Patients afflicted with neurologic, orthopedic, metabolic, and other diseases frequently have reduced weight bearing and use of limbs. In addition, some conditions may be treated with splints, casts, or other coaptation devices, resulting in decreased limb use. The tissues most affected by disuse and immobilization are cartilage, joint capsule, muscle, ligament, tendon, and bone. Knowledge of how these tissues respond to disuse and immobilization, the types of deleterious tissue changes, and the time frame over which these changes occur, are important in understanding the need for physical rehabilitation. For example, stress deprivation of joints results in proliferation of connective tissue within the joint space, adhesions between synovial folds, adherence of connective tissue to cartilage surfaces, atrophy of cartilage, reduced proteoglycan (PG) content, ulceration at points of cartilage to cartilage contact, disorganization of cellular and fibrillar ligament alignment, reduced collagen mass, increased ligament compliance, weakened ligament insertion sites, reduced load-to-failure and energy-absorbing capacity of the bone-ligament-bone complex, and osteoporosis of the involved extremity.1 Rehabilitation of patients with acute or chronic neurologic or orthopedic conditions involves the application of controlled challenges to tissues to improve strength, condition, and function. It is important to understand how to safely remobilize tissues after injury and a period of immobilization. Rehabilitation must sufficiently challenge tissues to enhance and positively influence their recovery and healing. However, if tissues are overchallenged, they may be damaged, ultimately delaying recovery or cause additional injury. There must be a balance between the simultaneous demands for protection against undue stress to facilitate healing, and the need for stress to attenuate atrophy of musculoskeletal tissues.2 A review of these concepts forms the basis for a rational approach to developing rehabilitation programs. Disorders of articular cartilage and joints are some of the most common and debilitating diseases encountered in veterinary practice. Understanding the normal structure and function of articular cartilage is a prerequisite to understanding the pathologic processes. The mechanical properties of articular cartilage arise from the complex structure and interactions of its biochemical constituents. The viscoelastic properties of cartilage, primarily resulting from fluid flow through the solid matrix, explain deformational responses observed during loading. For example, cartilage that is loaded rapidly is stiffer than the same cartilage that is loaded slowly. Degenerative processes include the breakdown of normal constituents of cartilage, especially the collagen network and PGs, which affects the mechanics of fluid flow through cartilage. Factors contributing to such breakdown include direct trauma, obesity, immobilization, and excessive repetitive loading of the cartilage. Training activities, without traumatic injury, do not appear to be a risk factor for developing osteoarthritis (OA) in normal joints, but such activity may be harmful to an abnormal joint.3 The extracellular matrix is composed of collagen, PGs, and water. The orientation of collagen and PGs functions to distribute forces over the subchondral bone and provides a smooth surface that allows movement of joints (Figure 7-1).3 Collagen provides tensile strength to the cartilage, and structural support for the extracellular matrix. Type II collagen is the primary form in articular cartilage and is unique to cartilage. Type IX collagen is also unique to articular cartilage and links type II fibrils together, limits the separation of collagen fibrils, limits fibril diameter, and binds PGs to collagen. Processes resulting in articular cartilage loss may cause collagen degradation, either enzymatically, mechanically, or both. Type IX collagen is especially susceptible to enzymatic degradation. PGs make up most of the extracellular matrix that is not collagen, and compose 22% to 38% of the dry weight of articular cartilage. PGs are highly hydrophilic, which results in water retention, creating swelling pressure and turgidity that is essential to articular cartilage function. A PG monomer consists of a core protein with one or more types of glycosaminoglycan (GAG) chains attached. The core protein has a hyaluronan binding region, GAG binding regions, and a carboxy-terminal end. A link protein helps to bind the PG chain to hyaluronan (Figure 7-2). Hyaluronan is a nonsulfated GAG located in the extracellular matrix and forms the backbone to which the PG monomers are covalently bound. Aggrecan refers to a PG monomer that combines with hyaluronan. Water composes 65-80% of total cartilage weight.3 Although PGs are highly hydrophilic, enclosure in the collagen meshwork limits their ability to expand. This keeps cartilage turgid, resisting deformation. Water moves slowly within the cartilage matrix during loading as a result of resistance of flow through the collagen meshwork and the hydrophilic nature of the PGs. Water is forced out of articular cartilage during loading and some water weeps onto the articular surface, allowing hydrostatic lubrication. When the load ceases, water is reabsorbed. With rapid loading, cartilage is stiffer because water distributes more slowly, resulting in less compression of the cartilage. Cartilage is more compliant with slow loading because there is additional time for fluid movement to the cartilage surface. Clinicians who attempt to restore joint function should understand the effects of immobilization on articular cartilage (Box 7-1). Disuse of cartilage may result in atrophic or degenerative changes, and these changes are more marked and appear sooner in areas of contact.4 Immobilization leads to a decrease in matrix and cellular components, disorganization of cartilage, and potential irreversible damage if immobilization is prolonged.5 In addition to a reduction in synovial fluid production with immobilization, nutrition to cartilage is diminished as a result of reduced synovial pumping and nutrient diffusion. The age of the dog, as well as the form of immobilization used, may affect results. Several models of joint immobilization and disuse have been studied, including casting with the limb in flexion or extension, external skeletal fixation, and non–weight-bearing models (such as sling application). The changes in mechanical behavior and biochemical composition of articular cartilage appear to be less dramatic in limbs immobilized in a sling than in limbs immobilized in a cast. Adult greyhound dogs had stifles placed in a sling at 90 degrees of flexion for 4 or 8 weeks, which allowed some limited motion of the stifle but no weight bearing. There were no changes in the compressive properties of cartilage, PG content, collagen content, or cartilage thickness.6 This suggests that immobilization with a sling may be less deleterious to articular cartilage as compared with other models of joint disuse in which cartilage changes are both progressive and degenerative.6 Immobilization with a joint in flexion does not generally lead to arthritic changes in the short term, but cartilage atrophy may occur. Immobilization of a joint in extension results in increased muscle contraction against the immobilization device and changes in articular cartilage similar to those seen in OA. The cartilage of limbs of young dogs immobilized in flexion for 3-11 weeks was grossly normal with no osteophytes.7,8 However, there was a 13% to 60% early and progressive reduction of PG content following immobilization, with the depletion being most severe in the superficial zones.4,8–10 Immobilization for as little as 6 days may result in a 40% reduction in cartilage PG synthesis, and up to 60% for joints immobilized for 8 weeks.4 In addition, there is a 10-20% increase in water content. The accelerated PG loss appears to be caused by a combination of decreased synthesis and increased proteolysis of PGs.11 The loss of PGs is somewhat selective, with preferential loss of chondroitin-6-sulfate.9 The levels of synovial fluid PG components, including link protein, keratan sulfate, and total sulfated GAG, were determined in dogs after a 3-week recovery period following either 4 or 8 weeks of immobilization with the stifle in flexion.12,13 The concentrations of keratan sulfate and sulfated GAG in the synovial fluid lavages were increased after disuse and after disuse with recovery compared with controls, but the levels of link protein remained low. This pattern of catabolism differs from that found in OA, in which link protein is elevated in synovial fluid lavages, along with keratan sulfate and sulfated GAG. After 3 weeks of immobilization, PG aggregation is poor. These changes are reversible, however, because PG aggregates were normal 2 weeks after removal of a cast that had been worn for 6 weeks.4 The interaction of PGs with hyaluronic acid (HA) may be reduced in older dogs because of an abnormality in the HA binding region of the PG core protein,4 but others have shown no abnormality in aggregation in young dogs.9,11 Young dogs subjected to 11 weeks of immobilization by splinting had a significant reduction in hyaluronan concentration in the tibial and femoral condyles, and the patellar surface of the femur, with a concurrent decrease in aggrecan, but the ratio of hyaluronan to aggregan was unchanged.14 Young dogs may respond differently, because there was no change in hyaluronan content in skeletally mature dogs following 4 to 8 weeks of immobilization, as compared with an 80% decrease during early OA.10 This difference supports the concept that the mechanism of cartilage degradation in disuse and OA is different, and immature dogs may respond differently than older dogs to immobilization. It may also help explain why the changes in cartilage following immobilization may be reversible, whereas the changes in osteoarthritic cartilage are not reversible. Cartilage thickness may be reduced 30-50% with immobilization, but the location of thinning within the cartilage is not consistent. In mature dogs, thinning occurs primarily in the uncalcified cartilage, whereas in young dogs it tends to occur in the calcified cartilage under the tidemark.4,8 Immobilization may result in 30% fewer chondrocytes per unit area in cartilage.4 The subchondral bone also atrophies. Sling immobilization of the stifle for 4 weeks results in elevation of gelatinase (neutral metalloproteinase 2 [MMP-2]) and almost complete suppression of tissue inhibitor of metalloproteinases (TIMP), especially in the superficial zone, as well as reduced protein synthesis by chondrocytes.15 In contrast, collagenase activity is normal. The return of MMP-2 and TIMP levels to normal was observed with a 2-week remobilization period or treatment with insulin-like growth factor-1 (IGF-1) and pentosan polysulfate. This suggests that cartilage is remodeling, similar to bone remodeling in disuse atrophy, but the material properties of the articular surface remain relatively intact following a period of immobilization with a sling. Contact forces between articulating surfaces appear to be necessary to maintain normal PG content of articular cartilage.9 The greatest depletion of GAGs (64%) occurred at the cranial and caudal extremes of the femoral condyles in the stifle joint cartilage of young beagle dogs following cast immobilization for 11 weeks in 90 degrees of flexion. These were locations where the immobilized cartilage lost contact with the opposing cartilage.9 The biochemical changes that occur with immobilization also affect the biomechanical properties of articular cartilage. Splint immobilization of stifle joints of young dogs for 11 weeks caused significant softening of femoral and tibial cartilage with no visible changes of the cartilage surface.16 The rate of deformation during loading increased 42% and the average thickness of the cartilage decreased 9% as compared with controls. Loading of cartilage may be necessary to maintain cartilage stiffness, because stiffness was maintained in the contact area between the patella and patellar surface of the femur as a result of sustained loading between the femur and the patella produced by the flexion of the knee joint, whereas cartilage softening was greatest in nonloaded areas. There is correlation between cartilage stiffness and PG concentration. Therefore loss of PGs during immobilization may also affect biomechanical properties. Immobilization of stifles with a cast caused significant softening of up to 30% in the lateral femoral and tibial cartilages.17 The GAG content of the cartilage was slightly decreased after immobilization, especially in the superficial zone of cartilage. The changes in stiffness correlated with alterations in GAG content of the superficial and deep zones. This confirms the key role of PGs in the regulation of cartilage stiffness. There may also be changes in the compressive and shear stiffness of cartilage from nonimmobilized contralateral limbs, possibly as a result of increased loading.18 The changes that occur to articular cartilage during immobilization are probably caused by a combination of decreased joint motion and reduced loading. Although both are important in maintaining cartilage integrity, normal loading is particularly important with contraction of muscles that span the joint and stabilize the limb during weight bearing.6 In a non–weight-bearing model that allowed joint motion of the stifle joint, cartilage thickness, PG content, and PG synthesis were reduced, whereas water content was increased.19 These changes were similar to those that occur with immobilization in a flexed position and suggest that weight bearing is necessary to prevent the bulk of changes. Some joint motion during immobilization is beneficial. Stifles immobilized for 6 weeks with a cast, allowing 8 degrees to 15 degrees of motion, had less PG loss and a smaller decrease in PG synthesis than occurred with more rigid fixation. The limited motion provided by casting was protective.11 Elimination of motion by rigid fixation induces even more pronounced atrophic changes to articular cartilage. To study the effects of immobilization on the ultrastructure and surface contour of articular cartilage, knee joints of adult dogs were immobilized with circular external fixation for two to four weeks.20 The degree of cartilage changes increased with the length of immobilization, and consisted of destruction of the superficial cartilage layer, cleft formation in the surface, and erosion of the articular cartilage. In another study, knees of mature dogs were immobilized for 6 weeks with external fixators. There was a 7% increase in water content compared with normal knee cartilage, and a 28% decrease in GAG content, which was greater than if some motion was allowed.11 More importantly, GAG content did not return to normal after a 1 week remobilization period as it did in dogs that were not rigidly immobilized. Spinal cord injury, which may result in reduced mobility and decreased joint motion, may also result in cartilage thinning.21 Immobilization of the stifle in flexion may be beneficial when joint instability exists, such as occurs with a ruptured cranial cruciate ligament (CCL), because bearing weight on an unstable stifle results in OA. Dogs undergoing transection of the CCL had their stifle joints maintained in flexion or were permitted ad libitum ambulation for 12 weeks.22 Dogs allowed to bear weight had osteophytes, fibrillation, and decreased cartilage PG content, although cartilage thickness was normal. PG synthesis was 80% greater than that in cartilage from the contralateral knee. In contrast, osteophytes were not seen when the limb was immobilized in flexion immediately after transection of the ligament, and the articular surface remained intact. The cartilage became atrophic, however, and PG content and PG synthesis were decreased compared with cartilage from the contralateral knee. Knee cartilage from immobilized limbs after cruciate ligament transection, therefore, resembled that from dogs whose limbs were immobilized without ligament transection (Box 7-2). The effect of position of the limb during immobilization on blood flow to the joint must also be considered. For example, forced abduction of experimentally induced hip dysplasia with secondary OA results in significant reduction of blood flow, whereas immobilization in flexion results in the highest blood flow to the femoral head.23 The response of cartilage to remobilization depends on the biomechanical demand that the joint is exposed to, the condition of the cartilage, and the length of the immobilization and remobilization period (Box 7-3). If the joint is subjected to high stresses and repeated loading immediately after immobilization, cartilage may not be able to resist the stresses and may become damaged because of injury to the softened matrix. The duration and the degree of load bearing after immobilization are factors that determine the cartilage response. Also, the ability to restore biomechanical properties may depend on an intact collagen network. The atrophic changes of cartilage that occur with a limb casted in flexion are somewhat reversible. Remobilization of a normal limb casted in flexion for 6 weeks by allowing ambulation for 3 weeks after cast removal resulted in the cartilage becoming normal.4 Longer periods of immobilization in young dogs may result in more long-standing effects, even with a gentle remobilization period. Skeletally immature female beagle dogs were immobilized in a cast for 11 weeks in 90-degree flexion followed by remobilization for 15 weeks.9 After remobilization, PG content remained an average 18% lower than controls, with content at the minimum contact sites 33% lower. GAG concentration was restored in the more loaded regions of the patellofemoral region and tibial condyles, but remained lower than the control values in the less loaded peripheral regions of the femoral condyles.24 Uncalcified cartilage thickness of the femoral condyles was 15% less than in the controls, and the thickness of the calcified zone was reduced in most regions of the remobilized stifles. The thinness of the calcified zone may be due to advanced bone growth from the subchondral bone during remobilization. Softening of the articular cartilage during immobilization may expose the calcified zone to increased mechanical loading and result in mineralization and thickening of subchondral bone. The changes induced by unloading are reversible to a great extent, but full restoration of articular cartilage may not occur at all sites following 15 weeks of remobilization. Immobilization of the skeletally immature joint therefore may affect the development of articular cartilage in such a way that very slow recovery or permanent alterations are induced. Longer periods of remobilization may be more beneficial. Hindlimbs of immature dogs were immobilized for 11 weeks and then remobilized for 50 weeks.17,25,26 Immediately after the immobilization period, cartilage GAG concentration was reduced similar to that found in other studies. The amount of collagen crosslinks was also reduced during immobilization. After 50 weeks of remobilization, GAG concentration was restored at most sites, but remained 9-28% lower than controls in the patella, medial femoral condyle, tibial condyles, and the proximal femoropatellar surface. Collagen crosslinks were restored to control levels, indicating that collagen is resistant to reduced joint loading and slow remobilization. Although the articular cartilage of immobilized limbs became similar to control cartilage following long periods of remobilization, full restoration of articular cartilage GAG concentration was not obtained in all sites, even after remobilization for 50 weeks. This suggests that joint immobilization of young dogs can cause long-lasting articular cartilage PG alterations. Because GAGs exert a strong influence on the biomechanical properties of cartilage, the biochemical changes that occur in cartilage during remobilization may also affect the biomechanical properties. The restoration of the biomechanical properties of articular cartilage was studied after 15 weeks of remobilization of the knee joint in mature beagles previously immobilized with a cast for 11 weeks.27 Remobilization improved the decreased stiffness and cartilage thickness in femoral, tibial, and patellar cartilage created by immobilization, and approached values of nonimmobilized controls. However, stiffness of the femoral condylar cartilage remained lower and cartilage permeability was higher compared with the controls. A study with the same period of immobilization but with a remobilization period of 50 weeks resulted in restoration of the biomechanical properties of cartilage in the lateral condyle of tibia, but not in the lateral condyle of femur, where stiffness remained 15% below the control level following remobilization.17 Similar to biochemical changes, articular cartilage biomechanical properties return toward normal during remobilization, although the recovery is not complete in all parts of the knee even after 50 weeks of remobilization. Immobilization of joints of young dogs may cause long-term, if not permanent, alterations of cartilage biomechanical properties, similar to changes observed in biochemical components of articular cartilage. This may predispose joints to degenerative changes later in life. The atrophic changes seen in cartilage following immobilization are less reversible following immobilization with a rigid external fixator. However, in one study, 2 to 4 weeks of remobilization following 2 to 4 weeks of immobilization with an Ilizarov external fixator demonstrated that the longer the period of remobilization, the greater the reparative processes.20 In contrast to the improvements seen with mild remobilization strategies, vigorous exercise after a period of immobilization may have deleterious effects on cartilage.28 Dogs that ran daily (9.5 km/d, 5 km/h) on a treadmill for 3 weeks after cast removal had continued decreases in cartilage thickness (20%) and PG content (35%), even though net PG synthesis was 16% greater than in cartilage from contralateral nonimmobilized stifles. Therefore vigorous loading of the joint following a period of immobilization may prevent reversal of cartilage damage and ultimately be detrimental to cartilage. It appears that the atrophic changes are more reversible with gentle spontaneous reloading. Various experimental models for producing atrophy of meniscal tissue have yielded contradictory results. These models have included denervation of the stifle, immobilization (both internal and external), and disarticulation. Active motion of the stifle may play a more important role than weight bearing in preventing meniscal atrophy. In one study, internal skeletal fixation, which did not allow motion of the stifle joint, was applied for 12 weeks. There was significant atrophy of the lateral meniscus, despite the opportunity for weightbearing.29 Another study evaluated the effect of active joint motion on the maintenance of meniscus mass in a unilateral ankle disarticulation non–weight-bearing model of disuse. This model maintained active knee motion without weight bearing. Despite the loss of collagen and calcium mass in the femur and tibia because of decreased weight bearing, there was no loss of meniscal mass.30 Although meniscal mass may be maintained when motion is allowed in stifles with limited weight bearing, there may be profound changes in biomechanical and biochemical properties. Meniscal cartilage stiffness decreased by 30% 8 weeks following transection of the CCL and immediate stabilization of the stifle in one study, despite the normal appearance of the meniscus.31 Although weight bearing and joint motion were not restricted following surgery, limb use was reduced and it is possible that a combination of inflammation and decreased limb use after surgery resulted in decreased stiffness. Chronic stifle instability may result in further deterioration of the meniscus because clinical cases of cranial cruciate ligament rupture (CCLR) undergoing partial meniscectomy had a 60% decrease in meniscal stiffness. The effect of early motion on healing meniscal repairs has been evaluated in humans at the time of anterior cruciate ligament (ACL) reconstruction. In one study, 80% of patients undergoing immediate postoperative range of motion (ROM) and early partial weight bearing had complete meniscal healing with no deleterious effects of immediate knee motion on meniscal repairs.32 Although meniscal repair is not generally performed in dogs, studies of healing medial meniscal incisions demonstrated that dogs with immediate limb mobilization had greater collagen content in the healing meniscus than those that were immobilized in a cast.33 There were no significant differences in collagen between the repaired menisci with mobilization and the control menisci. Prolonged immobilization resulted in decreased collagen formation in healing tissue. The vascular response during meniscal healing is also affected by immobilization.34 In a study of rabbits with meniscal injury, meniscal blood flow was increased fivefold in animals in which the stifle was allowed motion, but this increase was prevented by immobilization 4 weeks after injury. Furthermore, healing was diminished in immobilized knees. Partial or complete meniscectomy is more commonly used to treat meniscal damage in dogs. In general, meniscal removal results in degeneration of articular cartilage, and cartilage stiffness is reduced.35 Less severe changes occur with partial meniscal resection, but the changes are greater on the tibial surface as compared with the femoral surface.36,37 Early joint motion may be beneficial to these patients. In studies of bilateral medial meniscectomy in dogs, one group was allowed immediate joint movement, and another group underwent knee joint immobilization with an external skeletal fixator (ESF) for 5 weeks immediately following meniscectomy. After removal of the fixation, the animals were allowed free movement for 21 weeks.38,39 The normal menisci and the regenerated tissue at the meniscectomy sites were examined for collagen content, collagen assembly, and GAG distribution. Although the collagen content of the regenerated tissue was not different from normal menisci, collagen fiber development and GAGs were not normal. Movement following meniscectomy is likely beneficial to matrix formation in the regenerating tissue. Regarding articular cartilage, twice as much PG was present in femoral condyles of meniscectomized animals encouraged to bear weight on joints immediately after surgery compared with controls or joints immobilized after surgery. Many reports have suggested an association between the biochemical and biomechanical properties of cartilage and the mechanical stress to which it is subjected. For example, the increased weight bearing in the limb opposite a limb casted in flexion resulted in a 19% increase in uncalcified cartilage thickness and a 25-35% increase in GAG concentration in the intermediate, deep, and calcified zones of the femoral condyles.8 It appears that increased weight bearing augments local PG content of the articular cartilage matrix. In fact, PG content and cartilage thickness are generally greater in central weight-bearing areas than in peripheral areas, and contact forces help maintain normal cartilage matrix.8 In general, chondroitin sulfate predominates in pressure-bearing regions, whereas dermatan sulfate predominates in tension-transmitting regions of connective tissues.40 The influence of static and intermittent stress on articular cartilage metabolism was examined in vitro using full-thickness plugs of cartilage from femoral condyles of normal adult dogs.40 When cartilage was exposed to a static stress equivalent to three times body weight, or to cyclic stresses at a duty cycle of 60 seconds on–60 seconds off, net GAG synthesis was suppressed to 30-60% of that in controls. Protein synthesis also decreased, and water content increased. In contrast, when a duty cycle of 4 seconds on–11 seconds off was used, GAG synthesis was increased by 34%, but protein synthesis or water content was not affected. The changes in GAG synthesis do not appear to be due to changes in diffusion of nutrients during loading. These results suggest that regular, intermittent stresses, such as those obtained with walking, are important to maintain normal articular cartilage. Continuous strong compression or heavy-impact loading to joints may cause deterioration of articular cartilage.41 Blunt trauma applied to cartilage results in changes in the zone of calcified cartilage, with an increase in cellular clones, bony bar formation in the calcified cartilage, vascular invasion, and decreased PG content, thickness of the calcified cartilage, and the number of chondrocytes.42,43 Blunt trauma to articular cartilage causes significant alterations in the deeper layers of cartilage, without disruption of the articular surface. The adaptation that occurs in functioning joints is known as Leed’s hypothesis, which states that cartilage becomes conditioned to transmit, without sustaining damage, the stresses to which it is most regularly subjected.27 Exercise places biomechanical and physiologic demands on articular cartilage.44 Mild to moderate levels of running in dogs may stimulate adaptation, but strenuous levels can cause detrimental articular cartilage changes (Box 7-4). There may be activity thresholds below and above which articular cartilage is maintained or destroyed. Between these thresholds, cartilage seems to adapt to the loads placed on it. Intermittent compression tends to stimulate chondrocyte biosynthesis. Joint motion without compression results in articular cartilage thinning, and static loading causes decreased chondrocyte biosynthesis.44 Cartilage subjected to high stress has higher PG content and is stiffer than cartilage exposed to low stress levels. Most studies of moderate running indicate no injury to articular cartilage, assuming there are no abnormal biomechanical stresses acting on the joints. Young beagle dogs running 4 km/d, at a speed of 4 km/h at a 15-degree incline on a treadmill for 15 weeks had no macroscopic damage to the cartilage surface and a 6% increase in mean cartilage stiffness, with most of the increase occurring on the patellar surface of the femur and the tibial condyles.45 Cartilage thickness also increased by a mean of 11%, with increases of 19-23% in the lateral condyle and patella surface of the femur. In addition, there was a 28% increase in GAG content of the femoral condyles, located mainly in the intermediate and deep zones.46 This degree of exercise had a mildly anabolic effect on properties of cartilage. Running 20 km/d on a treadmill for 15 weeks did not further improve mechanical properties (Figure 7-3).47 Skeletally immature dogs subjected to 15 weeks of running exercise at a rate of 40 km/d had no change in cartilage total hyaluronan or PGs.14 However, running 20 km/d for nearly 1 year resulted in a 6% reduction in thickness of the uncalcified cartilage of the medial femoral condyle, with an 11% reduction in GAG content.48 Although tibial cartilage was stiffer, femoral condyle stiffness was unchanged with this degree of training.47 In similar studies of running 40 km/d, the effects of training for 1 year on young canine articular cartilage were investigated and found that the cartilage response to running training was site dependent.49,50 Cartilage stiffness decreased 12-14% in the lateral, but not in the medial condyles of the femur and tibia, perhaps because of excessive impact loading and damage to the lateral region.49 GAG content was depleted in the superficial weight-bearing areas of the joint, including the lateral femoral condyle.50 There were slight increases in the thickness of the uncalcified and calcified cartilage, and the subchondral bone.51 There also appeared to be reorientation of the superficial zone collagen network.52 Although no overt cartilage damage was seen, softening of the cartilage may jeopardize the ability of cartilage to maintain its normal structural and functional properties over time. The response to long-term exercise may be different in other joints. Although there was a decrease in GAG content and cartilage thickness in the head of the humerus following training, cartilage stiffness was not affected.49,50 The articular cartilage of the femoral head appears to adapt very well to long-term distance training.53 Strenuous training protocols in older dogs may result in deleterious changes to cartilage. Running aged dogs on a treadmill at 9.6-12.8 km/h for 1 h/d, 6 d/week for 8 months led to matrix degradation in the femoral head. PG content was decreased, and there was irreversible destruction of collagen fibrils, with erosion and fibrillation of the cartilage surface. Older dogs may be more vulnerable to mechanical stress changes than younger animals.54 A study attempted to use magnetic resonance imaging (MRI) of cartilage and serum and synovial fluid markers of cartilage damage to determine if training injury to cartilage could be detected and monitored in dogs training at different intensities.55 Three groups were evaluated: (1) a training group that ran on a slope of 10 degrees at a speed of 200 m/min, (2) an intensified training group that ran on a slope of 10 degrees at a speed of 300 m/min, and (3) a control group that was allowed unrestricted cage activity. Dogs in the training and intensified groups were trained daily, running for an hour in the morning and in the afternoon for 10 weeks. Changes in the MRI examination suggestive of early injury of articular cartilage were noted in the training and intensified groups as early as 2 weeks of training. Damage was most severe at 4 to 6 weeks, and then gradually improved. There were no differences between the two training groups. Elevations of cartilage oligomeric matrix protein, matrix metalloproteinases 1 and 3, and tissue inhibitor of matrix metalloproteinase 1 in serum and synovial fluid were seen in both training groups. Histologic examinations at the end of training demonstrated cartilage damage and repair in the stifle joints in the training and intensified groups; furthermore, the intensified group indicated more remodeling of articular cartilage. The authors concluded that high-intensity training may induce injury, followed by a repair process. In addition, changes in biomarkers reflected pathologic changes in the cartilage better than MRI. One study evaluated the effect of lifelong low-impact exercise on articular cartilage.56 Dogs were exercised on a treadmill at 3 km/h for 75 min, 5 d/wk for 527 weeks while wearing jackets so that the total weight carried was 130% of body weight. There were no ligament, meniscal, or cartilage injuries, or osteophytes found on necropsy. Biochemical, cartilage thickness, and biomechanical properties were not affected by training. These results suggest that a lifetime of relatively low-impact weight-bearing exercise in dogs with normal joints does not cause alterations in the structure and mechanical properties of articular cartilage that might lead to joint degeneration.56 Excessive exercise during periods of acute joint inflammation may exacerbate synovitis. Passively exercising the stifle joints of dogs in a model of acute inflammation for 5 seconds every 15 minutes for 4 hours resulted in a twofold increase in the synovial fluid leukocyte count, whereas exercise for 5 minutes every 15 minutes for 4 hours produced a ninefold increase in inflamed joints.57 Forms of acute inflammatory arthritis may require periods of physical inactivity for recovery. Observations of humans with rheumatoid arthritis suggest that excessive activity may increase the severity of the disease.58 Immobilization was chondroprotective to guinea pig stifles following an intraarticular injection of iodoacetate. Animals that were not immobilized had decreased GAG content and a 10-20% reduction in the number of chondrocytes 1 week after injection. Three weeks after injection, cell death and loss of GAG progressed, and surface fibrillation and osteophytes developed. Although the loss of GAGs was not altered by immobilization after iodoacetate injection, the depletion of chondrocytes was reduced. Furthermore, neither osteophytes nor fibrillation developed.59 Therefore immobilization may be protective against exacerbation of synovitis during acute bouts of inflammatory joint disease. However, isometric exercise does not appear to increase joint inflammation.60 The effects of passive range of motion and isometric exercises on experimental inflammatory arthritis in a rabbit model were assessed. Passive range of motion significantly increased synovial leukocyte counts over control values of the nonexercised joints, but isometric exercises across a joint with acute synovitis did not affect synovial leukocyte count. Another factor to consider is the increased synovial cell count in joint motion as compared to the overall deleterious effects of immobilization on articular cartilage. A study of acute crystal-induced joint inflammation in rabbit knees compared the effects of joint movement with immobilization.61 Over 42 days, immobilized knees had greater cartilage histologic abnormalities and loss of GAGs compared with exercised joints. Overall, joint motion enhanced the synovitis, but joint immobilization had more adverse effects on articular cartilage. Another study of a rabbit antigen-induced synovitis model had similar findings, with less articular cartilage damage at the end of the 6-week study in those rabbits that had early continuous passive range of motion as compared to those with immobilized knees.62 Therefore, it seems prudent to allow active motion of inflamed joints, with reasonable caution. Aquatic exercise may be appropriate to reduce weight-bearing stresses on articular cartilage while allowing active joint motion. Although moderate training programs appear to have no detrimental effects on articular cartilage in animals with normal joints, training program may be deleterious to articular cartilage in dogs with abnormal biomechanical stresses acting on joints. Transection of the CCL is a common model to produce OA. OA occurs as a result of joint instability during weight bearing. Joint immobilization may be somewhat protective against OA development. In a CCL transection model, the stifle joint of one group was immobilized in a cast for 12 weeks, and the other group had no immobilization for 12 weeks.22 Stifles of dogs with CCL transection without immobilization had changes typical of OA, including increased water content and PG synthesis, and decreased PG content, but the cartilage thickness was normal. Osteophytes were not seen with CCL transection and immobilization, but the cartilage was atrophic (40% thinner), water content was increased, and PG content and synthesis were diminished. These data suggest that OA develops with cruciate ligament transection as a result of mechanical instability. If the knee is immobilized after cruciate transection so that loading is reduced, OA does not develop in the short term. Medications are commonly prescribed for dogs with joint injuries. Many of these drugs may have deleterious effects on articular cartilage metabolism, especially if the joint is immobilized. For example, fluoroquinolones, such as enrofloxacin and ciprofloxacin, may be toxic to chondrocytes, resulting in cellular changes that alter cell adhesion.63 Knowledge of these effects may result in more effective rehabilitation strategies to minimize damage to cartilage. Alternatively, some drugs may provide beneficial effects to immobilized cartilage. Nonsteroidal antiinflammatory drugs (NSAIDs), such as aspirin, are commonly used in the management of OA. Aspirin may suppress PG synthesis in normal canine knee cartilage, as well as in early OA cartilage, such as that following CCL transection.64,68 Aspirin may affect GAG synthesis by inhibiting uridine diphosphate-glucose dehydrogenase, an enzyme important in the synthesis of chondroitin sulfate.64 Uptake of acetylsalicylic acid increases by 35% in osteoarthritic cartilage, suggesting that the drug permeates it more readily than in normal cartilage. The lower PG content of OA cartilage may be more important than fibrillation or surface disruption in rendering it vulnerable to aspirin.69 In addition, aspirin suppresses GAG synthesis in unloaded cartilage to a much greater extent in vitro than it does in loaded cartilage.70,71 Aspirin also markedly reduces prostaglandin E2 synthesis by the cartilage, and drug concentrations in cartilage from loaded zones are lower than those in cartilage from unloaded sites. Aspirin administration aggravates the changes that occur in cartilage during limb immobilization. In one study, a high dose of aspirin (40 mg/kg tid) was administered daily for 6 weeks while one hindlimb was immobilized in a cast.69 Whereas the cartilage of contralateral nonimmobilized limbs in dogs receiving aspirin had normal GAG content, the decreases in GAG content and net PG synthesis were significantly greater in immobilized stifles receiving aspirin as compared with immobilized stifles alone. Aspirin also apparently causes synthesis of type I rather than type II collagen by atrophic articular cartilage during immobilization.70 Although these results indicate that aspirin has an adverse effect on articular cartilage of immobilized joints, aspirin administration did not preclude reversal of these changes if the dog was allowed to walk freely for 3 weeks after cast removal.69 However, if dogs ran daily on a treadmill for 3 weeks after cast removal, the decrease in GAG content of immobilized knees persisted and actually worsened in dogs receiving aspirin. Although high doses of aspirin may have detrimental effects on cartilage, other NSAIDs apparently have little or no negative effects at doses that achieve expected levels in synovial fluid. In fact some NSAIDs may be somewhat chondroprotective, including carprofen,71,72 deracoxib,73,74 and etodolac.75,76 Treatment with corticosteroids may also have detrimental effects on normal cartilage and cartilage of immobilized limbs. Prednisone given to normal dogs with immobilized stifle joints resulted in a 58% loss of GAG content in one study.77 In nonimmobilized joints of dogs receiving prednisone, GAG content decreased 11-31%, compared with controls. The use of HA in dogs may have some benefit to articular cartilage following immobilization. In one study, stifles were immobilized for 4 weeks using transarticular external skeletal fixation.78 Treated dogs received HA. The PG content was reduced after immobilization in all dogs. Remobilization of the stifles was associated with damage to the surface and tangential layers of cartilage. However, remobilization with HA improved PG content and reduced structural damage. In another study, casts were placed on the hindlimbs of dogs for 92 days. Beginning on day 56, dogs received intraarticular injections of HA. Injections were repeated at 4-day intervals until the end of the study. Although femoral condylar articular cartilage had decreased PG content in all cast groups, the decrease was less in the HA-treated group. HA may inhibit inflammatory mediators, such as tumor necrosis factor-alpha (TNF-α) and stromelysin.79 Other studies have indicated that HA may not be beneficial for treatment of OA, however.80 Joint capsules are also affected by immobilization and remobilization. The functional and structural consequences of immobilization and remobilization of the glenohumeral joint after 12 weeks of immobilization were studied in 10 beagle dogs.81 One forelimb was immobilized in a cast. The cast was removed and remobilization was allowed for 4, 8, and 12 weeks. After 12 weeks of immobilization, the passive ROM was markedly impaired, intraarticular pressure was increased during movement, and the filling volume of the joint cavity was reduced. There was synovial lining hyperplasia and vascular proliferation in the wall of the joint capsule, but there was no increase of fibrous collagen in the capsular wall. Both the functional and structural changes were unaltered after 4 weeks of remobilization, but after 8 weeks they began to reverse, and they returned to normal levels after 12 weeks. Both functional and structural changes after 12 weeks of immobilization of a normal glenohumeral joint are reversible by remobilization. The collagen composition of the capsule seems unrelated to the degree of capsular contraction that occurs during 12 weeks of immobilization. Similar changes were observed in the carpus and elbow in dogs in which normal forelimbs were immobilized for 16 weeks.82 All dogs had a 20-30% decrease in ROM in the elbow and carpal joints, which returned to normal within 6 weeks after remobilization. In addition, all dogs were significantly lame immediately following immobilization, but returned to soundness within 6 weeks after remobilization began. Limbs casted for 32 weeks had severe joint stiffness and muscle atrophy, and recovery of normal limb use was delayed by several weeks.83 Deficits were also present after only 12 weeks of immobilization, but full function resumed much sooner. It is helpful to know which tissues contribute to joint contractures following prolonged immobilization. One study evaluated contractures in a rat model by immobilizing one knee in flexion.84 After 8 weeks, the animals were allowed to resume unrestricted activity for 4 weeks. Interestingly, 88% of the contractures remained in the immobilized group after dividing skin and muscle, suggesting an important contribution of the caudal knee capsule in limiting knee mobility. In fact, another model in rats confirmed the role of the joint capsule in contracture during knee joint immobilization.85 Joint contracture rapidly progressed after joint immobilization until 8 weeks and advanced more slowly after 8 weeks, suggesting that joint contracture develops early in immobilization and progresses over time. After releasing the caudal capsule, the immobilized group regained knee extension. The contribution of collagen content as a specific cause of joint capsule contracture is somewhat controversial. Type I collagen composes approximately 85% of the collagen content of the joint capsule, and provides mechanical strength, contributes to tissue elasticity, and is produced in sites undergoing fibrosis.86 Collagen type III may be present in tissues requiring high levels of mechanical compliance. It is a constituent of normal synovial tissue, and is also present in inflamed and proliferative rheumatoid synovium. Some studies have shown an increase in joint capsule collagen content, especially with concurrent joint injury,87–89 whereas others have shown no change or even a decrease in collagen content with joint immobilization. In a rat knee immobilization model, mRNA of type I collagen decreased rapidly after immobilization, but the immunoreactivity of the capsule was not changed in the immobilized or the control groups at any time points.90 In another study, the collagen of control and immobilized periarticular connective tissue was type I collagen, whereas type III collagen, which occurs in response to inflammation, was not present.91 The periarticular connective tissue contracture process is apparently different from the intraarticular process in which fibrofatty connective tissue and type III collagen proliferation are prominent. A later study confirmed that types I and III collagen were not increased in the joint capsule following immobilization of rat knees, suggesting that another process, such as synovial or capsular adhesions, was responsible for joint contracture.89 In addition to potential changes in collagen content, GAG and water content decrease in the joint capsule and synovium with immobilization.91 This may reduce the plasticity and lubrication efficiency of these tissues. Changes of the joint capsule undergoing contracture may also be related to growth factors. Joint capsule may produce transforming growth factor–beta1 (TGF-β1) and connective tissue growth factor (CTGF) after immobilization, and these growth factors may play a role in causing and maintaining capsular changes. Collagen synthesis and myoblast induction are related to TGF-β, and CTGF is regulated by TGF-β.92,93 CTGF also induces type I collagen formation. In a flexion knee immobilization model in rats, TGF-β1, CTGF, and fibrotic change were greater in the caudal capsule and may have resulted in limited motion in extension.92 Another study of joint injury followed by immobilization indicated similar increases in mRNA and protein levels for collagen types I and III, matrix metalloproteinases 1 and 13, and TGF-β1 were significantly greater in immobilized capsules compared with controls, whereas tissue inhibitor of matrix metalloproteinases were significantly less in experimental capsules.93 It may be possible to prevent joint contractures by somehow blocking these growth factors. In another study, although both TGF-β1 and CTGF were increased in the joint capsule after immobilization, collagen type I was not increased.93 The specific cause of joint capsule contracture may be associated with the proliferation of myofibroblasts in the joint capsule following immobilization. In one study rabbit knee joints were rigidly immobilized in maximum flexion.93 After 8 weeks groups were allowed periods of remobilization for 0, 8, 16, or 32 weeks. The average flexion contracture of knees was greater in the remobilization groups as compared with nonimmobilized controls, especially during early mobilization. Myofibroblast numbers in the posterior joint capsules were elevated four- to fivefold in the knees with contractures when compared with the contralateral knees, suggesting that these cells may be involved with joint contracture. A follow-up study indicated that these changes occur relatively soon after immobilization, and the changes persist for some time.93 Differentiated myofibroblasts are characterized by the expression of alpha–smooth muscle actin (α-SMA), a protein associated with the contractile properties of this cell.94 Mast cells and neuropeptide nerve fiber numbers may also be involved in joint capsule contracture because elevated numbers of these cells have been implicated in other fibrotic and contracture conditions. In a posttraumatic contracture model in rabbits, the knee joint was immobilized.95 Rabbits were sacrificed 4 or 40 weeks after surgery (including 32 weeks of remobilization). Myofibroblasts, mast cells, and nerve fibers were four to five times greater in the contracture capsules when compared with the control capsules at all time points, suggesting myofibroblasts, mast cells, or neuropeptide influences may underlie some of the pathologic changes in the joint capsule following trauma. In addition, the neuropeptides substance P and calcitonin gene–related peptide can cause mast cell degranulation, and this may contribute to neurogenic inflammation, pain, and swelling.96 The form of joint mobilization may be important in restoring joint motion after a period of rehabilitation. The effect of low torque–long duration stretching to increase the ROM and to restore the mechanical properties of contracted joints was studied in rat knees immobilized for 40 days.97 After remobilization, the contracted knees were placed in one of four treatment groups treated with repeated stretches of diverse torques and duration: stretching with low torque (0.02 Nm) and long duration (40 minutes), high torque (0.045 Nm) and short duration (20 minutes), high torque and long duration, and low torque and short duration. Animals received three sessions a week for 4 weeks, for a total of 12 treatment sessions. Low-torque and long-duration repeated stretching resulted in greater restoration of ROM with more normal mechanical properties compared with high-torque and short-duration stretching. Heat may be useful to improve motion in joints that have contracture after immobilization. Infrared and ultrasonic heat therapies were compared in a rat knee flexion contracture model.98 Six treatment sessions of stretching with infrared or ultrasound over a 2-week period were more effective than stretching without heat, but there was no significant differences between the two heat treatment groups. Regular therapeutic exercise is also important to recover joint motion after immobilization. Joint ROM was reduced by 46% in a 2-week ankle joint immobilization model in rats.99 Immobilization was followed by free cage activity and free remobilization or treadmill running once a week, three times a week, or six times a week. Ankle joint motion was improved in all three treadmill exercise groups in comparison with the free remobilization group, with running three and six times a week being more beneficial for recovery of joint contracture compared with no running or once-a-week running (Box 7-5).
Responses of Musculoskeletal Tissues to Disuse and Remobilization
Cartilage
Normal Articular Cartilage
Figure 7-2 Proteoglycan monomer consisting of a core protein, glycosaminoglycans, hyaluronan backbone, and link protein.
Cartilage Changes Caused by Disuse and Immobilization
The Effects of Remobilization on Cartilage
Meniscal Cartilage Changes
Adaptations of Cartilage to Increased Mechanical Stress
Effects of Training on Cartilage
Mild to Moderate Training
Severe Training
Lifelong Training
Effects of Exercise on Cartilage during Acute Inflammation
Abnormal Biomechanical Stresses
Effects of Medications on Immobilized Cartilage
Joint Capsule
Changes in Joint Capsule with Immobilization
Remobilization of Joint Capsule Immobilization
Responses of Musculoskeletal Tissues to Disuse and Remobilization
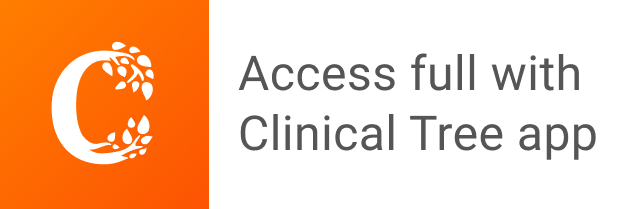