two hybrid fibers (I+IIA and IIAX).1,3 The oxidative/glycolytic ratio of canine muscle fibers decreases from type I (highest) to IIX (lowest). Type IIA is intermediate, with both hybrids between their respective pure isoforms.1 The decrease in oxidative potential from type I to type IIX is three to four times less than other mammals, which is another finding supporting the superior oxidative capacity of canine skeletal muscle. Even the least oxidative muscle fibers in dogs have a substantial capability for aerobic metabolism compared with fast fibers of other species. The maximum shortening velocity of canine muscle fibers increases from type I (slowest) to type IIX (fastest).3
Muscle Fatigue
Contraction of skeletal muscle at some rates required for exercise cannot continue indefinitely. When a muscle is unable to maintain power output during contraction, it has reached the point of fatigue. There are several explanations for the cause of fatigue. One controversial explanation is that fatigue can originate from the central nervous system (CNS), possibly because of psychological factors that can either enhance or limit performance. Dogs may serve as a good example for this theory because the personality of certain breeds of dogs might allow them to continue to exercise beyond the point that other breeds or species would continue. The drive to run or retrieve might allow some dogs to continue to participate in intense physical activity despite obvious exhaustion, simply as the result of verbal encouragement, whereas other breeds or species would not continue regardless of the stimulus. The evidence on CNS involvement in fatigue is unclear and unproven.
However, there are several changes at the cellular level of skeletal muscle that result in fatigue during exercise. Fatigue is mainly an imbalance between ATP requirements by muscle and the ability to produce ATP. During exercise, the need for ATP by skeletal muscle dramatically increases. As the components needed to regenerate ATP levels become depleted, there is less ATP available to provide energy for sustained muscle contraction, resulting in an inability to exercise or perform at the same level or rate as before. Phosphate is one of the components needed for formation of ATP. When ATP formation cannot keep up with ATP consumption, excessive inorganic phosphate ions accumulate in the muscle cell. High concentrations of phosphate ions can directly interfere with cross bridging of actin and myosin, as well as inhibit calcium release from the sarcoplasmic reticulum.4,5 Lactic acid (lactate) is an end product of glucose metabolism by way of the glycolytic pathway. Lactate is formed during conditions of inadequate oxygen supply to muscle or by muscle fibers with low numbers of mitochondria. Accumulation of lactic acid as a result of anaerobic metabolism within muscle cells can also inhibit enzymes involved in production of ATP.
Glycogen is an important energy source used during exercise. Depletion of muscle glycogen is a critical factor associated with fatigue in elite athletes during prolonged periods of exercise.6 Sled dogs are a good example of an elite endurance athlete. High-endurance canne athletes can have a remarkable ability to maintain muscle glycogen concentrations during periods of prolonged exercise. Despite limited carbohydrate intake, sled dogs running 160 km (99.4 miles) per day for 5 consecutive days did not develop cumulative muscle glycogen depletion.7,8 On the first day of exercise there was a decrease in muscle glycogen content; however, each of the subsequent periods of exercise resulted in stable muscle glycogen content, which was greater than the content after the first day. It has been determined that muscle glycogen preservation in high-endurance athletes, such as sled dogs, occurs as a result of attenuation of depletion rather than rapid replenishment.8 During the first portion of multiday endurance racing, sled dogs use a significant portion of muscle glycogen. On subsequent days they use only a small percentage of their remaining muscle glycogen stores. Rather than glycogen, sled dogs use other energy sources such as muscle triglycerides. Dogs are likely capable of this form of metabolism because of their innate ability to use free fatty acids, their ability to perform high levels of training in endurance activities, and the consumption of a diet with a large percentage of fat as one of its components. Canine skeletal muscle is composed mostly of type I, IIA, and IIAX muscle fibers, all of which are capable of high levels of oxidative metabolism.1 Highly aerobic mammals such as dogs have an increased capability to use fat-based substrates for energy metabolism.9 The high-fat, high-protein diet of sled dogs can help preserve muscle glycogen stores during exercise. Evaluation of fasted rats subjected to several bouts of repeated exercise showed similar results of muscle glycogen preservation, suggesting that metabolic adjustments allow certain species, such as dogs, to preserve muscle glycogen stores during bouts of repeated exercise. There may be a critical concentration of muscle glycogen that the body protects against excessive consumption during repeated exercise. Compared with humans, conditioned canine athletes may have an advantage regarding prolonged exercise based on their highly aerobic metabolism and ability to use alternative substrates for energy and maintenance of muscle glycogen stores.
Conditioning and Training of Skeletal Muscle
Conditioning of skeletal muscle involves performing physical exercise that prepares muscle for the specific task that it will be asked to perform. Training pertains to incorporating the specific exercise into the type of sporting event or activity the dog will be involved in. A portion of training also involves behavior modification required to perform the specific activity. During exercise training athletes are frequently pushed to perform an activity until physically unable to continue. This is commonly termed the overload principle, which refers to the need for a system to be exercised to a level beyond that which it is accustomed for training to have an effect. Placing the system in an overloaded state causes it to adapt to new conditions, thereby improving a system’s ability to perform a task at a higher level of performance. This principle applies to the cardiovascular system, the musculoskeletal system, and other body systems. Factors that influence overload include intensity, duration, and frequency of a particular exercise. Most types of exercise training can be divided into either endurance or strength training activities. It is important that training uses the systems and structures involved in the activity. This is a training principle called specificity. For example, a dog that competes in a sport that requires pulling loads would be better served to run while pulling against resistance as opposed to trotting for a long duration on a treadmill. In humans, engaging in training activities that target specific muscle groups results in adaptations in the muscle types targeted. For example, endurance-type exercises, such as running prolonged distances at a submaximal heart rate, increases the capacity to produce energy in an aerobic manner by increasing capillaries and mitochondria, especially in type I muscle fibers.10
High levels of endurance are very important in dogs that participate in prolonged periods of exercise such as sporting, herding, and long-distance racing dogs. Endurance relates to the ability of a muscle or group of muscles to undergo many repetitions of contraction under low loads. Endurance exercise usually targets specific muscle groups involved in the specific action and lasts for a prolonged time, usually greater than 15 minutes. Endurance exercises for dogs include trotting, running, swimming, land and underwater treadmill activity, and long-distance sled pulling. It was previously believed that endurance exercise does not result in an increase in type I muscle fibers. More recent research indicates that prolonged endurance exercise can promote a shift of type II muscle fibers to type I in humans.11 Other changes associated with long-term endurance training include an increase in capillary density and vascularity of muscle tissue, increasing the delivery of oxygen and nutrients to the muscle. The issue of muscle fiber type conversion after endurance exercise in humans remains somewhat controversial, but can be explained by the fact that muscle fibers are classified by staining them and as their aerobic capacities are altered, fibers that were borderline in classification can “shift” into another category.
Studies have evaluated skeletal muscle adaptation in dogs during periods of endurance training. One study of foxhounds undergoing endurance exercise failed to show the biochemical and histochemical adaptations in skeletal muscle that have been found in some studies involving humans.12 Foxhounds did not show alterations in the activity of succinate dehydrogenase (SDH), muscle fiber type, or capillary density of skeletal muscle after a 12-week endurance training program.12 These findings contrast with those of humans, who do undergo detectable skeletal muscle adaptations after endurance training. SDH is a measure of the oxidative capacity of a muscle. There was no difference in SDH activity, muscle fiber staining, or capillary density in trained and untrained foxhounds, suggesting that an untrained dog is already well suited to support the highly oxidative metabolism of endurance exercise, or that the training program employed in that study was not challenging enough to result in muscle fiber changes. The lack of a change in fiber type staining after endurance exercise may support the theory that dogs, such as foxhounds, are highly reliant on oxidative enzyme metabolism during exercise independent of the pattern of motor unit recruitment.12
Another study, however, concluded that endurance exercise can induce a shift of type II to type I muscle fibers.13 Beagles exercising on a treadmill 5 days per week for 55 weeks demonstrated an increase in type I fibers in the thoracic spinal muscles as well as the triceps muscle.13 The unique nature of canine skeletal muscle as well as the shortcomings of various staining and imaging techniques has made classification of canine skeletal muscle a difficult task, much less classifying changes as a result of endurance training. It is fairly well accepted that canine skeletal muscle has a relatively high capacity for aerobic metabolism.1 It may be likely that dogs experience changes in muscle fiber type in response to exercise; however, some of the shared characteristics of type I and type II fibers in dogs have led to misclassification, and therefore uncertainty in potential changes in muscles and their fiber types.1 Newer techniques used to identify canine muscle fiber types might provide insight into identification of possible fiber type conversion as a result of endurance exercise. In addition to a lack of concrete evidence to support the theory of muscle fiber type conversion, dogs undergoing endurance exercise have not shown an increase in muscle capillary density, capillary to fiber ratio, or capillary area to fiber ratio in some studies.12 The lack of a change in capillary density in skeletal muscle after endurance training suggests that microcirculation of untrained foxhounds is already adequate to meet the demands of endurance training or the overload needed to promote changes was not achieved in these studies. Even untrained dogs have a high aerobic capacity and appear to be genetically adapted for efficient oxygen extraction and use.
Other adaptations and alterations in body systems occur after endurance training that likely allow for improved performance during exercise. Although there may not be specific biochemical or histochemical change in muscle, there is some support that endurance exercise can improve the functional blood tissue gas exchange properties of skeletal muscle in dogs. A study evaluating endurance-trained dogs and dogs with one limb immobilized found that at a constant rate of oxygen delivery, endurance-trained dogs had an increased VO2 max, mainly as a result of increased oxygen diffusive conductance (DO2) in skeletal muscle.14 An interesting discovery, however, was that despite a 31% reduction in muscle weight, there was no difference in VO2 or DO2 between immobilized dogs and a control group during exercise.14
Strength of muscle refers to the maximal force a muscle or group of muscles can generate during one repetition. Muscle strength is associated with an increase in muscle size (hypertrophy). Type II muscle fibers are capable of developing more force per cross-sectional area and contract at a higher velocity than type I fibers (Figure 9-2). Strength training increases the size of type II fibers more than type I.10 High-resistance training increases the number of contractile proteins in type II fibers, which increases the cross-sectional area and the force they are able to generate. The enlargement of muscles that occurs with strength training is thought to mostly occur as a result of hypertrophy of muscle fibers and not hyperplasia (adding muscle fibers). Strength in dogs is mainly related to speed and the ability to carry or pull loads. Strength is also important for dogs that must accelerate and decelerate very rapidly, such as dogs that participate in agility competitions. Like strength, sprinting speed is also most affected by type II muscle fibers. The distribution of certain fiber types also depends on genetic factors. Sprinting breeds, such as greyhounds, have a greater percentage of type II muscle fibers, whereas breeds such as foxhounds have larger percentages of type I fibers and are more adept at endurance exercise. Short-duration, maximal-intensity workout programs are best suited for promoting an increase in strength or speed. In addition to conditioning muscles of the limbs it is also important to focus on spinal muscles. During sprinting exercise it has been observed that spinal muscles are among the first to experience fatigue.15 Incline treadmill running and uphill sprinting are good activities to strengthen muscles, including the spinal muscles. Other strengthening exercises include running or racing, pulling or carrying resistance loads, swimming against jets, and trotting or running up inclines or stairs. Performing strength exercise can increase the size of muscle groups and the force they are capable of generating. The opposite is true if skeletal muscle is subjected to periods of disuse or immobilization. Under these conditions, the muscles undergo atrophy and decreased muscle strength of the limb. A study that evaluated muscle atrophy caused by immobilization found that immobilization of the hindlimb for a period of 10 weeks reduced the diameter of type II fibers by 50% and type I fibers by 35% in the vastus lateralis muscle and maximum production of tetanic torque by the affected limb decreased by 50%.16 When removed from the immobilized state, growth hormones increased the size of muscle fibers and increased strength of the previously immobilized limb during the recovery period significantly more than a control group.16
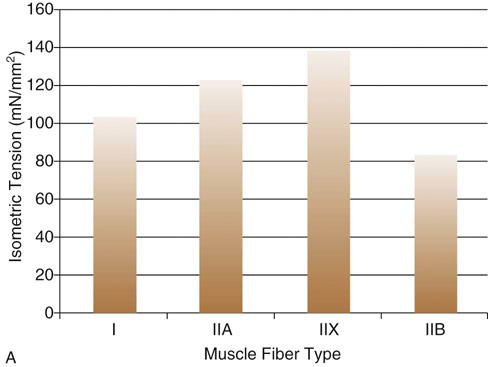
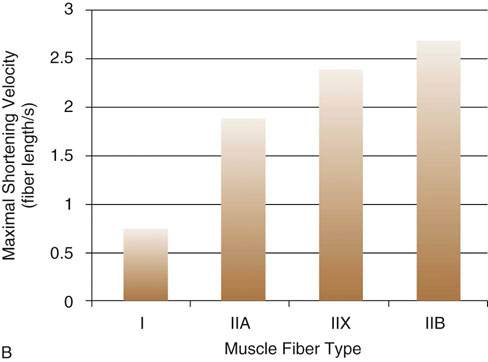
Respiration
The term respiration can relate to pulmonary or cellular respiration. This section discusses pulmonary respiration and the effect of exercise on ventilation. The term pulmonary respiration refers to ventilation of air into and out of the lungs and the exchange of gases between inspired or expired air and lung tissue. Ventilation refers to the mechanical movement of air into and out of the lungs. Diffusion is the process by which gas molecules move either from air into blood or blood into air. In addition to providing a means of supplying oxygen to the blood and tissues of the body and allowing for release of carbon dioxide, the pulmonary system plays an important role in regulating acid-base balance during rest and during exercise, as well as thermoregulation. Inflow of air to the lungs occurs as a result of intrapulmonary pressure dropping below atmospheric pressure, and expiration occurs when intrapulmonary pressure rises above atmospheric pressure. The most important inspiratory muscle is the diaphragm; however, any muscle capable of increasing the volume of the chest cavity can contribute to inspiration. Because of the elastic nature of pulmonary tissue and the thoracic cavity, expiration at rest is typically a passive event. During exercise, expiration can become partially active because of contraction of muscles of the abdominal wall.
Precise control of ventilation and pulmonary gas exchange during exercise is required to maintain PO2, PCO2, and acid-base balance. During exercise a change in ventilation is required to keep up with metabolic needs, which requires an increase in ventilation.17,18 Higher demand for oxygen and a need to rid the body of large amounts of carbon dioxide necessitate higher rates of ventilation. The respiratory control center in the medulla oblongata initiates the drive to inspire and expire. To increase ventilation, input to the respiratory center can come from both humoral and neural sources. At the onset of exercise, one theory states that impulses from the motor cortex to control skeletal muscle movement can “spill” over into the respiratory center as they pass through the medulla, causing an increase in ventilation.19 This is further supported by the fact that during strenuous exercise, arterial PO2, PCO2, and pH often remain almost the same as during resting. At the initial onset of exercise, ventilation increases prior to any significant changes in blood chemicals. This supports the theory that initial ventilatory changes are associated with signals sent to the respiratory center of the brainstem at the same time that signals are sent to skeletal muscle. Chemoreceptors in respiratory muscles may respond to potassium and H+ and send afferent information to the respiratory center. Central chemoreceptors detect increases in PCO2 and decreases in pH. Peripheral chemoreceptors are sensitive to increases in PCO2 and decreases in PO2 or pH. All of these receptors act to fine-tune ventilation during exercise. Input from higher brain centers, regulation of PCO2, and lowering of pH caused by increased blood lactate levels are all methods thought to increase ventilation during exercise.
The process of breathing at rest is clearly initiated in the CNS after input from other areas of the CNS, as well as peripheral signals. However, the exact causes of breathing in dogs during exercise prompted investigation to determine whether ventilation is an active or passive process. Some have proposed that the passive forces from limb movement in trotting dogs contribute a substantial amount to hyperpnea during exercise.20 The forces believed to affect respiration include the cranial and caudal displacement of the abdominal organs during acceleration or deceleration; concussive forces of limb impact, which change intrathoracic pressure; flexion and extension of the lumbosacral region resulting in compressive forces within the abdomen thus leading to intrathoracic pressure changes; and inertial forces on the chest wall caused by limb contact with the ground.20
A subsequent study demonstrated different results that contradict the proposition that breathing in dogs during exercise is largely a passive event associated with stride. It was concluded that breathing in exercising dogs is a neural-driven event. Diaphragmatic electromyographic activity, esophageal pressure changes, and phasic diaphragmatic shortening and breathing frequency remain strictly synchronous during exercise and are not significantly associated with stride.21 Active recruitment of the diaphragm and abdominal muscles is the major contributor to intrathoracic pressure changes and generation of airflow, and oscillations of abdominal contents associated with locomotion are not critical for airflow in exercising dogs.21
The diaphragm is required to perform very high levels of work to provide adequate ventilation during exercise. The diaphragm muscle is highly oxidative, preventing it from fatiguing easily. Historically, the muscles of the respiratory system were not thought to be a limiting factor during exercise. Respiratory muscle fatigue is defined as a condition in which there is a loss in the capacity for developing force or velocity of muscle contraction of respiratory muscles, resulting from muscle activity under load, which is reversible by rest.22 Studies have confirmed that respiratory muscle fatigue can occur during high-intensity exercise in humans.23 It is also believed that during prolonged high-intensity exercise, respiratory muscle fatigue can limit exercise performance in humans. One notable finding in humans was that fatiguing respiratory muscles cause reflex vasoconstriction to skeletal muscle of the limbs, resulting in exercise limitation of muscles, thus limiting some of the oxygen demand.23 There have been no studies evaluating respiratory muscle fatigue in healthy exercising dogs; however, the condition occurs in cases of cardiogenic and septic shock.24,25 It is likely that the highly aerobic nature of the canine diaphragm muscle normally protects it from fatigue.
Healthy dogs are likely to have a low likelihood of developing respiratory muscle fatigue. However, dogs with underlying conditions, especially those involving the respiratory system, are more likely to be susceptible to fatigue. Dysfunction of the respiratory system, such as pneumonia, increases the work load for respiratory muscles. Dogs engaged in strenuous exercise can reach high rates of ventilation. Although respiratory muscles such as the diaphragm may not fatigue easily during exercise, energy is still required to maintain such a high rate of ventilation. One consideration is that the cost of energy to maintain high rates of ventilation can represent a significant amount of total energy expenditure of a dog during exercise and thus represent a potential limiting factor of performance. A study that calculated the dynamic respiratory work of exercising dogs suggested that the higher rates of ventilation and elevated body temperature associated with exercise may induce changes that decrease the cost of breathing.26 Dogs exercising on a treadmill showed a decrease in dynamic respiratory work as a result of decreased airway resistance that resulted in up to an eightfold savings of energy expenditure of respiratory muscles. When body temperature rises to more than 39° C and respiratory rate increases to 120 breaths per minute, there is an apparent decrease in dynamic respiratory work as a result of lower airway resistance.26 It is unknown whether there is a progressive decrease in airway resistance as body temperature rises or if a sudden change in breathing frequency induces the change. It is believed that the most likely site of resistance reduction is at the level of the larynx. During states of hyperthermia, cats have reduced laryngeal resistance to almost half of normal values.27 The lower airway resistance in exercising dogs decreases the amount of energy required to maintain the rates of ventilation required for strenuous exercise. This mechanism may help resist respiratory muscle fatigue as well as conserve energy for use by skeletal muscles in the trunk and limbs.
Depending on the environment a dog exercises in, it is possible for ambient temperature to have direct effects on the respiratory system of athletes. Repeated exercise in cold environments can result in airway disease. Dogs exercising in cold conditions can develop pulmonary changes similar to a condition known as “ski asthma” in humans. Dogs exercising for prolonged periods in cold environments show significantly more reactivity to aerosol histamine and overall greater airway resistance when compared with dogs not racing in cold environments.28 This type of dysfunction can persist for at least 4 months after rest. It is believed that continuous cold air hyperventilation causes excessive heat and water loss, leading to airway mucosal injury. The specific effects of cold weather changes of the respiratory system on exercise performance have not been fully evaluated. However, any condition that results in inflammatory changes of the airways would likely have some degree of negative effect on performance.
Cardiovascular System
The primary purpose of the cardiovascular system is to deliver oxygen and nutrients to the tissues of the body as well as remove wastes and aid in temperature regulation. To accomplish these tasks, the cardiovascular system works in conjunction with the respiratory system. During exercise, cardiac output must increase in proportion to the increase in metabolic rate. In human athletes there is a linear relationship between percent maximal oxygen uptake (VO2 max) and cardiac output. Cardiac output is the quantity of blood pumped into the aorta each minute by the heart and is determined by the product of the heart rate and volume of blood ejected per heartbeat (stroke volume). This in turn determines the amount of blood that flows through the circulation. An increased demand for oxygen and nutrients by skeletal muscles during exercise requires an increase in cardiac output as well as redistribution of blood from inactive organs to active skeletal muscle.
The onset of exercise induces changes that increase cardiac output. The initial changes in the cardiovascular system at the onset of exercise are thought to originate from centrally generated motor signals followed by modification and fine-tuning from baroreceptors, chemoreceptors, and mechanoreceptors throughout the body.29 Both inhibition of the parasympathetic and activation of the sympathetic nervous system act to increase heart rate and strength of contraction during exercise. Stroke volume is also increased during exercise. Venous return to the heart is increased by venoconstriction, which decreases the storage capacity of the venous system and causes blood to move back toward the heart. The mechanical action of contracting skeletal muscle compresses veins to push blood toward the heart. The rhythmic action of breathing also promotes blood flow from the abdomen to the thorax. Increased flow of blood to the heart increases the volume of blood in the ventricles at the end of diastole (preload) available for the heart to pump back into circulation for skeletal muscle demands. The increased preload also acts to increase the strength of muscular contraction by way of the Frank-Starling mechanism.
Other methods of meeting the increased oxygen demands of skeletal muscle during exercise are to increase blood flow to muscle by decreasing blood flow to other organs and vasodilatation of skeletal muscle arterioles. Skeletal muscles in resting humans receive approximately 15-20% of total cardiac output; during maximal exercise this increases to 80-85% of total cardiac output.29 There is some controversy as to the exact mechanism by which skeletal muscle blood flow increases, and blood flow to other organs decreases during exercise. During exercise, vascular resistance in skeletal muscles decreases while vascular resistance in visceral organs increases.30 Initial vascular resistance within skeletal muscle is believed to decrease as the result of withdrawal or attenuation of sympathetic input to skeletal muscle arterioles; however, maintenance of vasodilatation is likely due to intrinsic control caused by local changes in metabolites such as pH, oxygen tension, and nitric oxide.29,31 Another possible mechanism that acts to increase blood flow to muscle in dogs is the concept of sympatholysis, which was demonstrated by attenuation of vasoconstrictor response during dynamic exercise compared with a resting state.32
Studies have been performed regarding the effect exercise has on the cardiovascular system of dogs. Similar to humans, changes in the cardiovascular system of dogs are required to meet the metabolic needs of skeletal muscle during exercise. Sled dogs, some of the most elite canine athletes, have provided invaluable information on the cardiovascular system during maximal exercise. The heart rate of sled dogs can dramatically increase at the onset of exercise. Heart rate can increase from a resting rate of 50 beats per minute to 300 beats per minute and remain between 250-300 beats per minute during a 60-minute run.33 Foxhounds exercising on a treadmill show similar trends of change in heart rate. Heart rate continues to increase as dogs are subjected in increasing levels of submaximal exercise. When foxhounds reach a state of maximal treadmill exercise (approximately 12 km/hr on a 28% grade), heart rates approach 300 beats per minute (Figure 9-3, A). When comparing heart rate of exercise-trained and untrained foxhounds at different levels of submaximal exercise, trained dogs have on average a heart rate 14 beats per minute lower than untrained dogs at each level of measured submaximal exercise.34 Exercise also affects blood pressure. Systolic pressures can increase to more than 200 mm Hg during exercise. However, diastolic pressures during exercise are frequently lower than resting values, resulting in a mean pressure similar to that of resting values33 (Figure 9-3, B). These changes are the result of arteriolar vasodilatation at the level of skeletal muscle, which decreases diastolic blood pressure.
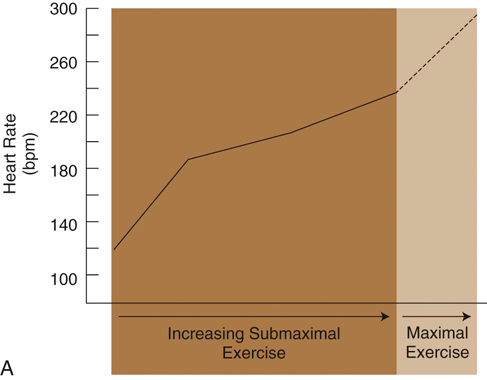
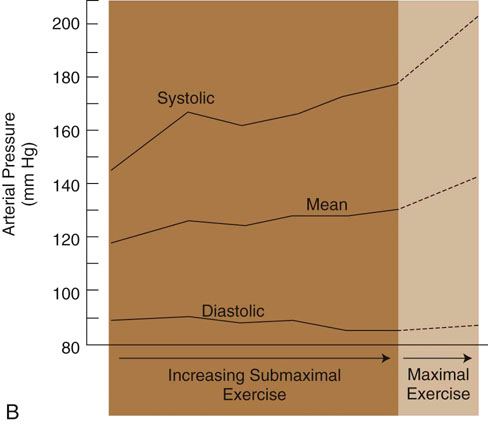
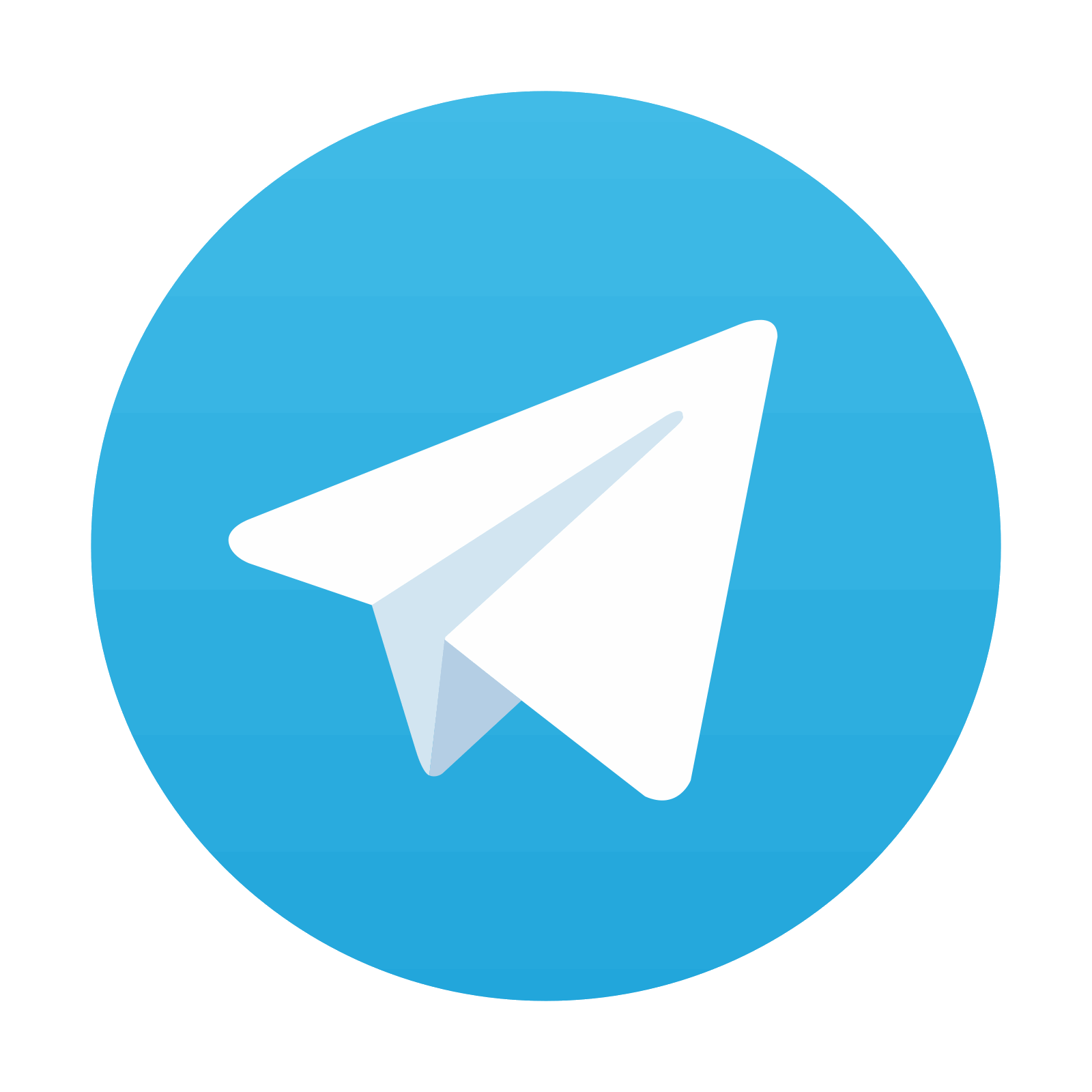
Stay updated, free articles. Join our Telegram channel
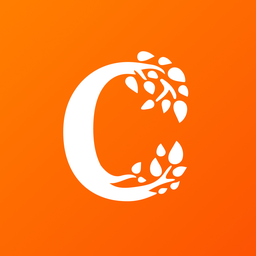
Full access? Get Clinical Tree
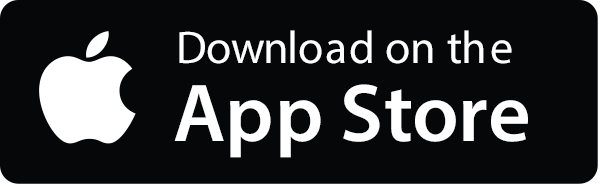
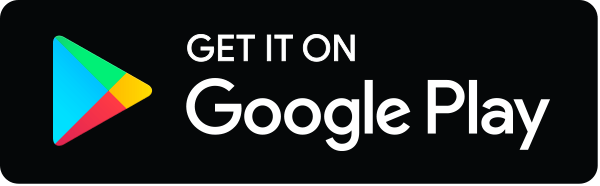